ABSTRACT
Pachymic acid, as one of bioactive ingredients in Poria Cocos, has been evidenced preclinically with pharmacological benefits including anti-bladder cancer action. Additionally, pachymic acid has been initiatorily identified in Chinese prescriptions applied for coronavirus disease 2019 (COVID-19) treatment. However, the molecular mechanisms and pharmacological targets associated with mitochondrial functions in pachymic acid against bladder cancer and COVID-19 have not been explored. In this study, network pharmacology and molecular docking detections were employed to identify common, candidate, and core targets that were related to pachymic acid treating bladder cancer COVID-19. Enrichment determinations using core target genes were used to reveal the anti-bladder cancer and COVID-19 molecular mechanisms exerted by pachymic acid. Firstly, all 1150 differentially expressed genes among bladder cancer and COVID-19 were ascertained and a risk score with key genes characterized by different risk groups was established. Furthermore, current study highlighted pachymic acid as a potential compound for anti-bladder cancer and COVID-19. Network pharmacology approaches ascertained ten key targets for pachymic acid against bladder cancer and COVID-19. Molecular docking findings showed that pachymic acid presented effectively binding abilities to key target proteins of chemokine (CC-motif) ligand 2 (CCL2), thrombospondin-1 (THBS1), matrix metallopeptidase 1 (MMP1) in severe acute respiratory syndrome coronavirus 2 ;(SARS-CoV-2). Our study indicates that pachymic acid may be used for treatment of bladder cancer and COVID-19 through increasing tumor immunity and inhibiting SARS-CoV-2 replication based on present bioinformatics findings.
Introduction
COVID-19 is a life-threatening infectious disease characterized with high infection and rapid transmission, causing global pandemic.[Citation1] Statistical data suggest that COVID-19 has caused the death toll up to 5 million infected people in the world, characterized with a lethality rate of around 2%.[Citation2] Due to continuously emerging virus variation, there is still no specific medicine to absolutely cure COVID-19. It is preliminarily found in clinical observations that patients with malignancy are more susceptible to infect with SARS-CoV-2 than other cancer-free cases owing to immunosuppression and immunodeficiency induced by chemotherapy or surgical excision.[Citation3] More obviously, cancer patients with COVID-19 may have a poorer prognosis or less survival rate.[Citation4] Clinically, bladder cancer cases might result in elevated risk of infecting SARS-CoV2 than those controls without cancers, characterized with worse prognosis and high mortality.[Citation5] Etiologically, it is observed that bladder cancer patients show a series of methylation alteration and immune abnormality,[Citation6] and these outcomes may reduce the therapeutic efficacy against COVID-19. In China, it is found that bladder cancer accounts for one of the most malignant cancers.[Citation7] The 5-year survival rate of bladder cancer patients in China is similar to that of European and American countries, approximately reaching 69%~77%.[Citation8] In view of bladder cancer patients potentially predisposing to COVID-19, some of bioactive ingredients, especially natural components, should be explored and identified for potential treatment against bladder cancer and COVID-19. Pachymic acid refers to a Poria cocos Wolf-isolated triterpenoid that is commonly used for hypoglycemic, sedative, and hypnotic usages.[Citation9] Preclinical evidences demonstrate that anti-cancer health benefits of pachymic acid are found correlation with underlying mechanisms, such as osteosarcoma involving regulation of phosphatase and tensin homolog (PTEN) and caspases-dependent apoptosis,[Citation10] lung cancer involving regulation of reactive oxygen species (ROS)-dependent c-Jun N-terminal kinase (JNK) and endoplasmic reticulum (ER) stress pathways.[Citation11] Our previous study has showed that pachymic acid exerts potential action against cystitis glandularis as highlighted in bioinformatics and experimental findings.[Citation12] An in vitro study displays that pachymic acid inhibits cell proliferation in human bladder cancers via inducing ROS generation, modulating Bcl-2 and inhibitor of apoptosis (IAP) family member expressions.[Citation13] In addition, a network pharmacology merged molecular docking analysis shows that pachymic acid may be used for possible treatment against COVID-19 through suppression of Mpro, a key protease in SARS-CoV-2.[Citation14] It is reported that network pharmacology strategy is currently used for identification of therapeutic targets and molecular mechanisms in candidate bioactive agents against human cancers and COVID-19.[Citation15,Citation16] Given current reference reports and experimental data, anti-bladder cancer and COVID-19 targets and mechanisms in pachymic acid activity should be investigated and revealed, respectively. Our research was designed to identify pharmacologically bioactive pachymic acid to suppress bladder cancer and COVID-19 and then further reveal related molecular mechanisms. In this research, we firstly applied bioinformatics analysis to perform the health risk evaluation for identifying differential expression genes (DEGs) among bladder cancer and COVID-19 and to identify the prognosis of cancer patients. And then pachymic acid was explored as potential bioactive agent to inhibit bladder cancer and COVID-19. By using network pharmacology approach, we ascertained the molecular mechanisms of pachymic acid against bladder cancer and COVID-19, and core therapeutic target proteins were validated via molecular docking simulation before future clinical application of pachymic acid treating bladder cancer and COVID-19.
