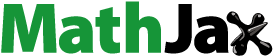
ABSTRACT
The aim of the present study was to determine the binding mechanisms between zein and folic acid in an ethanol – water solution. Fluorescence spectroscopy demonstrated that folic acid quenched the fluorescence intensity of zein by both dynamic and static fluorescence quenching mechanisms, with static quenching playing a prominent role. The binding constants (Ka) of the zein-folic acid complex at 292, 302, and 312 K were evaluated to be 5.97 × 105, 3.00 × 105 and 1.54 × 105 L/mol, respectively. The process of folic acid binding zein was driven by ∆H0 = –51.24 kJ/mol, ∆S0 = –64.90 J/mol•K. Van der Waals forces and hydrogen bonding primarily governed the formation of the zein-folic acid complex. Confirmation of the complex formation was obtained via UV-visible absorption spectroscopy, Fourier transform infrared spectroscopy, and circular dichroism spectroscopy, which also revealed alterations in the secondary structure of zein upon binding to folic acid. Measurements of particle size and zeta potential demonstrated an enhancement in the stability of the complex system. Scanning electron microscopy and transmission electron microscopy showed the complex to possess a spherical morphology, with significant changes in the zein’s morphology following its binding to folic acid. Additionally, zein increased the photostability of folic acid against UV light radiation. Molecular docking and molecular dynamics simulations were used to investigate the binding mechanisms involved further, and the results effectively elucidated the observed experimental phenomena. This research establishes a significant theoretical foundation for the utilization of zein as a system for delivering and safeguarding folic acid.
Introduction
Among bioactive compounds, the vitamin B family has garnered considerable interest owing to its vital biological functions.[Citation1] Folic acid (FA), also recognized as vitamin B9, stands as an indispensable micronutrient and bioactive compound. It plays a pivotal role in various human biochemical processes, including nucleic acid and protein synthesis, the generation of red and white blood cells, and the synthesis of modulators for brain function.[Citation2,Citation3]
Indeed, FA holds a critical role in human health, and its deficiency can result in various health issues, including abnormalities in fetal nervous system development, megaloblastic anemia, Alzheimer’s disease, cardiovascular disease, and various types of cancer.[Citation4,Citation5] Given that FA cannot be synthesized within mammalian cells, FA-containing vitamins serve as crucial exogenous sources for its delivery in both food and medicine.[Citation6] However, FA faces several biological limitations. It is highly susceptible to UV light, oxygen, and heat, which can lead to the generation of inactive products.[Citation7,Citation8] Moreover, FA is prone to degradation in acidic conditions.[Citation9] Therefore, there is an urgent need for strategies that safeguard FA from the acidic environment of the stomach and other unfavorable environmental factors to enhance its bioavailability.
Protein modification or encapsulation stands as a common strategy employed to enhance FA stability and its bioavailability.[Citation9–12] Clear evidence indicates protective interactions between FA and various proteins, resulting in the formation of noncovalent complexes. For instance, FA formed hydrophobic interactions with β-casein, effectively inhibiting the photodecomposition of FA.[Citation13] FA also engaged in hydrophilic and hydrophobic interactions with human and bovine serum albumins, leading to partial protein unfolding. These serum albumins can serve as carrier proteins for FA, facilitating its delivery to target molecules.[Citation10] Additionally, a robust affinity was observed between FA and ovalbumin, with the interaction primarily stabilized by hydrophobic and hydrogen bonds.[Citation14] Buffalo whey proteins strongly bind to FA through noncovalent interactions and form ground-state complexes, improving the photostability of FA against UV radiation.[Citation15] The binding of FA to lactoferrin occurs spontaneously at different temperatures, and all the complexes exhibit good thermodynamic stability.[Citation16] These studies of the molecular interactions between proteins and FA play key roles in the assessment of new carrier agent systems, such as FA protection and delivery systems.
Zein, a secure plant protein renowned for its limited water solubility, elevated alcohol solubility, proficient film-forming capabilities, biocompatibility, and biodegradability, has gained recognition.[Citation17–19] Zein is helical and comprises nine helical segments of approximately 20 amino acids with glutamine-rich “turns” or “loops.”[Citation20] Within this three-dimensional framework, zein has the potential to accommodate various guests.[Citation21] Furthermore, zein possesses a distinct hydrophilic and hydrophobic amino acid region, which contributes to its unique amphiphilic and self-assembly properties. Zein’s unique structure and qualities make it a suitable encapsulation material. Researchers have successfully encapsulated a variety of bioactive small molecules, such as epigallocatechin-3-gallate,[Citation22] ferulic acid,[Citation23] anthocyanins,[Citation24] curcumin,[Citation25] and resveratrol,[Citation26] into zein nanoparticles by the anti-solvent precipitation method. These zein-based nanotransport systems have exhibited increased photostability, enhanced pH stability, and increased resistance to gastrointestinal conditions.[Citation27] By using a variety of spectroscopic techniques and theoretical calculations, it was found that these bioactive small molecules have a moderate or higher binding affinity with zein in an ethanol – water solution.[Citation24,Citation28–31] For example, zein exhibited robust binding to curcumin via hydrophobic interactions, leading to an approximate 50% reduction in α-helix content and an augmentation in β-sheets within the zein structure.[Citation30] The interaction between zein and anthocyanins was driven by van der Waals forces, hydrogen bonding, and hydrophobic interactions, with anthocyanins capable of binding to the inner active pockets of zein.[Citation24] These interactions have a significant impact on the stability and functional properties of the complexes formed.[Citation32,Citation33] Moreover, zein displays mucoadhesive properties and a relatively high resistance to the effects of digestive enzymes,[Citation34] which can effectively improve the bioavailability of complexed or encapsulated bioactive small molecules.
Based on the above analysis, we believe that zein could be an optional protein for protecting FA. Because zein is soluble in alcohol solutions but insoluble in water, protein-bioactive small molecule interactions are often investigated in an ethanol-water environment. FA, despite being a hydrophilic vitamin, exhibits poor water solubility in neutral aqueous solutions due to its planar shape and intramolecular π-π interactions.[Citation14] This low water solubility causes obstacles in formulations and delivery processes.[Citation35] Notably, solubility enhancement is observable in both alkaline and acidic environments. Additionally, FA demonstrates mild solubility in organic solvents like methanol, ethanol, and butanol.[Citation36] In an ethanol-water solution, the solubility of FA can be significantly increased by appropriately adjusting the solution’s pH, enabling the incorporation of FA into zein via an anti-solvent method. This technique has been employed for FA delivery using zein as the carrier, and the relative oral bioavailability of encapsulated FA was significantly improved.[Citation34] However, the precise molecular-level binding mechanism between zein and FA remains unexplored. Understanding the mechanism of binding of bioactive small molecules to carrier proteins is beneficial for predicting the consequences of these interactions in the human body and elucidating the properties of this complex with important biological significance in vitro.[Citation37,Citation38]
The objective of this work was to investigate the binding mechanism between zein and FA in an ethanol-water solution. FA’s molecular structure comprises three components: a glutamic acid moiety, a p-aminobenzoic acid moiety, and a pterin moiety. FA includes hydrophilic hydroxyl, amino, and carboxyl groups, along with hydrophobic long carbon chains and aromatic groups. Consequently, the interaction between zein and FA is intricate, necessitating a variety of methods for its examination. In this study, the binding mechanism between zein and FA in an ethanol-water solution was explored through multi-spectroscopy, dynamic light scattering (DLS), electron microscopy, molecular docking, and molecular dynamics (MD) simulation. This research expands the application potential of the plant protein zein as a transportation and protection system for vitamin substances.
Materials and methods
Materials and sample preparation
Folic acid (FA) (catalog number F103635) with a purity of ≥ 97% was sourced from Aladdin Corp. in Shanghai, China. Zein (catalog number Z3625) was procured from Sigma‒Aldrich Corp. in St. Louis, MO, USA. All other reagents used in the experiments met analytical grade standards. Throughout the entirety of the experiments, ultra-pure water was employed.
To initiate the experiments, a precise quantity of zein was weighed and dissolved in an 80% (v:v) ethanol solution to attain a concentration of 10.0 µM (with the molecular weight of zein set at 25 KD). FA was dissolved at varying concentrations in PBS (20 mM Na2HPO4/NaH2PO4, pH 8.0). It should be noted that all solutions were freshly prepared for use.
Absorbance measurements
The ultraviolet – visible (UV – vis) spectra of zein and different concentrations of FA were determined by a UV spectrophotometer (UV-2550, Shimadzu, Japan) at wavelengths ranging from 235‒450 nm. The zein solution and FA solution were mixed in specific proportions. The concentration of zein remained constant at 2.5 µM, while the concentration of FA was varied within the range of 0–25 µM. A quartz cuvette with a 1 cm path length was employed for the testing. To ascertain the actual absorption of zein-FA complexes, the contribution of an equal quantity of FA was subtracted from the absorption of the mixture at the corresponding concentration.[Citation39]
Fluorescence measurements
Fluorescence measurements were conducted using a fluorescence spectrophotometer (LS55, PerkinElmer, USA) at temperatures of 292 K, 302 K, and 312 K. The zein solution and FA solution were mixed in specified proportions. The concentration of zein remained fixed at 2.5 µM, while the concentration of FA was varied within the range of 0–25 µM. Samples were excited with a wavelength of 280 nm, and emission spectra were recorded in the range of 290‒420 nm. Both the emission and excitation slit widths were set at 5 nm. Raw spectra were corrected by subtracting solvent and FA backgrounds. Additionally, the observed fluorescence data were adjusted for the inner filter effect (IFE) caused by FA absorption in the excitation and emission wavelengths using Equation (1)[Citation40–42]:
where Fobs and Fcor refer to the fluorescence intensity before and after correction, respectively. Aex and Aem are the absorption values of the system at an excitation wavelength of 280 nm and an emission wavelength of 305 nm, respectively.
