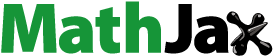
ABSTRACT
The study of infectious diseases includes both the progression of the disease in its host and how it transmits between hosts. Understanding disease transmission is important for recommending effective interventions, protecting healthcare workers, and informing an effective public health response. Sampling the environment for infectious diseases is critical to public health since it can provide an understanding of the mechanisms of transmission, characterization of contamination in hospitals and other public areas, and the spread of a disease within a community. Measurements of biological aerosols, particularly those that may cause disease, have been an ongoing topic of research for decades, and so a wide variety of technological solutions exist. This wide field of possibilities can create confusion, particularly when different approaches yield different answers. Therefore, guidelines for best practice in this area are important to allow more effective use of this data in public health decisions. This review examines air, surface and water/wastewater sampling methods, with a focus on aerosol sampling, and a goal of recommending approaches to designing and implementing sampling systems that may incorporate multiple strategies. This is accomplished by developing a framework for designing and evaluating a sampling strategy, reviewing current practices and emerging technologies for sampling and analysis, and recommending guidelines for best practice in the area of aerosol sampling for infectious disease.
Introduction
Joshua L. Santarpia
Environmental sampling has been used throughout the COVID-19 pandemic to identify routes of transmission, identify emerging outbreaks, and characterize contamination in a variety of environments. Although these goals have been given recent attention, the measurement methods have been used for many decades for a variety of purposes, from biodefense and remediation to the characterization of other infectious diseases. A wide variety of technologies and approaches have been used to accomplish these goals. Individually, each approach can provide useful information, and combinations of approaches are often necessary to provide more complete risk assessments.
When sampling an environment for an infectious disease, the focus is on identifying disease causing agents and characterizing their hazards to humans, animals and/or agriculture. This is distinct from efforts to characterize or understand the entire living ecosystem in an environment. In most cases, the focus of infectious disease sampling is on collecting and identifying viruses and bacteria, since they may be both found in the environment and can be communicable between hosts. While, in some cases, the techniques described here can be applied to other disease-causing agents, such as fungi and parasites, these other agents are not the focus of this review.
A significant area of this review focuses on measurement and sampling of infectious aerosols. This area of sampling has received recent attention, as it pertains so closely to understanding the transmission of respiratory diseases, such as influenza (Blachere et al. Citation2011) and SARS-CoV-2 (Tang et al. Citation2020). Several considerations must be made when considering sampling for these biogenic aerosols. The first is the relevant particle size. In many cases, the fundamental size of the virus (typically from 10 to several hundred nanometers) or bacteria (typically from 500 to several 1000 nanometers) is the primary consideration when determining how to sample for a pathogen (Hinds 1982). For infectious diseases, the particle size is likely driven by the source of the aerosol (e.g., human or animal respiratory emissions (Morawska et al. Citation2009)) rather than the size of the pathogen. Therefore, both the type of pathogen and the aerosol source must be considered in the design of an aerosol sampling system. The wide variety of potential methods to sample and measure biological aerosols creates uncertainty in the validity of any particular method. Therefore, guidelines for infectious aerosol sampling are needed.
Previous review articles have covered relevant topics in some depth, including biological aerosol sampling (Mainelis Citation2020; Santarpia Citation2016; Shammi, Mostafizur Rahman, and Mohammad Tareq Citation2021; Šantl-Temkiv et al. Citation2020), real-time monitoring of bioaerosols (Šantl-Temkiv et al. Citation2020; Cho et al. Citation2020; Huffman et al. Citation2020; Huffman and Joshua Citation2017) and bioaerosol hazards to human and animal health (Anderson et al. Citation2017; Kim, Kabir, and Ara Jahan Citation2018; Mubareka et al. Citation2019) Further, many established guidelines (Sehulster et al. Citation2004; Silvestri et al. Citation2021) and scientific reviews (Otter et al. Citation2013) are available for the application of surface sampling for infectious diseases. Likewise, technologies for monitoring wastewater for pathogens and other contaminants have also been the subject of past work (United States. Environmental Protection Agency. Region VII. Citation1974), and an expansion of the national wastewater monitoring system is being considered by the National Academies (Based Disease Surveillance for Public Health Action Citation2023).