Materials and methods
Identification of DEGs in bladder cancer and COVID-19
First of all, transcriptomic analysis data for bladder cancer were downloaded from The Cancer Genome Atlas (TCGA) database (https://portal.gdc.cancer.gov/repository). The R-package “edge” tool was used to perform the difference assay of the transcriptomic profiling data in bladder cancer, and the filtering settings used | log (2fold change) |>2 and false discovery rate (FDR) <0.05. And then, COVID-19-correlative genes were collected and detected from Online Mendelian Inheritance in Man (OMIM) database (http://omim.org), GeneCards database (relevance score > 1) (www.genecards.org), and the National Coalition Building Institute (NCBI) gene database (https://www.ncbi.nlm.nih.gov/). All intersection genes between bladder cancer and COVID-19 were obtained accordingly, as previously reported.[Citation17]
Analysis of the associativity between prognosis and clinical phenotype
Construction of a multivariate Cox model for the intersection of COVID-19 target extraction was used to identify molecular clinical signatures associated with prognosis.[Citation18] Survival analysis was performed by using the Kaplan–Meier method and log-rank test to determine the correlation between the molecular clinical signature and cancer patient survival.[Citation19]
Clinical correlation and stratified analysis of prognosis
Chi-square test was used to investigate the associativity between prognostic and clinical characteristics, comprising age, gender, tumor node metastasis stage.[Citation20] To assess whether the risk score was independent for available clinicopathological features, we performed univariate and multivariate analyses via the associated datasets. In addition, we also determined whether prognostic factors still have predictive potential in different subgroups based on age, gender, clinical stage, and risk factors, so we used the receiver operating characteristic (ROC) curve to identify the accuracy of current models.[Citation21]
Targets-based network establishment of pachymic acid and bladder cancer/COVID-19
Furthermore, candidate targets of pachymic acid were screened and collected from available online databases, including SuperPred (http://prediction.charite.de), Pharmmapper (http://lilab.ecust.edu.cn/pharmmapper/), Integrative Pharmacology-based Research Platform of Traditional Chinese Medicine (TCMIP) (http://www.tcmip.cn/TCMIP/). The identified targets of pachymic acid were used to compare with DEGs in bladder cancer and COVID-19 for selecting intersection genes. All intersection genes were used for plotting the protein–protein interaction (PPI) network through String online tool (https://cn.string-db.org/). The topological parameters were determined by using network analyzer setting in the Cytoscape software (https://cytoscape.org/).[Citation22]
Enrichment analysis and visualization of network data
In brief, detection and visualization of Gene Ontology (GO) and Kyoto Encyclopedia of Genes and Genomes (KEGG) from all intersection targets in pachymic acid and bladder cancer/COVID-19 were implemented through applying the R-packages of “ClusterProfiler.,” “org.Hs.eg.Db,” “ggplot2” settings. The output data of the enrichment data were presented as bar and bubble charts based on adjusted p-value Cut off = 0.05, q-value Cut off = 0.05. Meanwhile, the Cytoscape software was used to build up the network diagram of pachymic acid and targets-GO function-KEGG pathway-bladder cancer/COVID-19.[Citation23]
Molecular docking analysis
To evaluate whether pachymic acid would dock with COVID-19-associated proteins, molecular docking technology was used to characterize the spatial-binding sites of pachymic acid. By using the PubChem database (https://pubchem.ncbi.nlm.nih.gov),[Citation24] we obtained the molecular structure of pachymic acid.[Citation24] The target protein structures of CCL2, THBS1, and MMP1 were downloaded from the Protein Data Bank (PDB) database (http://www.rcsb.org/pdb/). The ChemBio3D Draw software was applied to raise hydrogen atoms and diminish energy to these protein structures. And the ligand and receptor were processed with AutoDock Tools (https://github.com/ccsb-scripps/AutoDock-Vina), aiming to add hydrogen atoms, eliminate water molecules and produce relational files. A root mean square deviation (RMSD) cluster analysis was used to set RMSD-tolerance as 4 Å for valid docking standardization. The PyMOL visualization software was employed to visualize and exhibit the receptor residues and the hydrogen bonds between protein ligands and receptors, as previously described.[Citation25]
Results
Screening of bladder cancer and COVID-19 related genes
After common screening, a total of 1774 candidate genes in bladder cancer were obtained. After searching via databases, another 2528 relevant genes of COVID-19 were collected. And then we obtained 189 intersection targets between bladder cancer and COVID-19 through Venn mapping analysis ().