Fourier transform infrared (FTIR) spectroscopy
Freeze-dried samples were readied in an 80% (v:v) ethanol solution. Infrared spectra of these samples were obtained using an FTIR spectrometer (NICOLET IS5, Thermo Scientific, Germany). The sample was blended with potassium bromide in a 1:100 ratio, subsequently compressed into a transparent sheet using a mold. Pure KBr served as the baseline reference. The wavelength range covered 4000‒400 cm−1, and each spectrum was generated from 32 scans, with a resolution of 4 cm−1.
Circular dichroism (CD) measurements
The CD spectra of the zein and FA mixtures were captured in the wavelength span of 190–260 nm within a N2 environment, employing a spectropolarimeter (J-1700, JASCO, Japan). The alterations in the secondary structure of zein were assessed using a CD multivariate secondary structure estimation (SSE) program under two conditions: in the absence (5 µM) and presence of varying concentrations of FA (5 µM, 25 µM, and 50 µM).
Dynamic light scattering (DLS)
Dynamic light scattering (DLS)The average particle size, polydispersity index (PDI), and zeta potential of the complexes resulting from the addition of FA at various ratios were determined using a Malvern Zetasizer Nano ZSE instrument (Malvern, England) with the DLS application program. These measurements were conducted at a temperature of 25°C after allowing for a 120-second equilibration period.
Radiation treatment
Mixtures containing FA (10.0 µM) and various concentrations of zein (0, 2.5, 5.0, and 10.0 µM) were exposed to UV light (365 nm) using an UV lamp (8 W, ZF-7B, Gucun, China). The fluorescence intensity (FI) of the mixtures was measured at 455 nm with an excitation wavelength of 348 nm. Samples were analyzed every 10 min for up to 40 min.
Morphology observation
The morphology of freeze-dried zein and complex samples was examined using scanning electron microscopy (SEM, SU8010, Hitachi, Japan). Prior to observation, the sample surface was coated with a thin layer of sputtered gold. Samples diluted to 0.5 mg/mL were deposited on plasma-cleaned carbon film-coated copper mesh, followed by negative staining with 0.5% uranyl acetate. The morphology of freshly prepared particles was investigated via transmission electron microscopy (TEM, JEM-1400plus, JEOL, Japan).
Molecular docking
AlphaFold2 (AF2), a state-of-the-art artificial intelligence (AI) system developed by DeepMind for protein structure prediction, can predict three-dimensional (3D) structures of proteins from amino acid sequences with atomic-level accuracy.[Citation43] The amino acid sequence of zein (ID: Q946V7) from the UniProt database was selected for this study.[Citation44] The 3D structure of zein was predicted using the single monomer mode, resulting in five top-ranked structures. The highest-ranked structure underwent optimization by GalaxyRefine, and its quality was assessed using PROCHECK. Protein pretreatment, including the addition of hydrogens and minimization of hydrogens in altered species, was performed using the academic version of Mastro (Schrodinger, California, USA). The 3D structure of FA was generated using RDKit, followed by optimization with the mmff94s force field.[Citation45] The single point energy of the generated conformation was calculated using the B3LYP/6–31 G** method,[Citation46] and the conformation with the lowest energy was selected for subsequent docking analysis. Charges were added to the lowest energy conformation using the AM1-BCC method through the UCSF Chimera program.[Citation47] Molecular docking studies were conducted using AutodockVina 1.2 (Scripps Research, California, USA). The binding pocket of zein was identified using PyVOL (Schrodinger, California, USA), with an energy grid box measuring 35 Å along each edge centered on the predicted region. The search space at the binding pocket was defined as a cube with dimensions of 22.5 × 22.5 × 22.5 Å in the X, Y, and Z axes.
MD simulation
MD simulations were conducted using the Gromacs 5.1.5 software package. The ligand-receptor complex was solvated in 80% ethanol at pH 7.0. Periodic boundary conditions were established with a minimum distance of 1.0 Å between the protein and the box edge. Initially, receptor topology files were converted using the pdb2gmx program and the ff99SB force field,[Citation48] while ligand topology files were processed using Amber Tools (UCSF, California, USA) with the general AMBER force field (GAFF).[Citation49] The simulation system then underwent a two-phase equilibrium for 100 ps, maintaining a constant temperature of 300 K and pressure of 1 atm. This equilibrium consisted of constant particle number, pressure, and temperature (NPT) and constant particle number, volume, and temperature (NVT) phases. Post-equilibrium, a 100 ns MD simulation was executed with a 2 fs time step. The LINCS (Linear Constraint Solver) algorithm was applied to constrain covalent bond lengths, and long-range electrostatic interactions were computed using the PME (Particle Mesh Ewald) method.[Citation50] Water molecules were handled by the SETTLE algorithm.[Citation51] Trajectory data was collected every 100 ps for subsequent analysis. The essential parameters of Gromacs, such as root mean square deviation (RMSD), root mean square fluctuation (RMSF), and radius of gyration (Rg) were analyzed using these trajectories. Additionally, the binding free energy was computed employing the gmx_MMPBSA tool via the Molecular Mechanics/Poisson-Boltzmann Surface Area (MM/PBSA) method.
Statistical analysis
All the experiments were performed at least in triplicate. The results are expressed as the mean ±SD and were statistically analyzed by one-way ANOVA (IBM SPSS Statistics 22, USA). A value of p < .05 was considered to indicate statistical significance. For the data analysis and graph creation, Origin 2018 (Origin Lab, USA) was utilized.
Results and discussion
UV – vis spectroscopy
UV – vis spectroscopy, a simple and reliable technique, is used to investigate protein structure changes and interactions.[Citation52] Zein possesses nine tyrosine residues and no tryptophan residues. As depicted in , zein without FA exhibited notable absorption at 278 nm, attributable to the π-π* electronic transitions of tyrosine residues in zein. The spectra of FA displayed strong absorption bands around 286 and 366 nm, corresponding to the π-π* and n-π* electronic transitions of the pterin ring and p-amino benzoyl acid moieties in FA, respectively.[Citation53] presents the spectra derived from subtracting the spectrum of free FA solutions from the absorbance of the zein-FA mixture at corresponding concentrations. Absorbance intensity escalated progressively with the addition of FA to the protein solution. This increase in absorbance is likely due to the contribution of π-π* transitions of the pterin moiety in FA.[Citation53] Additionally, a slight redshift was observed, with the maximum absorption wavelength shifting from 278 nm to 280 nm. These findings suggest that FA can bind to zein, forming a zein-FA complex and that the microenvironment around the aromatic residues undergoes minor modifications with increasing FA concentration, indicative of a conformational transformation in the protein molecules.[Citation54]
Figure 1. (a) UV‒vis spectra of 2.5 µM zein in the presence of FA at different concentrations (0–25 µM). (b) Fluorescence emission spectrum of 2.5 µM zein in the presence of FA at 312 K. Stern–Volmer plots (c) and Lineweaver–Burk plots (d) of zein quenched by increasing concentrations of FA at 292 , 302 , and 312 K.
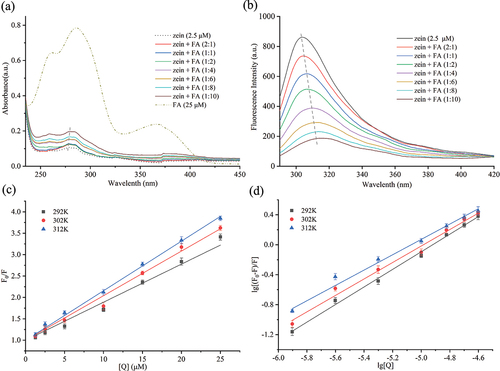
Fluorescence spectroscopy
Fluorescence quenching is a widely utilized method for studying the interactions between proteins and active small molecules.[Citation55] Monitoring changes in emission peaks enables the determination of protein conformational alterations. Proteins exhibit intrinsic fluorescence, predominantly emanating from tryptophan, tyrosine, and phenylalanine residues.[Citation56] Among these, tryptophan and tyrosine are the most important chromophoric groups in proteins, given phenylalanine’s relatively low quantum yield. Zein, containing no tryptophan and nine tyrosine residues, exhibits fluorescence due to the excitation of tyrosine residues. Typically, tyrosine is excited at a wavelength of 280 nm, and its maximum emission intensity is influenced by the surrounding environment. An ethanol-water solution, commonly used for zein, shows zein’s maximum emission wavelength to be around 305 nm in an approximately 80% v/v solution.[Citation57] A more polar local environment results in a redshift of the maximum emission wavelength, while a less polar environment causes a blueshift.[Citation31] The concentration of zein was fixed at 2.5 μM. At this concentration, a clear, reproducible spectrum could be obtained over the entire wavelength range which did not suffer from the detection limit of the instrument. Once a fixed concentration of zein with different concentrations of FA was mixed, the emission spectra of zein were conspicuously decreased, which was a concentration-dependent response to the conformational changes of proteins and is referred to as quenching.[Citation38] However, the concentration of FA should not be too large, and its self-sudden extinction should be avoided. illustrates the fluorescence spectra of zein at varying FA concentrations. The figure shows that the peak intensities of zein decrease with increasing FA concentrations. The quenching efficiency of 25 µM FA (1:10 ratio) on zein fluorescence was found to be 77.95%, suggesting that FA can almost completely quench zein’s fluorescence. The occurrence of quenching manifested the capability of FA in interacting with zein and quenching its fluorescence intensity.[Citation38] The λmax of zein exhibited a redshift from 305 nm to 316 nm upon the addition of FA, indicating that FA interacts with zein and alters the microenvironmental polarity around the tyrosine residues in zein.[Citation58] Fluorescence quenching includes dynamic quenching and static quenching. The mechanism of fluorescence quenching can be distinguished by the Stern-Volmer equation [Citation59]:
where F0 and F represent the fluorescence intensities before and after the addition of FA, respectively; [Q] denotes the concentration of the quencher, FA. τ0 is the average lifetime of the fluorescent substance in the absence of any quencher. KSV and kq are the Stern-Volmer quenching constant and the dynamic quenching constant, respectively. The primary source of intrinsic fluorescence in zein is attributed to Tyr, with a τ0 value of approximately 3.6 × 10−9 s. Stern-Volmer plots () depicting the fluorescence quenching of zein by FA at temperatures of 292, 302, and 312 K, were used to derive values for KSV, kq, and the linear regression coefficient R2 () using EquationEquation (2)(2)
(2) . Based on this equation, KSV is simply calculated as the slope of a plot of F0/F versus [Q]. It describes the rate at which a fluorophore interacts with a quencher through a dynamic collision process. As per the data presented in , there was a slight rise in the values of KSV with the corresponding increase in temperature, suggesting that the quenching process was initiated by dynamic quenching.[Citation60] On the other hand, the calculated kq values exceed 1013, significantly surpassing 2.0 × 1010 L/mol•s (the maximum limit for diffusion-controlled collision quenching rate constant), indicating that the static quenching may be the prominent mechanism in the zein-FA system.[Citation32,Citation61,Citation62] Therefore, it could be deduced that the quenching process of zein by FA was initiated by a combined mechanism of quenching, which involves both dynamic and static quenching processes.[Citation35,Citation63] This type of quenching originating from the formation of complexes between proteins and ligands has a more complex relationship between KSV and temperature. This is because, depending on the forces involved in the interaction, an increase in temperature would increase or decrease complex formation between the protein and the ligand.[Citation31] For instance, an increase in complex formation would be predicted if hydrophobic interactions predominated because of the strengthening of hydrophobic forces at higher temperatures. Conversely, a decrease in complex formation would be anticipated if hydrogen bonding predominated because hydrogen bridges weaken at higher temperatures.