The goals of this review are: i) to examine recent advances or applications of aerosol sampling technology, and provide an overview of wastewater and surface sampling techniques ii) to create a framework to design infectious disease sampling that may use multiple sampling modalities, iii) propose a straightforward guideline for sampling infectious aerosols, and iv) identify areas of future research.
Historical background
Probing the environment for microorganisms traces its roots back to the origin of the germ theory of disease and Louis Pasteur and John Tyndall in the 1860s and 1870s (Conant Citation1957). Although most often recognized for the process of pasteurization, which bears his name, Pasteur’s work to disprove the theory of spontaneous generation of life could be seen as the first intentional sampling of airborne microorganisms. In these experiments, Pasteur demonstrated that only sterilized broth that is exposed to airborne particles will grow bacteria. John Tyndall’s later experiments to create “optically pure” air similarly cultivated bacteria from airborne microorganisms. These early experiments relied solely on passive collection of microbial aerosols, a technique that is still in use. Sampling of water to detect the presence of bacteria associated with sewage was first reported in 1885 (Fewtrell and Bartram Citation2013). Fomites (materials to which infectious agents might adhere, including clothing, utensils, and furniture) have been recognized for centuries for their role in the transmission of disease (Clendening Citation1942). Routine sampling of fomites in hospitals was commonplace during the early 20th century but by the 1970s, following recommendations by both the U.S. Centers for Disease Control (CDC) and the American Hospital Association (AHA), routine, random sampling of hospital environments was replaced by targeted sampling (Sehulster et al. Citation2004).
Humanity has a long and dark history of using disease as a weapon (Beedham and Davies Citation2021). The threat of nefarious use of biological agents has prompted many countries, including the United States, to invest in technologies to monitor for and remediate a biological attack (Garza et al. Citation2005; Institute of Medicine US and National Research Council US Committee on Effectiveness of National Biosurveillance Systems: Biowatch and the Public Health System Citation2011). These include air sampling technologies that monitor for potential threats in real-time (DeFreez et al. Citation2005; Gieray et al. Citation1997; Hairston, Jim, and Quant Citation1997; Healy et al. Citation2014), as well as collection of aerosol samples for offline analyses (Dybwad, Skogan, and Martha Blatny Citation2014). Biological defense technologies also include approaches for evaluating the efficacy of remediation techniques, including surface sampling and trace detection of deposited material (Silvestri et al. Citation2021).
Environmental monitoring in hospitals is recommended by the U.S. CDC for: 1) outbreak investigation, 2) research, 3) monitoring of potentially hazardous conditions, 4) validating the effectiveness of remediation activities, and 5) quality assurance to validate changes in practice or new equipment (Sehulster et al. Citation2004). During the ongoing COVID-19 pandemic many hospitals engaged in environmental sampling to inform the development of effective personal protective equipment and patient isolation practices (Chia et al. Citation2020; Liu et al. Citation2020). Environmental sampling in public spaces is also a component of understanding public health risk. Much work has been done to understand transmission of influenza and other diseases in public schools (Coleman and Sigler Citation2020; Crowe et al. Citation2021; Fong et al. Citation2020), on airplanes (Nagda et al. Citation2000), and norovirus on cruise ships (Bert et al. Citation2014). During the COVID-19 pandemic, environmental sampling increased to characterize public health risk in many environments. These included hospital studies to confirm airborne transmission routes (Chia et al. Citation2020; Liu et al. Citation2020; Santarpia et al. Citation2020), studies of contamination in various hospital environments (Barksdale et al. Citation2021), studies on cruise ships where outbreaks had occurred (Yamagishi et al. Citation2020), investigation in various forms of transportation (Lednicky et al. Citation2021b), quarantine facilities (Santarpia et al. Citation2020), and homes (Laumbach et al. Citation2022) with the overall goal of informing risk of transmission in public settings. Wastewater monitoring was found to be a useful tool for diseases that are shed through excretory material. Monitoring outbreaks of diseases such as polio (Russo et al. Citation2022), noroviruses (Katayama et al. Citation2007) and COVID-19 (Wu et al. Citation2021) relied on the use of wastewater sampling. As with biodefense applications, a variety of tools and approaches have been developed to facilitate this work, some purpose made, and others repurposed from other endeavors.