Findings in prognosis and clinical phenotype
These 189 candidate molecular signatures were inputted to the univariate Cox regression model to yield 42 potential molecular signatures. Being entered the multi-factor Cox regression model for forward and backward optimization, and then finally 21 alternative genes with different hazard ratios were obtained as shown in . And the nomogram showed a more intuitive display of the changes in the five-year and three-year survival rates of bladder cancer patients ().
Independent prognostic analysis results
Cox regression data showed that the risk score of bladder cancer patients had a significant prognostic effect, and we set the median of the risk score as a critical value to distinguish bladder cancer patients. As a result, 431 bladder cancer patients were assigned as high-risk and low-risk groups. Risk scores based on the 21 molecular signatures were significantly associated with overall survival (OS) in patients with bladder cancer. Kaplan–Meier curve analysis visibly exhibited that the high-risk group had a shorter OS compared with that in low-risk group (P = 1.166e-14, log-rank test). The distribution of risk scores, survival status in the dataset based on these 21 prognostic gene signatures are shown in .
Verification of model-data accuracy
Univariate Cox regression analysis showed that risk score and staging condition were independent prognostic indicators, and the HR for risk score was 1.062 (95% CI = 1.041–1.084, P < .001) (). In multivariate analysis, risk score remained an independent prognostic indicator (HR = 1.055, 95% CI = 1.032 − 1.079, P < .001) (). We used the dataset to construct an ROC curve for assessing risk prediction ability. As shown in , risk score, tumor node metastasis stage mostly contributed to the three- and five-year survival rate and sensitivity in bladder cancer patients. The predictive ability of the risk score reached 0.807. The predictive capabilities of the three-year and five-year survival models for bladder cancer patients reached 0.751 and 0.717. And then we performed dimensionality reduction analysis on these data, and the principal component analysis (PCA) graph was used to better describe the distribution of data. The PCA picture represented the distribution of risk signature genes across all patient gene expression profiles, with relative dispersion between high- and low-risk groups ().
Figure 4. Risk score and staging condition as independent prognostic indicators using monofactor analysis (a). Risk score as an independent prognostic indicator using multivariate analysis (b).
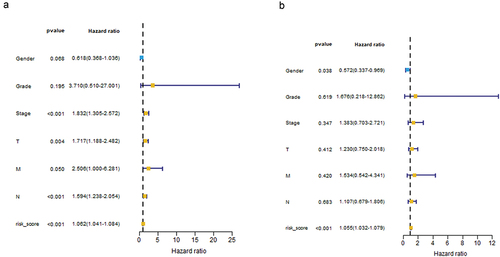
Identifying of potential targets of pachymic acid against bladder cancer and COVID-19
After being screened and corrected by the available databases, a total of 324 molecular targets of pachymic acid were obtained. The targets collected were mapped through Venn diagram, and a total of 10 intersection targets among bladder cancer/COVID-19 and pachymic acid were identified and interrelated, including F2, IDO1, KCNH2, NTRK3, CCL2, PTGS2, ACTA2, MMP1, CCNE1, and THBS1 ().
Findings in GO and KEGG enrichment analysis
The enrichment data showed that GO-based biological processes were mainly involved in positive regulation of response to external stimulus, negative regulation of fibrinolysis, regulation of fibrinolysis, positive regulation of fibroblast migration, negative regulation of astrocyte differentiation, chronic inflammatory response, regulation of metal ion transport, regulation of wound healing, positive regulation of transforming growth factor beta production, positive regulation of cation transmembrane transport (). The cell components related to target genes were main involvement with endoplasmic reticulum lumen. Other molecular functions associated with target genes were mostly involved in oxidoreductase activity, acting on single donors with incorporation of molecular oxygen, incorporation of two atoms of oxygen, oxidoreductase activity, acting on single donors with incorporation of molecular oxygen, dioxygenase activity, growth factor binding, heme binding, tetrapyrrole binding, heparin binding, serine-type endopeptidase activity, serine-type peptidase activity, serine hydrolase activity (). Additionally, KEGG analysis mostly comprised 11 molecular pathways (FDR < 0.05), including IL-17 signaling pathway, bladder cancer, malaria, coronavirus disease-COVID-19, p53 signaling pathway, microRNAs in cancer, small cell lung cancer, rheumatoid arthritis, human papillomavirus infection, TNF signaling pathway ().