Table 1. Stern – Volmer quenching constants of the zein-FA complex at different temperatures.
To analyze the static binding parameters of zein in the presence of FA, such as the binding constant (Ka) and the number of binding stoichiometries (n), the Lineweaver – Burk equation was used[Citation64]:
where F0, F, and [Q] are the same as in EquationEquation (2)(2)
(2) . Ka is the binding constant, and n is the binding stoichiometry. By constructing plots of lg [(F0–F)/F] against lg [Q] (), values for the binding constant (Ka), the number of binding sites (n), and the linear regression coefficient (R2) at various temperatures (292, 302, and 312 K) were ascertained. These values were determined from the intercept and slope of the plots, as depicted in . Experiments were conducted at 292–312 K since proteins do not undergo any gross structural changes in this temperature range.[Citation65] Excessive temperatures will induce zein to form disulfide-crosslinked protein oligomers and polymers, leading to irreversible thermal denaturation and consequent severe changes in the secondary structure of the proteins.[Citation66] The binding constant (Ka) describes the affinity between a protein and a specific ligand. It represents the tendency of the protein to form a complex with the ligand. A higher Ka value indicates a stronger binding affinity between the biomolecule and its ligand. As per the data presented in , the binding constant Ka values were greater than 105 across all the evaluated temperatures, indicating a relatively high affinity between zein and FA.[Citation29] The observed decrease in the Ka with increasing temperature suggests that higher temperatures diminish the binding affinity within zein-FA complexes. Conversely, lower temperatures appear to enhance their interaction. This trend aligns with findings reported for the formation of human serum albumin-FA[Citation67] and zein-resveratrol complexes.[Citation31] The binding stoichiometry, indicated by an n value near 1, reveals that FA binds positively and cooperatively to zein.[Citation68] This implies that one FA molecule interacts with each zein molecule, consistent across different temperatures.
Table 2. Thermodynamic and binding parameters of the zein-FA complex at different temperatures.
To determine the main binding force in the formation complex of zein-FA, we need thermodynamic parameters, including the enthalpy change (ΔH0), entropy change (ΔS0), and free energy change (ΔG0), which can be assessed by the van’t Hoff equation[Citation69,Citation70]:
where R is the molar gas constant (8.314 J/mol•K), and Ka is the binding constant. The analysis of binding constants at different temperatures facilitated the determination of the changes in enthalpy (ΔH0), entropy (ΔS0), and Gibbs free energy (ΔG0), with their values presented in . The negative values of both ΔG0 and ΔH0 indicate that the interaction between zein and FA is exothermic and that their binding process is spontaneous.[Citation71] This correlation between the signs of thermodynamic parameters and the nature of potential interactions was previously outlined by Ross and Subramania.[Citation72] ∆H0 <0 and ∆S0 >0 indicate the presence of electrostatic forces; ΔH0 >0 and ΔS0 >0 indicate hydrophobic forces; ΔH0 <0 and ΔS0 <0 denote van der Waals forces and hydrogen bonding, respectively. In the current scenario, where both ΔH0 and ΔS0 exhibit negative values, it can be inferred that van der Waals forces and hydrogen bonding constitute the predominant intermolecular forces governing the interactions between FA and zein. This outcome additionally validated that the static quenching process held a dominant position. Also, our group has studied the interaction mechanism between zein and FA in an alkali aqueous solution. At 302 and 322 K, the binding constants Ka were 5.52 × 104 and 2.12 × 104 L/mol, respectively, and hydrogen bonding and van der Waals forces were the predominant forces in the formation of zein-FA complexes.[Citation73] It can be seen that zein and FA were combined in both solvents by hydrogen bonding and van der Waals forces. In comparison, there was a higher affinity between zein and FA in an ethanol – water solution. Therefore, it can be inferred that FA and zein may combine in vivo with moderate or high affinity through hydrogen bonding and van der Waals forces.
FTIR spectroscopy
To better understand the potential intermolecular interactions between zein and FA, FTIR analysis was carried out. illustrates the infrared spectra of FA, zein, and their complexes. In the FA spectrum, bands in the range of 3600–3100 cm−1 arise from stretching vibrations of O – H in glutamic acid and N – H in the pterin ring. The peak at 1694.6 cm−1 corresponds to C=O stretching vibration, while the band at 1606.6 cm−1 is associated with N – H bending. Bands from 1485.0 to 1412.7 cm−1 are characteristic of phenyl and pterin rings’ absorption.[Citation74] The zein spectrum features major bands at 3301.3 cm−1, indicative of O – H, N – H, and hydrogen bond stretching (amide A); 1658.6 cm−1, linked to C =O bond stretching (amide I); and 1537.2 cm−1, associated with C – N bond stretching and N – H bond bending (amide II).[Citation75] In the zein-FA complexes (molar ratios 1:1, 1:5, and 1:10), notable differences compared to pure zein are observed. The amide A band in zein-FA complexes shows a significant red shift, and the peaks are located at 3310.6 and 3339.8 cm−1 with the ratios of 1:1 and 1:5, respectively. At this band, the peak of the zein-FA1:10 breaks into two peaks at 3376.2 and 3129.7 cm−1, which also occurred after the interaction between preheated whey protein isolates and anthocyanins.[Citation76] These changes suggest the strong hydrogen bond formation between zein and FA. The hydrogen bonds likely form between zein’s glutamine amide groups and FA’s carboxylic (glutamic acid) OH groups, and the pteridine NH2 and NH groups.[Citation77] With increasing FA concentration, the amide I and II bands exhibit changes in position and intensity, indicative of interactions primarily with the C=O of p-amino-benzoic acid of FA.[Citation77] This results in the rearrangement of the secondary structure of zein, as detailed in the CD spectroscopy analysis. The amide I band shows a blueshift and the amide II band a redshift, revealing an electrostatic interaction between zein and FA.[Citation78] Besides, the absorption peak of amide II was related to the β-sheet regions at the interface of proteins as turns, hence the weakening of this band is manifested as a certain degree of aggregation of particles.[Citation79] It is most obvious in the spectrum of zein-FA1:10. New peaks distinct from those in the FA monomer appear in the zein-FA (1:1, 1:5, and 1:10) complex spectra located in the 1300–750 cm−1 range, corresponding to C – O stretching vibrations.[Citation80] These peaks intensify and also break up into two bands with increasing FA concentration, highlighting a direct relationship to FA. However, the typical peak of FA near 1694.6, 1606.6, 1485.0 and 1412.7 cm−1 do not show up in the FTIR spectra of the three ratios of the complexes. Therefore, these new peaks are most likely owing to protein denaturation and partial aggregation produced by the progressive addition of FA. This particular level of aggregation is what gives the FA the best protection. These changes in the spectra of the zein indicate that interaction between FA and the protein functional groups has occurred, in particular the O – H, N – H, and amide bands. This indicates that bonding has aroused between FA and zein.
Figure 2. (a) FTIR spectra of the FA, zein, and zein-FA samples. (b) CD spectra of zein (5.0 µM) and zein with different concentrations of FA. (c) The particle size,PDI, and zeta potential of zein (2.5 µM) and zein with different concentrations of FA. (d) Natural log of (FI/FI0) versus UV irradiation time for mixtures containing FA (10.0 µM) and various concentrations of zein (0, 2.5, 5.0, and 10.0 µM). Different letters indicate a statistically significant difference (p < .05).