Findings in molecular docking analysis
We searched CCL2, THBS1, and MMP1 main proteins through the PDB database, and we selected 6TPZ, 5FOE, and 966C spatial structures for molecular docking respectively. In the 6TPZ of CCL2, the RMSD of the proligand was 2.734 Å. The hydrogen bond between the original ligand NV2 and the 6TPZ acted on the amino acid residue of ASN-140 (2.9 Å), and the free docking energy with the protein was −7.8 Kcal/mol. Pachymic acid formed a hydrogen bond with the amino acid residue of 6TPZ (3.0 Å), and the free docking energy with the protein was −6.3 Kcal/mol (). In the 5FOE of THBS1, the RMSD of the proligand was 2.378 Å. The hydrogen bond between the original ligand GDP and the 5FOE acted on the amino acid residues in SER-1017 (3.1Å), GLY-53 (3.4 Å), PHE-54 (2.5 Å), ASN-55 (3.1 Å), LEU-56 (3.4 Å), THR-383 (3.0 Å), SER-382 (3.0Å), ARG-290 (3.1Å), ASP-366 (2.5 Å), and the free docking energy with the protein was −11.1 Kcal/mol. Pachymic acid formed the hydrogen bond with the amino acid residues in ARG-290 (3.2Å), SER-382 (3.4Å), and the free docking energy with the protein was −3.9 Kcal/mol (). In the 966C of MMP1, the RMSD of the proligand was 2.604 Å. The hydrogen bond between the original ligand RS2 and the 966C protein acted on the amino acid residues in HIS-228 (3.2Å), HIS-218 (3.2Å), HIS-222 (3.2Å), ALA-182 (2.2 Å), LEU-181 (2.0 Å), ASN-180 (2.6 Å), and the free docking energy with the protein was −9.9 Kcal/mol. Pachymic acid formed a hydrogen bond with the amino acid residue ASN-180 (2.3 Å), and the free docking energy with the protein was −5.3 Kcal/mol ().
Discussion
COVID-19 has ranked the greatest public health challenge in the world. And the COVID-19 treatments include antiviral agents, neutralizing antibody therapy, and hormonotherapy.[Citation26] However, specific medicine for treating COVID-19 have not yet been on the market. Malignant tumor is one of the clinical challenges in global burden of life-threatening diseases.[Citation27] Bladder cancer is one of the leading causes of tumor-induced death in the world.[Citation28] Although individual heterogeneity and drug tolerance occur, immunotherapy is still one of the clinical treatment strategies with potent efficacy for bladder cancer patients.[Citation29] In medical diagnosis, bladder cancer patients, especially advanced stage, are commonly found with alterations of immune cell components when extended chemotherapy.[Citation30] Immune dysregulation may lead to potential tumorigenicity, including bladder cancer.[Citation31] As reported previously, these cancer patients may further suffer the high risk for mortal COVID-19 outcomes based on interaction of tumor therapy action affecting the immune function and chronic comorbidity induced by COVID-19.[Citation32] Thus, we conclude that bladder cancer patients may be more vulnerable to infection of SARS-CoV-2 during the pandemic, resulting in further affecting clinical treatment efficacy and reducing overall survival duration. In this study, we primarily obtained 1150 DEGs in bladder cancer, and 189 intersecting genes between bladder cancer and COVID-19. We conducted a risk score with 21 risk factors that was correlated with the corresponding clinical phenotype and gene expression characteristic in bladder cancer patients. These findings of gene expression or clinical phenotype associated with patient prognosis could be used to construct the prognostic models. Additionally, the assessment data suggested that increased death rate/risk in bladder cancer patients was linked with higher risk scores. Meanwhile, the prognostic analysis using risk score indicated therapeutic efficiency for bladder cancer cases. The analytical findings of the target 21 gene expressions that was significantly associated with overall survival of bladder cancer patients presented these genes might act as potential diagnostic hallmarks for bladder cancer and COVID-19. Dimensionality reduction analysis exhibited that PCA map represented the distribution of risk-signature genes across the gene expression profiles of all bladder cancer patients, characterized with relative dispersion between the high-risk and low-risk groups. Using network pharmacology analysis indicated that all 10 core targets in pachymic acid against bladder cancer/COVID-19 were identified and characterized for functional enrichment visualization. GO-related findings exhibited that anti-bladder cancer/COVID-19 processes of pachymic acid might be involved in regulation of microenvironmental regulation and reduction of cell proliferation. KEGG-related findings revealed that anti-bladder cancer/COVID-19 mechanisms of pachymic acid might be associated with regulation of molecular immunology and inhibition of viral activity. Molecular docking analysis further identified the key targeting proteins, including CCL2, THBS1, and MMP1. CCL2, an integral chemotactic gene, binds to the tumor cell receptor CC Chemokine Receptor 4 (CCR4), and enhances the regeneration ability of cancer cells, and then binds to CCR2 in immune cells to cause an immunosuppressive microenvironment and promote tumor angiogenesis.[Citation33] CCL2 may lead to angiogenesis through heat shock protein 47 activation in bladder cancers in vivo and in vitro.[Citation34] Thus, CCL2 and related receptor may be the pharmacological targets in malignant cancers as CCL2 can trigger host anti-tumor action.[Citation35] Additionally, CCL2 is a promising prognostic factor for a severe course of COVID-19.[Citation36] THBS1, a cell adhesion molecule, plays key roles in the tumor microenvironment to inhibit angiogenesis, mediate antitumor immunity, and modulate tumor cell migration.[Citation37] It is found that THBS1 may be used for prognostic valuation in developing bladder cancer on the basis of pathological significance.[Citation38] THBS1 may be a potential effector in cell progression via regulating miR-19a-3p action, characterized as a therapeutic target for treatment with bladder cancer.[Citation39] Additionally, inflammatory-regulated THBS1 gene in COVID-19 patient can be acting on potential biomarker to manage COVID-19.[Citation40] MMP1, an activator of the G protein-coupled protease activated receptor-1, refers to an underlying target functioning in oncogenesis and metastasis in common cancers.[Citation41] It is clinically found that urinary MMP1 levels may be used as potential therapeutical target in patients with bladder cancer.[Citation42] Excessive MMP1 expression is found in COVID-19 patient and then is related to the COVID-19 severity.[Citation43] As reported previously, anti-cancer activities of pachymic acid are evident through regulating endoplasmic reticulum stress and mitochondrial dysfunction.[Citation44] Additionally, anti-cancer effects in vivo and in vitro of pachymic acid are found in preclinical studies.[Citation45,Citation46] Thus, we consider that pachymic acid may enhance the therapeutic effectiveness of antivirals and immunotherapy for anti-bladder cancer/COVID-19 in future clinical practice. Instead, our present report still occurs in certain limitations. To start with, the risk assessment score was determined and exhibited through retrospective report assay. And current prospective findings warrant to further be verified clinically. And then, the pharmacological biotargets based on network pharmacology and molecular docking analyses still need to be certified experimentally prior to ultimate clinical application.