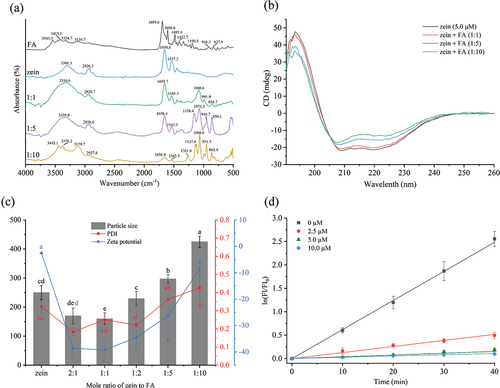
CD spectroscopy
CD is an effective and simple technique for monitoring the secondary structure modifications of proteins when they are attached to ligands.[Citation81] As illustrated in , the CD spectra of zein exhibit a positive peak at 193 nm, a zero crossing around 202 nm, and two negative peaks at 209 and 223 nm. These characteristics are indicative of a predominantly α-helical secondary structure.[Citation82] The negative peak at 208 nm is ascribed to π–π* transitions, while the negative peak at 223 nm is likely due to n–π* transitions of the α-helix and random coil structures.[Citation44] FA binding to zein results in a decrease in the 208 nm and 223 nm bands, suggesting a reduction in the content of the α-helix. In addition, the peaks do not show conspicuous shifts, suggesting considerable changes in the secondary structure of zein.[Citation83] The secondary structure content of zein, both with and without FA, was analyzed using CD multivariate secondary structure estimation (SSE) software, with data presented in . This analysis reveals that the α-helix content significantly decreases from 67.0% to 40.8%, accompanied by a substantial increase in β-sheets from 0.3% to 39.0%. These findings indicate that FA’s presence alters zein’s secondary structure, disrupting the original α-helices and leading to a more open, disordered structure.[Citation84] Extended β-sheets are commonly found in aggregated proteins.[Citation85] The results further confirm that the aggregation occurred at a high FA concentration and the aggregates were partly composed of β-sheets. These changes observed in the CD spectra suggest that upon complexation with high concentrations of FA, zein may have formed a melt-like stable intermediate structure, i.e., the molten globule (MG) state, which is an equilibrium intermediate state between the native (N) state and the fully unfolded (U) state.[Citation86,Citation87] It might influence the physiological functioning of the protein.[Citation88] This phenomenon is similar with FTIR spectrum results.
Table 3. Secondary structure analysis of zein and zein-FA complexes based on CD spectra.
Particle size and surface charge
The exploration of protein aggregation and changes in particle size, PDI, and zeta potential following the binding of zein to FA was conducted. illustrates that the particle size of zein at a concentration of 2.5 µM was 250 ± 24 nm. With a fixed zein concentration, the particle size of the zein-FA complexes initially decreased and subsequently increased with the addition of increasing amounts of FA. As the zein-to-FA molar ratio shifted from 2:1 to 1:1, the particle size of the complex diminished from 170 ± 27 nm to 160 ± 20 nm. This decrease suggests an interaction between zein and FA, leading to smaller, more stable particles. Conversely, when the zein-to-FA molar ratio increased from 1:1 to 1:10, the particle size of the complex expanded from 160 ± 20 nm to 425 ± 19 nm. This increase in size may be attributed to the high concentration of FA inducing protein aggregation, resulting in enlarged particle sizes. The polydispersity indices of all the complexes were less than 0.43, which suggested that the particle size distributions were relatively narrow.
The trend in the absolute zeta potential of the complex was observed to be inverse to that of the particle size. Zeta potential effectively characterizes the mutual attraction among particles. A lower absolute value of the zeta potential indicates diminished repulsion between particles, facilitating easier attraction and aggregation among them. Conversely, a higher absolute value suggests increased mutual repulsion, reducing the likelihood of particle collision and aggregation. reveals that zein particles exhibit a low negative charge of −2.7 mV. At neutral pH, zein possesses a very low surface charge, resulting in insufficient electrostatic repulsion to counteract strong hydrophobic attraction among nanoparticles, leading to aggregation.[Citation89] Upon the addition of FA, a marked increase in the absolute value of the zeta potential is observed. This enhancement in the absolute potential value of the composite system suggests an interaction between zein and FA, enhancing system stability. When the zein-to-FA molar ratio is increased from 2:1 to 1:1, the zeta potential of the zein-FA complex shifts from −38.5 mV to −41.4 mV. This increase in the absolute potential value signifies greater system stability, potentially due to the interaction between zein and FA at low concentrations. This interaction may alter the conformation of the protein’s secondary structure, leading to protein unfolding and increased electrostatic and steric hindrance between particles. Consequently, more anionic groups are exposed on the particle surface. As the zein-to-FA molar ratio further increases from 1:1 to 1:10, the zeta potential of the complex changes from −38.5 mV to −23.1 mV, indicating a decrease in system stability. This change could be due to enhanced interactions between zein and FA, promoting protein aggregation. The resulting increase in particle size reduces the specific surface area and decreases the absolute value of the potential.
Influence of zein on the photosensitivity of FA
FA is light sensitive and is easily degraded by UV light. When FA is irradiated by UV light, the C9-N10 bond is broken and free pterin is released. The high quantum yield of free pterin leads to an increase in its fluorescence intensity at 455 nm.[Citation13] Therefore, we can monitor the degradation of FA using fluorescence spectroscopy. The natural log of the ratio of FI (fluorescence intensity at time t) to FI0 (fluorescence intensity at time zero) of the UV-irradiated zein-FA mixture was calculated (). When FA was irradiated alone, a significant increase in intensity was observed, indicating partial release of pterins and great damage to FA. The rate of photodegradation of FA in the zein-containing mixture was significantly reduced, and this protective effect was superior with increasing zein concentration. This could be a result of FA’s interaction with zein’s binding cavity, which prevents FA from hydrolyzing in the presence of light.[Citation15]
SEM and TEM
SEM and TEM were utilized to investigate the microstructures of the samples. displays the microscopic morphology of zein and zein-FA complexes with molar ratios of 1:1 and 1:5. All samples were spherical in shape. The SEM images reveal non-uniform particle sizes, attributed to the preparation method involving freeze-dried samples and solvent evaporation. The surface of zein without FA appeared smooth (). At a 1:1 zein-FA ratio, the particle size of the complex remained relatively unchanged, but the surface became notably rougher (). The 1:5 zein-FA complex exhibited more size variability (). Higher FA concentrations led to aggregated complex proteins forming larger particles with rougher surfaces, aligning with the results from particle size and zeta potential analyses. TEM images provided a more accurate representation of particle size. As depicted in , the surface of zein without FA was indeed smooth, and the particle size was about 250 nm. The particles in the 1:1 zein-FA complex appeared significantly shrunken with rough surfaces, and the particle size was approximately 200 nm (). With increased FA, the complex particle size gradually enlarged. The particles of the 1:5 zein-FA complex were less homogeneous in particle size, in line with the PDI results; also, the average particle size was essentially comparable to that of zein without FA () These results are generally consistent with the trends observed in the particle size measurements.
Molecular docking study
The 3D structure of zein was determined, and the quality of the model was assessed using PROCHECK (details provided in Supplementary Materials). Following the determination of the protein structure, molecular docking was conducted using AutoDock Vina. illustrate that the FA molecule is embedded within the hydrophobic cavity of zein. Docking analysis, as shown in identified multiple interactions between zein and FA. These interactions predominantly include van der Waals forces, hydrogen bonding, and three types of π-stacking interactions: π-lone, π-π, and π-alkyl. FA engages in van der Waals interactions with zein at various residues, specifically Gln202, Val204, Leu211, Val208, Leu64, Phe210, Gln192, Leu196, Ile58, Ser61, Ser57, and Gln30. Additionally, hydrogen bonds are formed with Pro201, Asn207, Gln214, Ser77, and Gln75. The hydrophobic interaction primarily occurs between the protein’s aromatic and aliphatic amino acids and the benzene and pterin rings of FA. A T-shaped π-π stacking interaction is observed between the benzene ring of FA and the side chain benzene ring of Phe199. Furthermore, the FA molecule’s pteridine ring forms π-alkyl and π-lone pair interactions with Leu78 and Gln195, respectively. The strongest binding affinity between zein and FA, as predicted by the docking analysis, is −10 kcal/mol (−41.8 kJ/mol). This value closely aligns with the binding energy determined by the binding constant.[Citation90]
Figure 4. Interaction between zein and FA. (a, b) 3D schematic diagram of the binding of zein to FA (red represents the hydrophobic interface; white indicates the hydrophilic interface). (c, d) Binding patterns of zein with FA (hydrogen bond is shown as a green dashed line). (e) 2D schematic diagram of the binding of zein to FA.

Molecular dynamics simulation study
The root mean square deviation (RMSD) measures the average displacement of atoms in a specific frame relative to the reference frame. Moreover, monitoring changes in protein structure over time is important for determining whether a system is balanced.[Citation91] As depicted in , the RMSD fluctuation of the zein-FA complex protein exhibited a relatively significant variation. FA likely interacts with zein and changes its structure. Since there was no ligand in the original structure of zein, when FA penetrated within, the conformation of zein may have adjusted, after which the material expanded first. During the last 40 ns of the simulation time, the RMSD value of zein-FA was found to be lower than that of zein. A low RMSD signifies a more stable protein structure with fewer variations in molecular conformation.[Citation92] This observation suggests that the structure of zein-FA tended to be stable, possibly even more stable than that of zein.
Figure 5. (a) The RMSD values of zein and the zein-FA complex. (b) The RMSF values of zein and the zein-FA complex. (c) The Rg values of zein and the zein-FA complex.
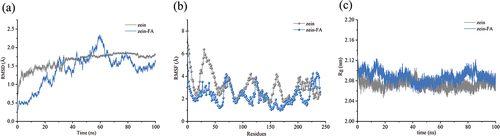
The root mean square fluctuation (RMSF) can be used to measure local changes in the protein chain and is an important parameter for characterizing the flexibility of a residue.[Citation93] illustrates that the RMSF fluctuation of zein-FA exhibited notably less variation than that of zein, particularly within the regions spanning 0–65, 103–124, 149–187, and 196–224. The RMSF values exhibited substantial alterations, accompanied by a marked reduction in flexibility, thereby underscoring the enhanced structural stability resulting from the interaction between zein and FA. Hydrogen bonds formed between residues Gln214 and Asn207 with FA molecules, while van der Waals forces were established between Val204, Leu211, Val208, Ser57, and Gln30 with FA molecules. Their RMSF values experienced a significant decline following FA binding, providing further evidence of the prominent role played by hydrogen bonding and van der Waals forces in the interaction between zein and FA. Additionally, of the nine tyrosine residues present in the zein molecule, the RMSF values of Tyr31, Tyr42, Tyr48, Tyr70, Tyr130, Tyr180, and Tyr200 were markedly reduced. Tyr31, Tyr42, and Tyr48 had decreases greater than 3 Å. These findings also provided a plausible explanation for the characterization of zein fluorescence quenching in the presence of FA.