Conclusion
Taken together, the therapeutic prognosis and clinical phenotype of bladder cancer patients were characterized via using bioinformatics analysis and medical model. In addition, pachymic acid is preliminarily identified as a promising bioactive ingredient for anti-bladder cancer/COVID-19 action, and the pharmacological mechanisms of anti-bladder cancer/COVID-19 mainly enrich in anti-cancer and anti-inflammatory pathways as well as immunomodulation. Moreover, pachymic acid may lower viral replication by effectively binding core target proteins in COVID-19.
Author contributions
Bo Ge contributed to the conception, design of the manuscript. Baotong Zhou, Bojian Liang, Min Chen, Yongbo Tang, Haiwei Hu, Qiuhong Chen, Jintao Wen, Zhouliang Wen, Ende Cui, Wei Zhong, Jimin Su contributed to the acquisition, analysis, and interpretation of data in this manuscript. Bo Ge drafted and revised this manuscript. All authors agree to be accountable for all aspects of work ensuring integrity and accuracy.
Disclosure statement
No potential conflict of interest was reported by the author(s).
Additional information
Funding
References
- Sharma, A.; Tiwari, S.; Deb, M. K.; Marty, J. L. Severe Acute Respiratory Syndrome Coronavirus-2 (SARS-CoV-2): A Global Pandemic and Treatment Strategies. Int. J. Antimicrob. Agents. 2020, 56(2), 106054. DOI: 10.1016/j.ijantimicag.2020.106054.
- Huang, Y. Z.; Kuan, C. C. Vaccination to Reduce Severe COVID-19 and Mortality in COVID-19 Patients: A Systematic Review and Meta-Analysis. Eu.R Rev. Med. Pharmacol. Sci. 2022, 26, 1770–1776.
- Liang, W.; Guan, W.; Chen, R.; Wang, W.; Li, J.; Xu, K.; Li, C.; Ai, Q.; Lu, W.; Liang, H., et al. Cancer Patients in SARS-CoV-2 Infection: A Nationwide Analysis in China. Lancet Oncol. 2020, 21(3), 335–337. DOI: 10.1016/S1470-2045(20)30096-6.
- Shuman, A. G.; Pentz, R. D. Cancer Research Ethics and COVID-19. Oncologist. 2020, 25(6), 458–459. DOI: 10.1634/theoncologist.2020-0221.
- Singh, M. K.; Jain, M.; Shyam, H.; Shankar, P.; Singh, V. Associated Pathogenesis of Bladder Cancer and SARS-CoV-2 Infection: A Treatment Strategy. VirusDis. 2021, 32(4), 613–615. DOI: 10.1007/s13337-021-00742-y.
- Chen, J. Q.; Salas, L. A.; Wiencke, J. K.; Koestler, D. C.; Molinaro, A. M.; Andrew, A. S.; Seigne, J. D.; Karagas, M. R.; Kelsey, K. T.; Christensen, B. C. Immune Profiles and DNA Methylation Alterations Related with Non-Muscle-Invasive Bladder Cancer Outcomes. Clin. Epigenetics. 2022, 14(1), 14. DOI: 10.1186/s13148-022-01234-6.
- Li, H. Z.; Zheng, R. S.; Du, L. B.; Zhang, S. W.; Zhu, C.; Wei, W. W.; He, J. Bladder Cancer Incidence, Mortality and Temporal Trends in China. Zhonghua Zhong Liu Za Zhi. 2021, 43(3), 293–298. DOI: 10.3760/cma.j.cn112152-20200421-00362.