The radius of gyration (Rg) is an important parameter for describing the compactness of a protein structure.[Citation94] An elevation in the Rg value is linked to the expansion of a protein molecule. As depicted in , the zein-FA complex exhibited elevated Rg values consistently throughout the simulation, indicating that the binding of FA induced a reduction in the protein’s compactness, resulting in a shift toward a looser structural state.[Citation90] This phenomenon can be elucidated by the outward expansion of proteins when FA was introduced into the internal region of zein. Consequently, it is hypothesized that the binding of FA facilitated a conformational rearrangement of zein, a hypothesis supported by CD spectral data. A comparable outcome was observed in the interaction between natamycin and zein.[Citation95]
The binding free energy serves as a crucial parameter for evaluating the interaction between zein and FA. Typically, it can be computed using the Molecular Mechanics/Poisson-Boltzmann Surface Area (MM/PBSA) method[Citation96] (refer to the Supplementary Material for detailed information). In , the negative values for van der Waals energy (ΔEvdW, −147.13 ± 14.15 kJ/mol) and electrostatic energy (ΔEele, −36.83 ± 3.29 kJ/mol) indicate that these two types of interactive forces play a promotive role in the binding between zein and FA. Conversely, the change in solvation-free energy (∆Gsolv, 33.85 ± 6.03 kJ/mol) is a positive value, indicating that desolvation has an adverse effect on binding and is not conducive to system integration. Therefore, van der Waals forces and electrostatic forces were the main contributors to the affinity between zein and FA. According to the findings from thermodynamic and FTIR analyses, hydrogen bonding emerges as a significant driving force, underscoring its pivotal role in electrostatic energy. The binding free energy serves as a crucial metric for evaluating protein-small molecule interactions. The binding free energy (ΔGbind) for the complex system is negative (−146.19 ± 13.87 kJ/mol), affirming the spontaneous nature of the interaction between zein and FA. To assess the energy contribution of individual residues in complex formation, we conducted energy decomposition analysis. The calculated per-residue energy contributions may be either positive or negative. Positive contributions are unfavorable, while negative ones favor complex stability. Typically, residues with an energy contribution exceeding −8 kJ/mol (−2 kcal/mol) are regarded as “hotspots,” significantly contributing to binding and complex stabilization.[Citation97] illustrates the residue energy decomposition for the zein-FA complex. The hotspot residues involved in the interaction between zein and FA include Ser61, Gln195, and Asn207. Moreover, more than 10 other residues, such as Agr60, Leu64, Phe199, and Phe210, exhibit energy contributions greater than −4 kJ/mol (−1 kcal/mol), collectively forming the binding pocket of zein-FA.
Conclusion
In this study, a variety of experimental methods, including multi-spectroscopy, DLS, SEM, and TEM, were applied to investigate the interaction between zein and FA. Meanwhile, the binding mode and mechanism of zein-FA interaction were probed through molecular docking and MD simulation. The findings from experimental methods and molecular simulation were consistent. The increased absorption and redshift of the UV – Vis spectra suggested the formation of zein – FA complexes. Drawing from the outcomes of fluorescence analysis and molecular docking, it was ascertained that FA forms binding interactions with zein primarily through van der Waals forces and hydrogen bonding, as evidenced by the negative value of the free energy change (ΔG). The fluorescence findings additionally unveiled that FA led to a reduction in the fluorescence intensity of zein, indicating a static quenching mechanism-dominated at play. Moreover, FTIR results underscored the significance of hydrogen bonding and electrostatic interactions in the complex interactions, with several newly detected peaks providing confirmation of a robust bonding between zein and FA, as opposed to mere physical attachment. CD analysis demonstrated that the binding of FA gave rise to secondary structure alterations accompanied by a decrease in the a-helix content and an increase in the β-sheet content. Rg also confirmed the expansion of the protein structure. The particle size and zeta potential change as a result of structural alterations, improving the stability of the complex system; nonetheless, high FA concentrations cause protein aggregation. These findings were further confirmed by SEM and TEM analyses. Zein improved the photostability of FA against UV radiation. Molecular docking and MD simulations indicated that the FA molecule was embedded into the hydrophobic cavity of zein, primarily through van der Waals forces, hydrogen bonding, and hydrophobic interactions. The MM/PBSA results showed that FA could bind with zein with a high binding free energy (−146.19 ± 13.87 kJ/mol), indicating that the two substances were strongly related. These findings provide valuable theoretical support for the development of the plant protein zein as an efficient carrier for FA.
Author’s contributions
Jun Zhu: Conceptualization, Investigation, Data curation, Writing-original draft, Writing-review & editing. Wenlin Sun: Methodology, Investigation. Wenqi Zhang: Software, Data curation. Nuo Hong: Formal analysis, Visualization, Data curation. Yuying Liao: Investigation, Formal analysis, Data curation. Yiling Yao: Investigation, Data curation, Visualization. Lala Cai: Investigation, Data curation, Visualization. Chunhua Xiong: Supervision, Project administration, Writing-review & editing. Lanying Yao: Supervision, Funding acquisition, Writing-review & editing, Project administration.
Supplemental Material
Download MS Word (268.3 KB)Acknowledgments
Financial support from the Key Research and Development Program of Zhejiang Province (No. 2018C02045) and the Department of Science and Technology of Zhejiang Province, China (No. LGN19B040001) is gratefully acknowledged.
Disclosure statement
No potential conflict of interest was reported by the author(s).
Data availability statement
The data that support the findings of this study are available from the corresponding author upon reasonable request.
Supplementary material
Supplemental data for this article can be accessed online at https://doi.org/10.1080/10942912.2024.2357609
Additional information
Funding
References
- Chungchunlam, S. M. S.; Moughan, P. J. Comparative Bioavailability of Vitamins in Human Foods Sourced from Animals and Plants. Crit. Rev. Food Sci. Nutr. 2023, 1–36. DOI: 10.1080/10408398.2023.2241541.
- Zheng, Y.; Cantley, L. C. Toward a Better Understanding of Folate Metabolism in Health and Disease. J. Exp. Med. 2018, 216(2), 253–266. DOI: 10.1084/jem.20181965.
- Ducker, G. S.; Rabinowitz, J. D. One-Carbon Metabolism in Health and Disease. Cell Metab. 2017, 25(1), 27–42. DOI: 10.1016/j.cmet.2016.08.009.
- Stover, P. J. Physiology of Folate and Vitamin B 12 in Health and Disease. Nutr. rev. 2004, 62(suppl_1), S3–S12. DOI: 10.1111/j.1753-4887.2004.tb00070.x.
- Zhao, R.; Aluri, S.; Goldman, I. D. The Proton-Coupled Folate Transporter (PCFT-SLC46A1) and the Syndrome of Systemic and Cerebral Folate Deficiency of Infancy: Hereditary Folate Malabsorption. Mol. Aspects Med. 2017, 53, 57–72. DOI: 10.1016/j.mam.2016.09.002.
- Scott, J.; Rébeillé, F.; Fletcher, J. Folic Acid and Folates: The Feasibility for Nutritional Enhancement in Plant Foods. J. Sci. Food Agric. 2000, 80(7), 795–824. DOI: 10.1002/(SICI)1097-0010(20000515)80:7<795:AID-JSFA599>3.0.CO;2-K.
- Nguyen, M. T.; Indrawati; Hendrickx, M. Model Studies on the Stability of Folic Acid and 5-Methyltetrahydrofolic Acid Degradation During Thermal Treatment in Combination with High Hydrostatic Pressure. J. Agric. Food. Chem. 2003, 51(11), 3352–3357. DOI: 10.1021/jf026234e.
- Borradale, D. C.; Kimlin, M. G. Folate Degradation Due to Ultraviolet Radiation: Possible Implications for Human Health and Nutrition. Nutr. rev. 2012, 70(7), 414–422. DOI: 10.1111/j.1753-4887.2012.00485.x.
- Zema, P.; Pilosof, A. M. R. On the Binding of Folic Acid to Food Proteins Performing As Vitamin Micro/Nanocarriers. Food Hydrocolloids 2018, 79, 509–517. DOI: 10.1016/j.foodhyd.2018.01.021.
- Bourassa, P.; Hasni, I.; Tajmir-Riahi, H. A. Folic Acid Complexes with Human and Bovine Serum Albumins. Food Chem. 2011, 129(3), 1148–1155. DOI: 10.1016/j.foodchem.2011.05.094.
- Liang, L.; Zhang, J.; Zhou, P.; Subirade, M. Protective Effect of Ligand-Binding Proteins Against Folic Acid Loss Due to Photodecomposition. Food Chem. 2013, 141(2), 754–761. DOI: 10.1016/j.foodchem.2013.03.044.
- Zhang, J.; Cui, H.; Qiu, J.; Wang, X.; Zhong, Y.; Yao, C.; Yao, L.; Zheng, Q.; Xiong, C. Stability of Glycosylated Complexes Loaded with Epigallocatechin 3-Gallate (EGCG). Food Chem. 2023, 410, 135364. DOI: 10.1016/j.foodchem.2022.135364.
- Zhang, J.; Liu, Y.; Liu, X.; Li, Y.; Yin, X.; Subirade, M.; Zhou, P.; Liang, L. The Folic acid/β-Casein Complex: Characteristics and Physicochemical Implications. Food. Res. Int. 2014, 57, 162–167. DOI: 10.1016/j.foodres.2014.01.019.
- Cen, C.; Chen, J.; Wang, W.; Zhang, J.; Yang, X.; Fu, L.; Wang, Y. Exploring the Interaction Mechanism of Dietary Protein Ovalbumin and Folic Acid: A Combination Research of Molecular Simulation Technology and Multispectroscopy. Food Chem. 2022, 385, 132536. DOI: 10.1016/j.foodchem.2022.132536.
- Bustos, L. F.; Judis, M. A.; Vasile, F. E.; Pérez, O. E. Molecular Interactions Involved in the Complexation Process Between Buffalo Whey Proteins Concentrate and Folic Acid. Food Chem. 2022, 396, 133734. DOI: 10.1016/j.foodchem.2022.133734.
- Aprodu, I.; Dumitrașcu, L.; Râpeanu, G.; Bahrim, G.-E.; Stănciuc, N. Spectroscopic and Molecular Modeling Investigation on the Interaction Between Folic Acid and Bovine Lactoferrin from Encapsulation Perspectives. Foods. 2020, 9(6), 744. DOI: 10.3390/foods9060744.
- Zhang, Y.; Cui, L.; Che, X.; Zhang, H.; Shi, N.; Li, C.; Chen, Y.; Kong, W. Zein-Based Films and Their Usage for Controlled Delivery: Origin, Classes and Current Landscape. J. Controlled Release. 2015, 206, 206–219. DOI: 10.1016/j.jconrel.2015.03.030.
- Dong, J.; Sun, Q.; Wang, J.-Y. Basic Study of Corn Protein, Zein, As a Biomaterial in Tissue Engineering, Surface Morphology and Biocompatibility. Biomaterials. 2004, 25(19), 4691–4697. DOI: 10.1016/j.biomaterials.2003.10.084.
- Kiran, F.; Afzaal, M.; Shahid, H.; Saeed, F.; Ahmad, A.; Ateeq, H.; Islam, F.; Yousaf, H.; Shah, Y. A.; Nayik, G. A., et al. Effect of Protein-Based Nanoencapsulation on Viability of Probiotic Bacteria Under Hostile Conditions. Int. J. Food. Prop. 2023, 26(1), 1698–1710. DOI: 10.1080/10942912.2023.2228514.
- Momany, F. A.; Sessa, D. J.; Lawton, J. W.; Selling, G. W.; Hamaker, S. A.; Willett, J. L. Structural Characterization of Alpha-Zein. J. Agric. Food. Chem. 2006, 54(2), 543–547. DOI: 10.1021/jf058135h.
- Kale, A.; Zhu, F.; Cheryan, M. Separation of High-Value Products from Ethanol Extracts of Corn by Chromatography. Ind. Crops Prod. 2007, 26(1), 44–53. DOI: 10.1016/j.indcrop.2007.01.006.
- Xie, H.; Liu, C.; Gao, J.; Shi, J.; Ni, F.; Luo, X.; He, Y.; Ren, G.; Luo, Z. Fabrication of Zein-Lecithin-EGCG Complex Nanoparticles: Characterization, Controlled Release in Simulated Gastrointestinal Digestion. Food Chem. 2021, 365, 130542. DOI: 10.1016/j.foodchem.2021.130542.
- Heep, G.; Almeida, A.; Marcano, R.; Vieira, D.; Mainardes, R. M.; Khalil, N. M.; Sarmento, B. Zein-Casein-Lysine Multicomposite Nanoparticles Are Effective in Modulate the Intestinal Permeability of Ferulic Acid. Int. J. Biol. Macromol. 2019, 138, 244–251. DOI: 10.1016/j.ijbiomac.2019.07.030.
- Li, Z.; Wang, Y.; Song, B.; Li, J.; Bao, Y.; Jiang, Q.; Chen, Y.; Yang, S.; Yang, Y.; Tian, J., et al. The Comparison Between Zein-Anthocyanins Complex and Nanoparticle Systems: Stability Enhancement, Interaction Mechanism, and in silico Approaches. Food Chem. 2023, 420, 136136. DOI: 10.1016/j.foodchem.2023.136136.
- Ding, R.; Zhang, M.; Zhu, Q.; Qu, Y.; Jia, X.; Yin, L. Curcumin Loaded Zein-Alginate Nanogels with “Core-shell” Structure: Formation, Characterization and Simulated Digestion. Int. J.Biol. Macromol. 2023, 251, 126201. DOI: 10.1016/j.ijbiomac.2023.126201.
- Bao, X.; Rong, S.; Fu, Q.; Liu, H.; Han, Y.; Liu, F.; Ye, Z.; Chen, S. Zein-Yeast Carboxymethyl Glucan Particles Formed by Anti-Solvent Precipitation for Encapsulating Resveratrol. Int. J. Biol. Macromol. 2023, 253, 127557. DOI: 10.1016/j.ijbiomac.2023.127557.
- Wang, Q.; Tang, Y.; Yang, Y.; Lei, L.; Lei, X.; Zhao, J.; Zhang, Y.; Li, L.; Wang, Q.; Ming, J. Interactions and Structural Properties of Zein/Ferulic Acid: The Effect of Calcium Chloride. Food Chem. 2022, 373, 131489. DOI: 10.1016/j.foodchem.2021.131489.
- Liu, C.; Lv, N.; Ren, G.; Wu, R.; Wang, B.; Cao, Z.; Xie, H. Explore the Interaction Mechanism Between Zein and EGCG Using Multi-Spectroscopy and Molecular Dynamics Simulation Methods. Food Hydrocolloids. 2021, 120, 106906. DOI: 10.1016/j.foodhyd.2021.106906.
- Wang, Q.; Tang, Y.; Yang, Y.; Lei, L.; Lei, X.; Zhao, J.; Zhang, Y.; Li, L.; Wang, Q.; Ming, J. The Interaction Mechanisms, and Structural Changes of the Interaction Between Zein and Ferulic Acid Under Different pH Conditions. Food Hydrocolloids. 2022, 124, 107251. DOI: 10.1016/j.foodhyd.2021.107251.
- Tiwari, P.; Ali, R.; Ishrat, R.; Arfin, N. Study of Interaction Between Zein and Curcumin Using Spectroscopic and in silico Techniques. J. Mol. Struct. 2021, 1230, 129637. DOI: 10.1016/j.molstruc.2020.129637.
- Joye, I. J.; Davidov-Pardo, G.; Ludescher, R. D.; McClements, D. J. Fluorescence Quenching Study of Resveratrol Binding to Zein and Gliadin: Towards a More Rational Approach to Resveratrol Encapsulation Using Water-Insoluble Proteins. Food Chem. 2015, 185, 261–267. DOI: 10.1016/j.foodchem.2015.03.128.
- Kaffash, M.; Tolou-Shikhzadeh-Yazdi, S.; Soleimani, S.; Hoseinpoor, S.; Saberi, M. R.; Chamani, J. Spectroscopy and Molecular Simulation on the Interaction of Nano-Kaempferol Prepared by Oil-In-Water with Two Carrier Proteins: An Investigation of Protein–Protein Interaction. Spectrochim. Acta. Part. A. 2024, 309, 123815. DOI: 10.1016/j.saa.2023.123815.
- Liu, F.; Ma, C.; McClements, D. J.; Gao, Y. A Comparative Study of Covalent and Non-Covalent Interactions Between Zein and Polyphenols in Ethanol-Water Solution. Food Hydrocolloids. 2017, 63, 625–634. DOI: 10.1016/j.foodhyd.2016.09.041.
- Peñalva, R.; Esparza, I.; González-Navarro, C. J.; Quincoces, G.; Peñuelas, I.; Irache, J. M. Zein Nanoparticles for Oral Folic Acid Delivery. J. Drug Delivery Sci. Technol. 2015, 30, 450–457. DOI: 10.1016/j.jddst.2015.06.012.
- Tabasi, M.; Maghami, P.; Amiri-Tehranizadeh, Z.; Reza Saberi, M.; Chamani, J. New Perspective of the Ternary Complex of Nano-Curcumin with β-Lactoglobulin in the Presence of α-Lactalbumin: Spectroscopic and Molecular Dynamic Investigations. J. Mol. Liq. 2023, 392, 123472. DOI: 10.1016/j.molliq.2023.123472.
- Bansal, M.; Singh, N.; Pal, S.; Dev, I.; Ansari, K. M. Chapter Three - Chemopreventive Role of Dietary Phytochemicals in Colorectal Cancer. In Advances in Molecular Toxicology; Elsevier: 2018; Vol. 12, pp 69–121. DOI: 10.1016/B978-0-444-64199-1.00004-X.
- Malek-Esfandiari, Z.; Rezvani-Noghani, A.; Sohrabi, T.; Mokaberi, P.; Amiri-Tehranizadeh, Z.; Chamani, J. Molecular Dynamics and Multi-Spectroscopic of the Interaction Behavior Between Bladder Cancer Cells and Calf Thymus DNA with Rebeccamycin: Apoptosis Through the Down Regulation of PI3K/AKT Signaling Pathway. J. Fluoresc. 2023, 33(4), 1537–1557. DOI: 10.1007/s10895-023-03169-4.
- Taheri, R.; Hamzkanlu, N.; Rezvani, Y.; Niroumand, S.; Samandar, F.; Amiri-Tehranizadeh, Z.; Saberi, M. R.; Chamani, J. Exploring the HSA/DNA/Lung Cancer Cells Binding Behavior of P-Synephrine, a Naturally Occurring Phenyl Ethanol Amine with Anti-Adipogenic Activity: Multi Spectroscopic, Molecular Dynamic and Cellular Approaches. J. Mol. Liq. 2022, 368, 120826. DOI: 10.1016/j.molliq.2022.120826.
- Abdollahi, K.; Ince, C.; Condict, L.; Hung, A.; Kasapis, S. Combined Spectroscopic and Molecular Docking Study on the pH Dependence of Molecular Interactions Between β-Lactoglobulin and Ferulic Acid. Food Hydrocolloids. 2020, 101, 105461. DOI: 10.1016/j.foodhyd.2019.105461.
- Bakar, K. A.; Feroz, S. R. A Critical View on the Analysis of Fluorescence Quenching Data for Determining Ligand–Protein Binding Affinity. Spectrochim. Acta. Part. A. 2019, 223, 117337. DOI: 10.1016/j.saa.2019.117337.