- National Cancer Center; Bladder Cancer Expert Committee of National Cancer Quality Control Center. [Quality Control Index for Standardized Diagnosis and Treatment of Bladder Cancer in China (2022 Edition)]. Zhonghua Zhong Liu Za Zhi. 2022, 44(10), 1003–1010. doi:10.3760/cma.j.cn112152-20220803-00531.
- Li, L.; Zuo, Z. T.; Wang, Y. Z. The Traditional Usages, Chemical Components and Pharmacological Activities of Wolfiporia Cocos: A Review. Am. J. Chin. Med. 2022, 50(2), 389–440. DOI: 10.1142/S0192415X22500161.
- Wen, H.; Wu, Z.; Hu, H.; Wu, Y.; Yang, G.; Lu, J.; Yang, G.; Guo, G.; Dong, Q. The Anti-Tumor Effect of Pachymic Acid on Osteosarcoma Cells by Inducing PTEN and Caspase 3/7-Dependent Apoptosis. J. Nat. Med. 2018, 72(1), 57–63. DOI: 10.1007/s11418-017-1117-2.
- Ma, J.; Liu, J.; Lu, C.; Cai, D. Pachymic Acid Induces Apoptosis via Activating ROS-Dependent JNK and ER Stress Pathways in Lung Cancer Cells. Cancer Cell Int. 2015, 15(1), 78. DOI: 10.1186/s12935-015-0230-0.
- Feng, Z.; Shi, H.; Liang, B.; Ge, T.; Cai, M.; Liu, F.; Huang, K.; Wen, J.; Chen, Q.; Ge, B. Bioinformatics and Experimental Findings Reveal the Therapeutic Actions and Targets of Pachymic Acid Against Cystitis Glandularis. BioFactors. 2021, 47(4), 665–673. DOI: 10.1002/biof.1734.
- Jeong, J. W.; Lee, W. S.; Go, S. I.; Nagappan, A.; Baek, J. Y.; Lee, J. D.; Lee, S. J.; Park, C.; Kim, G. Y.; Kim, H. J., et al. Pachymic Acid Induces Apoptosis of EJ Bladder Cancer Cells by DR5 Up-Regulation, ROS Generation, Modulation of Bcl-2 and IAP Family Members. Phytother. Res. 2015, 29(10), 1516–1524. DOI: 10.1002/ptr.5402.
- Wu, Z.; Chen, X.; Ni, W.; Zhou, D.; Chai, S.; Ye, W.; Zhang, Z.; Guo, Y.; Ren, L.; Zeng, Y. The Inhibition of Mpro, the Primary Protease of COVID-19, by Poria Cocos and Its Active Compounds: A Network Pharmacology and Molecular Docking Study. R.S.C. Adv. 2021, 11(20), 11821–11843. DOI: 10.1039/D0RA07035A.
- Liu, J.; Chen, Y.; Nie, L.; Liang, X.; Huang, W.; Li, R. In Silico Analysis and Preclinical Findings Uncover Potential Targets of Anti-Cervical Carcinoma and COVID-19 in Laminarin, a Promising Nutraceutical. Front Pharmacol. 2022, 13, 955482. DOI: 10.3389/fphar.2022.955482.
- Yang, L.; Xiong, H.; Li, X.; Li, Y.; Zhou, H.; Lin, X.; Chan, T. F.; Li, R.; Lai, K. P.; Chen, X. Network Pharmacology and Comparative Transcriptome Reveals Biotargets and Mechanisms of Curcumol Treating Lung Adenocarcinoma Patients with COVID-19. Front Nutr. 2022, 9, 870370. DOI: 10.3389/fnut.2022.870370.
- Yang, L.; Yang, N.; Huang, H.; Yu, J.; Sui, X.; Tao, L.; Gao, Y.; Liu, Z. Bioinformatics Analysis to Identify Intersection Genes, Associated Pathways and Therapeutic Drugs Between COVID-19 and Oral Candidiasis. Comb. Chem. High Throughput Screen. 2023, 26(8), 1533–1546. DOI: 10.2174/1386207325666221007111239.
- Zheng, W.; Wu, H.; Wang, T.; Zhan, S.; Liu, X. Quercetin for COVID-19 and DENGUE Co-Infection: A Potential Therapeutic Strategy of Targeting Critical Host Signal Pathways Triggered by SARS-CoV-2 and DENV. Brief Bioinform. 2021, 22(6), bbab199. DOI: 10.1093/bib/bbab199.
- Tang, B.; Zhu, J.; Zhao, Z.; Lu, C.; Liu, S.; Fang, S.; Zheng, L.; Zhang, N.; Chen, M.; Xu, M., et al. Diagnosis and Prognosis Models for Hepatocellular Carcinoma Patient’s Management Based on Tumor Mutation Burden. J. Adv. Res. 2021, 33, 153–165. DOI: 10.1016/j.jare.2021.01.018.
- McHugh, M. L. The Chi-Square Test of Independence. Biochem. Med. (Zagreb). 2013, 23, 143–149. DOI: 10.11613/BM.2013.018.
- Cheng, X.; Wang, X.; Nie, K.; Cheng, L.; Zhang, Z.; Hu, Y.; Peng, W. Systematic Pan-Cancer Analysis Identifies TREM2 as an Immunological and Prognostic Biomarker. Front. Immunol. 2021, 12, 646523. DOI: 10.3389/fimmu.2021.646523.
- Shannon, P.; Markiel, A.; Ozier, O.; Baliga, N. S.; Wang, J. T.; Ramage, D.; Amin, N.; Schwikowski, B.; Ideker, T. Cytoscape: A Software Environment for Integrated Models of Biomolecular Interaction Networks. Genome Res. 2003, 13(11), 2498–2504. DOI: 10.1101/gr.1239303.
- Qin, Q.; Qin, L.; Xie, R.; Peng, S.; Guo, C.; Yang, B. Insight into Biological Targets and Molecular Mechanisms in the Treatment of Arsenic-Related Dermatitis with Vitamin a via Integrated in silico Approach. Front Nutr. 2022, 9, 847320. DOI: 10.3389/fnut.2022.847320.
- Kim, S.; Chen, J.; Cheng, T.; Gindulyte, A.; He, J.; He, S.; Li, Q.; Shoemaker, B. A.; Thiessen, P. A.; Yu, B., et al. PubChem in 2021: New Data Content and Improved Web Interfaces. Nucleic Acids Res. 2021, 49(D1), 1388–1395. DOI: 10.1093/nar/gkaa971.
- Seeliger, D.; de Groot, B. L. Ligand Docking and Binding Site Analysis with PyMol and Autodock/Vina. J. Comput. Aided. Mol. Des. 2010, 24(5), 417–422. DOI: 10.1007/s10822-010-9352-6.
- Yuan, Y.; Jiao, B.; Qu, L.; Yang, D.; Liu, R. The Development of COVID-19 Treatment. Front. Immunol. 2023, 14, 1125246. DOI: 10.3389/fimmu.2023.1125246.
- de Martel, C.; Georges, D.; Bray, F.; Ferlay, J.; Clifford, G. M. Global Burden of Cancer Attributable to Infections in 2018: A Worldwide Incidence Analysis. Lancet Glob. Health. 2020, 8(2), 180–190. DOI: 10.1016/S2214-109X(19)30488-7.
- Tran, L.; Xiao, J. F.; Agarwal, N.; Duex, J. E.; Theodorescu, D. Advances in Bladder Cancer Biology and Therapy. Nat. Rev. Cancer. 2021, 21(2), 104–121. DOI: 10.1038/s41568-020-00313-1.
- Li, T.; Liu, T.; Zhao, Z.; Pan, Y.; Xu, X.; Zhang, Y.; Zhan, S.; Zhou, S.; Zhu, W.; Guo, H., et al. Antifungal Immunity Mediated by C-Type Lectin Receptors May Be a Novel Target in Immunotherapy for Urothelial Bladder Cancer. Front. Immunol. 2022, 13, 911325. DOI: 10.3389/fimmu.2022.911325.
- Song, D.; Powles, T.; Shi, L.; Zhang, L.; Ingersoll, M. A.; Lu, Y. J. Bladder Cancer, a Unique Model to Understand Cancer Immunity and Develop Immunotherapy Approaches. J. Pathol. 2019, 249(2), 151–165. DOI: 10.1002/path.5306.
- Martin, A.; Woolbright, B. L.; Umar, S.; Ingersoll, M. A.; Taylor, J. A. Bladder Cancer, Inflammageing and Microbiomes. Nat. Rev. Urol. 2022, 19(8), 495–509. 3rd. DOI: 10.1038/s41585-022-00611-3.
- Seneviratne, S. L.; Wijerathne, W.; Yasawardene, P.; Somawardana, B. COVID-19 in Cancer Patients. Trans. R. Soc. Trop. Med. Hyg. 2022, 116(9), 767–797. DOI: 10.1093/trstmh/trac015.
- Su, W.; Han, H. H.; Wang, Y.; Zhang, B.; Zhou, B.; Cheng, Y.; Rumandla, A.; Gurrapu, S.; Chakraborty, G.; Su, J., et al. The Polycomb Repressor Complex 1 Drives Double-Negative Prostate Cancer Metastasis by Coordinating Stemness and Immune Suppression. Cancer Cell. 2019, 36(2), 139–155. DOI: 10.1016/j.ccell.2019.06.009.
- Ma, W.; Ou, T.; Cui, X.; Wu, K.; Li, H.; Li, Y.; Peng, G.; Xia, W.; Wu, S. HSP47 Contributes to Angiogenesis by Induction of CCL2 in Bladder Cancer. Cell. Signal. 2021, 85, 110044. DOI: 10.1016/j.cellsig.2021.110044.