- Farajzadeh-Dehkordi, N.; Farhadian, S.; Zahraei, Z.; Gholamian-Dehkordi, N.; Shareghi, B. Interaction of Reactive Red195 with Human Serum Albumin: Determination of the Binding Mechanism and Binding Site by Spectroscopic and Molecular Modeling Methods. J. Mol. Liq. 2021, 327, 114835. DOI: 10.1016/j.molliq.2020.114835.
- Ying, M.; Huang, F.; Ye, H.; Xu, H.; Shen, L.; Huan, T.; Huang, S.; Xie, J.; Tian, S.; Hu, Z., et al. Study on Interaction Between Curcumin and Pepsin by Spectroscopic and Docking Methods. Int. J. Biol. Macromol. 2015, 79, 201–208. DOI: 10.1016/j.ijbiomac.2015.04.057.
- Yang, Z.; Zeng, X.; Zhao, Y.; Chen, R. AlphaFold2 and Its Applications in the Fields of Biology and Medicine. Signal Trans. Tar. Ther 2023, 8(1), 115. DOI: 10.1038/s41392-023-01381-z.
- Tan, H.; Zhou, H.; Guo, T.; Zhang, Y.; Ma, L. Integrated Multi-Spectroscopic and Molecular Modeling Techniques to Study the Formation Mechanism of Hidden Zearalenone in Maize. Food Chem. 2021, 351, 129286. DOI: 10.1016/j.foodchem.2021.129286.
- Halgren, T. A. Merck Molecular Force Field. I. Basis, Form, Scope, Parameterization, and Performance of MMFF94. J. Comput. Chem. 1996, 17(5–6), 490–519. DOI: 10.1002/(SICI)1096-987X(199604)17:5/6<490:AID-JCC1>3.0.CO;2-P.
- Kruse, H.; Goerigk, L.; Grimme, S. Why the Standard B3LYP/6-31G* Model Chemistry Should Not Be Used in DFT Calculations of Molecular Thermochemistry: Understanding and Correcting the Problem. J. Org. Chem. 2012, 77(23), 10824–10834. DOI: 10.1021/jo302156p.
- Pettersen, E. F.; Goddard, T. D.; Huang, C. C.; Couch, G. S.; Greenblatt, D. M.; Meng, E. C.; Ferrin, T. E. UCSF Chimera–A Visualization System for Exploratory Research and Analysis. J. Comput. Chem. 2004, 25(13), 1605–1612. DOI: 10.1002/jcc.20084.
- Hornak, V.; Abel, R.; Okur, A.; Strockbine, B.; Roitberg, A.; Simmerling, C. Comparison of Multiple Amber Force Fields and Development of Improved Protein Backbone Parameters. Proteins Struct. Funct. Bioinf. 2006, 65(3), 712–725. DOI: 10.1002/prot.21123.
- Wang, J.; Wolf, R. M.; Caldwell, J. W.; Kollman, P. A.; Case, D. A. Development and Testing of a General Amber Force Field. J. Comput. Chem. 2004, 25(9), 1157–1174. DOI: 10.1002/jcc.20035.
- Hess, B.; Bekker, H.; Berendsen, H. J. C.; Fraaije, J. G. E. M. LINCS: A Linear Constraint Solver for Molecular Simulations. J. Comput. Chem. 1997, 18(12), 1463–1472. DOI: 10.1002/(SICI)1096-987X(199709)18:12<1463:AID-JCC4>3.0.CO;2-H.
- Miyamoto, S.; Kollman, P. A. Settle: An Analytical Version of the SHAKE and RATTLE Algorithm for Rigid Water Models. J. Comput. Chem. 1992, 13(8), 952–962. DOI: 10.1002/jcc.540130805.
- Lu, Q.; Chen, C.; Zhao, S.; Ge, F.; Liu, D. Investigation of the Interaction Between Gallic Acid and α-Amylase by Spectroscopy. Int. J. Food. Prop. 2016, 19(11), 2481–2494. DOI: 10.1080/10942912.2015.1059345.
- Baibarac, M.; Smaranda, I.; Nila, A.; Serbschi, C. Optical Properties of Folic Acid in Phosphate Buffer Solutions: The Influence of pH and UV Irradiation on the UV-VIS Absorption Spectra and Photoluminescence. Sci. Rep. 2019, 9(1), 14278. DOI: 10.1038/s41598-019-50721-z.
- Kaur, L.; Rahman, A. J.; Singh, A.; Pathak, M.; Datta, A.; Singhal, R.; Ojha, H. Binding Studies for the Interaction Between Hazardous Organophosphorus Compound Phosmet and Lysozyme: Spectroscopic and in-Silico Analyses. J. Mol. Liq. 2022, 355, 118954. DOI: 10.1016/j.molliq.2022.118954.
- Arroyo-Maya, I. J.; Campos-Terán, J.; Hernández-Arana, A.; McClements, D. J. Characterization of Flavonoid-Protein Interactions Using Fluorescence Spectroscopy: Binding of Pelargonidin to Dairy Proteins. Food Chem. 2016, 213, 431–439. DOI: 10.1016/j.foodchem.2016.06.105.
- Aprodu, I.; Ursache, F.-M.; Turturică, M.; Râpeanu, G.; Stănciuc, N. Thermal Stability of the Complex Formed Between Carotenoids from Sea Buckthorn (Hippophae Rhamnoides L.) and Bovine β-Lactoglobulin. Spectrochim. Acta. Part. A. 2017, 173, 562–571. DOI: 10.1016/j.saa.2016.10.010.
- Shukla, R.; Cheryan, M. Zein: The Industrial Protein from Corn. Ind. Crops Prod. 2001, 13(3), 171–192. DOI: 10.1016/S0926-6690(00)00064-9.
- Kalhori, F.; Yazdyani, H.; Khademorezaeian, F.; Hamzkanloo, N.; Mokaberi, P.; Hosseini, S.; Chamani, J. Enzyme Activity Inhibition Properties of New Cellulose Nanocrystals from Citrus Medica L. Pericarp: A Perspective of Cholesterol Lowering. Luminescence. 2022, 37(11), 1836–1845. DOI: 10.1002/bio.4360.
- Papadopoulou, A.; Green, R. J.; Frazier, R. A. Interaction of Flavonoids with Bovine Serum Albumin: A Fluorescence Quenching Study. J. Agric. Food. Chem. 2005, 53(1), 158–163. DOI: 10.1021/jf048693g.
- Rezaei, S.; Meftah, H.-S.; Ebtehajpour, Y.; Rahimi, H. R.; Chamani, J. Investigation on the Effect of Fluorescence Quenching of Calf Thymus DNA by Piperine: Caspase Activation in the Human Breast Cancer Cell Line Studies. DNA Cell Biol. 2023, 43(1), 26–38. DOI: 10.1089/dna.2023.0269.
- Mohammadi, M.; Shareghi, B.; Akbar Saboury, A. Comparative Studies on the Interaction of Spermidine with Carboxypeptidase a Using Multispectroscopic and Docking Methods. Int. J. Biol. Macromol. 2020, 147, 821–831. DOI: 10.1016/j.ijbiomac.2019.09.242.
- Shinde, M.; Kale, K.; Kumar, K.; Ottoor, D. Effect of Quercetin on the Amiloride–Bovine Serum Albumin Interaction Using Spectroscopic Methods, Molecular Docking and Chemometric Approaches. Luminescence. 2021, 36(1), 129–141. DOI: 10.1002/bio.3926.
- Asgharzadeh, S.; Shareghi, B.; Farhadian, S. Structural Alterations and Inhibition of Lysozyme Activity Upon Binding Interaction with Rotenone: Insights from Spectroscopic Investigations and Molecular Dynamics Simulation. Int. J. Biol. Macromol. 2024, 254, 127831. DOI: 10.1016/j.ijbiomac.2023.127831.
- Condict, L.; Kasapis, S. Critical Issues Encountered in the Analysis of Protein-Phenolic Binding Interactions via Fluorescence Spectroscopy. Food Hydrocolloids. 2022, 124, 107219. DOI: 10.1016/j.foodhyd.2021.107219.
- Sharifi-Rad, A.; Mehrzad, J.; Darroudi, M.; Saberi, M. R.; Chamani, J. Oil-In-Water Nanoemulsions Comprising Berberine in Olive Oil: Biological Activities, Binding Mechanisms to Human Serum Albumin or Holo-Transferrin and QMMD Simulations. J. Biomol. Struct. Dyn. 2021, 39(3), 1029–1043. DOI: 10.1080/07391102.2020.1724568.
- Cabra, V.; Arreguin, R.; Vazquez-Duhalt, R.; Farres, A. Effect of Temperature and pH on the Secondary Structure and Processes of Oligomerization of 19 kDa Alpha-Zein. Biochim. Biophys. Acta Proteins Proteom. 2006, 1764(6), 1110–1118. DOI: 10.1016/j.bbapap.2006.04.002.
- Chilom, C. G.; Bacalum, M.; Stanescu, M. M.; Florescu, M. Insight into the Interaction of Human Serum Albumin with Folic Acid: A Biophysical Study. Spectrochim. Acta. Part. A. 2018, 204, 648–656. DOI: 10.1016/j.saa.2018.06.093.
- Raeessi-Babaheydari, E.; Farhadian, S.; Shareghi, B. Evaluation of Interaction Between Citrus Flavonoid, Naringenin, and Pepsin Using Spectroscopic Analysis and Docking Simulation. J. Mol. Liq. 2021, 339, 116763. DOI: 10.1016/j.molliq.2021.116763.
- Farhadian, S.; Shareghi, B.; Saboury, A. A.; Momeni, L. Counteraction of Lactose on the Thermal Stability and Activity of α-Chymotrypsin: Thermodynamic, Kinetic and Docking Studies. Rsc. Adv. 2016, 6(76), 72201–72212. DOI: 10.1039/C6RA11833J.