- Conti, I.; Rollins, B. J. CCL2 (Monocyte Chemoattractant Protein-1) and Cancer. Semin. Cancer Biol. 2004, 14(3), 149–154. DOI: 10.1016/j.semcancer.2003.10.009.
- Pius-Sadowska, E.; Niedźwiedź, A.; Kulig, P.; Baumert, B.; Sobuś, A.; Rogińska, D.; Łuczkowska, K.; Ulańczyk, Z.; Wnęk, S.; Karolak, I., et al. CXCL8, CCL2, and CMV Seropositivity as New Prognostic Factors for a Severe COVID-19 Course. Int. J. Mol. Sci. 2022, 23(19), 11338. DOI: 10.3390/ijms231911338.
- Isenberg, J. S.; Roberts, D. D. THBS1 (Thrombospondin-1). Atlas Genet Cytogenet. Oncol. Haematol. 2020, 24(8), 291–299. DOI: 10.4267/2042/70774.
- Nakamura, Y.; Miyata, Y.; Takehara, K.; Asai, A.; Mitsunari, K.; Araki, K.; Matsuo, T.; Ohba, K.; Sakai, H. The Pathological Significance and Prognostic Roles of Thrombospondin-1, and -2, and 4N1K-Peptide in Bladder Cancer. Anticancer. Res. 2019, 39(5), 2317–2324. DOI: 10.21873/anticanres.13348.
- Xu, G.; Li, J.; Yu, L. MiR-19a-3p Promotes Tumor-Relevant Behaviors in Bladder Urothelial Carcinoma via Targeting THBS1. Comput. Math Methods Med. 2021, 2021, 2710231. DOI: 10.1155/2021/2710231.
- Hamldar, S.; Kiani, S. J.; Khoshmirsafa, M.; Nahand, J. S.; Mirzaei, H.; Khatami, A.; Kahyesh-Esfandiary, R.; Khanaliha, K.; Tavakoli, A.; Babakhaniyan, K., et al. Expression Profiling of Inflammation-Related Genes Including IFI-16, NOTCH2, CXCL8, THBS1 in COVID-19 Patients. Biologicals. 2022, 80, 27–34. DOI: 10.1016/j.biologicals.2022.09.001.
- Foley, C. J.; Luo, C.; O’Callaghan, K.; Hinds, P. W.; Covic, L.; Kuliopulos, A. Matrix Metalloprotease-1a Promotes Tumorigenesis and Metastasis. J. Biol. Chem. 2012, 287(29), 24330–24338. DOI: 10.1074/jbc.M112.356303.
- Du, X.; Lin, B. C.; Wang, Q. R.; Li, H.; Ingalla, E.; Tien, J.; Rooney, I.; Ashkenazi, A.; Penuel, E.; Qing, J. MMP-1 and Pro-MMP-10 as Potential Urinary Pharmacodynamic Biomarkers of FGFR3-Targeted Therapy in Patients with Bladder Cancer. Clin. Cancer Res. 2014, 20(24), 6324–6335. DOI: 10.1158/1078-0432.CCR-13-3336.
- Syed, F.; Li, W.; Relich, R. F.; Russell, P. M.; Zhang, S.; Zimmerman, M. K.; Yu, Q. Excessive Matrix Metalloproteinase-1 and Hyperactivation of Endothelial Cells Occurred in COVID-19 Patients and Were Associated with the Severity of COVID-19. J. Infect. Dis. 2021, 224(1), 60–69. DOI: 10.1093/infdis/jiab167.
- Yang, T.; Tian, S.; Wang, Y.; Ji, J.; Zhao, J. Antitumor Activity of Pachymic Acid in Cervical Cancer Through Inducing Endoplasmic Reticulum Stress, Mitochondrial Dysfunction, and Activating the AMPK Pathway. Environ. Toxico. 2022, 37(9), 2121–2132. DOI: 10.1002/tox.23555.
- Gui, Y.; Sun, L.; Liu, R.; Luo, J. Pachymic Acid Inhibits Inflammation and Cell Apoptosis in Lipopolysaccharide (LPS)-Induced Rat Model with Pneumonia by Regulating NF-Κb and MAPK Pathways. Allergol. Immunopathol. 2021, 49(5), 87–93. DOI: 10.15586/aei.v49i5.468.
- Lee, Y. H.; Lee, N. H.; Bhattarai, G.; Kim, G. E.; Lee, I. K.; Yun, B. S.; Hwang, P. H.; Yi, H. K. Anti-Inflammatory Effect of Pachymic Acid Promotes Odontoblastic Differentiation via HO-1 in Dental Pulp Cells. Oral Dis. 2013, 19(2), 193–199. DOI: 10.1111/j.1601-0825.2012.01970.x.