- Sadeghi-Kaji, S.; Shareghi, B.; Saboury, A. A.; Farhadian, S. Spectroscopic and Molecular Docking Studies on the Interaction Between Spermidine and Pancreatic Elastase. Int. J. Biol. Macromol. 2019, 131, 473–483. DOI: 10.1016/j.ijbiomac.2019.03.084.
- Rabbani, G.; Lee, E. J.; Ahmad, K.; Baig, M. H.; Choi, I. Binding of Tolperisone Hydrochloride with Human Serum Albumin: Effects on the Conformation, Thermodynamics, and Activity of HSA. Mol. Pharmaceutics. 2018, 15(4), 1445–1456. DOI: 10.1021/acs.molpharmaceut.7b00976.
- Subramanian, S.; Ross, J. B. A.; Ross, P. D.; Brand, L. Investigation of the Nature of Enzyme-Coenzyme Interactions in Binary and Ternary Complexes of Liver Alcohol Dehydrogenase with Coenzymes, Coenzyme Analogs, and Substrate Analogs by Ultraviolet Absorption and Phosphorescence Spectroscopy. Biochemistry. 1981, 20(14), 4086–4093. DOI: 10.1021/bi00517a022.
- Zhu, J.; Yao, Y.; Liao, Y.; Cai, L.; Zhang, W.; Hong, N.; Sun, W.; Xiong, C.; Yao, L. The Interaction Mechanism Between Zein and Folic Acid in Alkaline Aqueous Solutions: An Experimental and Molecular Simulation Study. Food Qual. Saf. 2024, 8, fyad064. DOI: 10.1093/fqsafe/fyad064.
- He, Y. Y.; Wang, X. C.; Jin, P. K.; Zhao, B.; Fan, X. Complexation of Anthracene with Folic Acid Studied by FTIR and UV Spectroscopies. Spectrochim. Acta. Part. A. 2009, 72(4), 876–879. DOI: 10.1016/j.saa.2008.12.021.
- Liu, F.; Sun, C.; Yang, W.; Yuan, F.; Gao, Y. Structural Characterization and Functional Evaluation of Lactoferrin-Polyphenol Conjugates Formed by Free-Radical Graft Copolymerization. R.S.C. Adv 2015, 5(20), 15641–15651. DOI: 10.1039/C4RA10802G.
- Zang, Z.; Tian, J.; Chou, S.; Lang, Y.; Tang, S.; Yang, S.; Yang, Y.; Jin, Z.; Chen, W.; Liu, X., et al. Investigation on the Interaction Mechanisms for Stability of Preheated Whey Protein Isolate with Anthocyanins from Blueberry. Int. J. Biol. Macromol. 2024, 255, 127880. DOI: 10.1016/j.ijbiomac.2023.127880.
- Corfield, R.; Martínez, K. D.; Allievi, M. C.; Santagapita, P.; Mazzobre, F.; Schebor, C.; Pérez, O. E. Whey Proteins-Folic Acid Complexes: Formation, Isolation and Bioavailability in a Lactobacillus Casei Model. Food Struct. 2020, 26, 100162. DOI: 10.1016/j.foostr.2020.100162.
- Su, J.; Guo, Q.; Chen, Y.; Mao, L.; Gao, Y.; Yuan, F. Utilization of β-lactoglobulin- (−)-Epigallocatechin- 3-gallate(EGCG) composite colloidal nanoparticles as stabilizers for lutein pickering emulsion. Food Hydrocolloids 2020, 98. DOI: 10.1016/j.foodhyd.2019.105293.
- Wei, Y.; Li, C.; Zhang, L.; Dai, L.; Yang, S.; Liu, J.; Mao, L.; Yuan, F.; Gao, Y. Influence of Calcium Ions on the Stability, Microstructure and in vitro Digestion Fate of Zein-Propylene Glycol Alginate-Tea Saponin Ternary Complex Particles for the Delivery of Resveratrol. Food Hydrocolloids. 2020, 106, 105886. DOI: 10.1016/j.foodhyd.2020.105886.
- Guerrero, P.; Retegi, A.; Gabilondo, N.; de la Caba, K. Mechanical and Thermal Properties of Soy Protein Films Processed by Casting and Compression. J. Food Eng. 2010, 100(1), 145–151. DOI: 10.1016/j.jfoodeng.2010.03.039.
- Olsson, T. S. G.; Williams, M. A.; Pitt, W. R.; Ladbury, J. E. The Thermodynamics of Protein–Ligand Interaction and Solvation: Insights for Ligand Design. J. Mol. Biol. 2008, 384(4), 1002–1017. DOI: 10.1016/j.jmb.2008.09.073.
- Selling, G. W.; Hamaker, S. A. H.; Sessa, D. J. Effect of Solvent and Temperature on Secondary and Tertiary Structure of Zein by Circular Dichroism. Cereal Chem. 2007, 84(3), 265–270. DOI: 10.1094/CCHEM-84-3-0265.
- Maheri, H.; Hashemzadeh, F.; Shakibapour, N.; Kamelniya, E.; Malaekeh-Nikouei, B.; Mokaberi, P.; Chamani, J. Glucokinase Activity Enhancement by Cellulose Nanocrystals Isolated from Jujube Seed: A Novel Perspective for Type II Diabetes Mellitus Treatment (In Vitro). J. Mol. Struct. 2022, 1269, 133803. DOI: 10.1016/j.molstruc.2022.133803.
- Liu, M.; Zhu, L.; Qu, X.; Sun, D.; Li, L.; Lin, R. Studies on the Binding of Paeonol and Two of Its Isomers to Human Serum Albumin by Using Microcalorimetry and Circular Dichroism. J. Chem. Thermodyn. 2007, 39(12), 1565–1570. DOI: 10.1016/j.jct.2007.05.003.
- Lefèvre, T.; Subirade, M. Molecular Differences in the Formation and Structure of Fine-Stranded and Particulate β-Lactoglobulin Gels. Biopolymers. 2000, 54(7), 578–586. DOI: 10.1002/1097-0282(200012)54:7<578:AID-BIP100>3.0.CO;2-2.
- Chamani, J.; Heshmati, M. Mechanism for Stabilization of the Molten Globule State of Papain by Sodium N-Alkyl Sulfates: Spectroscopic and Calorimetric Approaches. J. Colloid. Interface. Sci. 2008, 322(1), 119–127. DOI: 10.1016/j.jcis.2008.03.001.
- Moosavi-Movahedi, A. A.; Chamani, J.; Ghourchian, H.; Shafiey, H.; Sorenson, C. M.; Sheibani, N. Electrochemical Evidence for the Molten Globule States of Cytochrome C Induced by N-Alkyl Sulfates at Low Concentrations. J. Protein Chem. 2003, 22(1), 23–30. DOI: 10.1023/a:1023011609931.
- Wang, L.; Wen, J.; Wang, L.; Jiang, L.; Zhang, Y.; Sui, X. Characterization of the Extreme pH-Induced Molten Globule State of Soy Protein Isolate and Its Influence on Functional Properties. Food Hydrocolloids. 2023, 144, 109040. DOI: 10.1016/j.foodhyd.2023.109040.
- Liu, C.; Lv, N.; Song, Y.; Dong, L.; Huang, M.; Shen, Q.; Ren, G.; Wu, R.; Wang, B.; Cao, Z., et al. Interaction Mechanism Between Zein and β-Lactoglobulin: Insights from Multi-Spectroscopy and Molecular Dynamics Simulation Methods. Food Hydrocolloids. 2023, 135, 108226. DOI: 10.1016/j.foodhyd.2022.108226.
- Lu, Y.; Zhao, R.; Wang, C.; Zhang, X.; Wang, C. Deciphering the Non-Covalent Binding Patterns of Three Whey Proteins with Rosmarinic Acid by Multi-Spectroscopic, Molecular Docking and Molecular Dynamics Simulation Approaches. Food Hydrocolloids. 2022, 132, 107895. DOI: 10.1016/j.foodhyd.2022.107895.
- Monhemi, H.; Housaindokht, M. R.; Nakhaei Pour, A. Effects of Natural Osmolytes on the Protein Structure in Supercritical CO2: Molecular Level Evidence. J. Phys. Chem B. 2015, 119(33), 10406–10416. DOI: 10.1021/acs.jpcb.5b03970.
- Sun, F.; Wang, H.; Wang, H.; Xia, X.; Kong, B. Impacts of pH and Temperature on the Conformation of a Protease from Pediococcus Pentosaceus R1 Isolated from Harbin Dry Sausage. LWT. 2021, 142, 111056. DOI: 10.1016/j.lwt.2021.111056.
- Ceruso, M. A.; Amadei, A.; Nola, A. D. Mechanics and Dynamics of B1 Domain of Protein G: Role of Packing and Surface Hydrophobic Residues. Protein Sci. 1999, 8(1), 147–160. DOI: 10.1110/ps.8.1.147.
- Lobanov, M. Y.; Bogatyreva, N. S.; Galzitskaya, O. V. Radius of Gyration As an Indicator of Protein Structure Compactness. Mol. Biol 2008, 42(4), 623–628. DOI: 10.1134/S0026893308040195.
- Wu, X.; Zhao, X.; Deng, Z.; Liang, X.; Fang, S. Investigation of Interactions Between Zein and Natamycin by Fluorescence Spectroscopy and Molecular Dynamics Simulation. J. Mol. Liq 327, 114873. DOI: 10.1016/j.molliq.2020.114873.
- Valdés-Tresanco, M. S.; Valdés-Tresanco, M. E.; Valiente, P. A.; Moreno, E. Gmx_mmpbsa: A New Tool to Perform End-State Free Energy Calculations with GROMACS. J. Chem. Theory Comput. 2021, 17(10), 6281–6291. DOI: 10.1021/acs.jctc.1c00645.
- Gohlke, H.; Kiel, C.; Case, D. A. Insights into Protein–Protein Binding by Binding Free Energy Calculation and Free Energy Decomposition for the Ras–Raf and Ras–RalGDS Complexes. J. Mol. Biol. 2003, 330(4), 891–913. DOI: 10.1016/S0022-2836(03)00610-7.