Another area that has received attention is monitoring the natural environment for infectious disease. The World Organization for Animal Health recommends sampling for infectious diseases among animal populations to prevent the spread of diseases such as avian influenza (The World Organisation for Animal Health Citation2022). Zoonosis is a major source of epidemics and pandemics worldwide. Diseases such as human immunodeficiency virus (HIV), filoviruses, coronaviruses and even influenza epidemics all have zoonotic origins (Brockwell-Staats, Webster, and Webby Citation2009; Chitnis, Rawls, and Moore Citation2000; Cunha and Opal Citation2014; Saéz et al. Citation2015). Besides zoonosis, the spread of diseases among animal populations, like highly pathogenic avian influenza (HPAI) (Blachere et al. Citation2018; Machalaba et al. Citation2015), foot and mouth disease virus (FMV) (Jamal and Belsham Citation2013) and African swine fever virus (ASFV) (Galindo and Alonso Citation2017) can cause significant risk to domestic animals and livestock. ASFV, in particular, affects the economies of swine production around the world, especially in south-east Asia. To date, there is no efficacious vaccine or treatment available. Culling infected animals and consistent surveillance/monitoring are necessary to avoid further ASFV outbreaks. ASFV can be spread via direct contact, consumption of contaminated food, and fomite transmission. Gebhardt et al. describe swab sampling locations where ASFV could naturally be found in the environment (feed mill environment, feed ingredients, feed delivery trucks). Results indicated feed delivery trucks and worker clothing were positive for ASFV contamination. Following this information, procedures were changed to include the decontamination of feed delivery trucks and for workers to change clothing upon arrival at the mill (Gebhardt et al. Citation2021)
Monitoring for the next epidemic or pandemic disease relying on direct sampling of wild animal populations requires skilled veterinary practice and is outside the scope of this review. However, surface, water and wastewater and aerosol sampling may provide insight into the spread and decontamination of those diseases once the source has been identified (Blachere et al. Citation2018; Cao et al. Citation2011; Gebhardt et al. Citation2021; Kosowska et al. Citation2021).
A framework for environmental sampling design
This review presents a framework for developing an environmental sampling plan based on the answers to three key questions and the application of technologies to their answers. These questions are:
What is the purpose?
What actions will be taken?
What are the potential hazards?
Defining the purpose, hazards and follow-on actions allows the selection of the most appropriate approaches and technologies to ensure that the system can provide adequate and actionable data.
Defining the purpose
The reasons described above to engage in environmental sampling for infectious disease can be placed in five major categories (outer ring of ):
Figure 1. A graphical representation of the framework for developing and evaluating environmental sampling activities. The 3 key questions, as well as the general answers are provided in the rings. Graphical depictions of technologies that may be employed are shown in the center, including (from top left):surface sampling, air sampling, water sampling, culture, protein detection, nucleic acid characterization, and real time aerosol monitoring (top center).
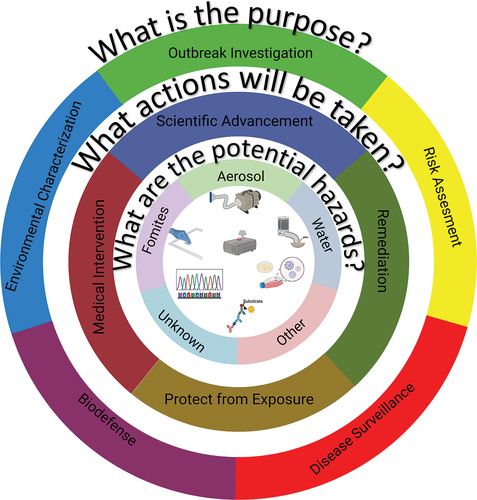
Outbreak investigation: The disease is often known, but not always, and the intent is usually to understand the transmission dynamics, stop or slow down the outbreak.
Risk assessment: The disease is generally known and the intent is to evaluate the potential risk of disease in a specific environment in order to enhance safety.
Disease surveillance: Known diseases in circulation are identified to help prepare the medical community. This is most often done by sampling the population directly, but certain types of environmental sampling are also used.
Biodefense: This type of sampling addresses intentional, nefarious exposures to disease-causing agents. Depending on the situation, this may involve protecting military and other populations from exposure to the disease or initiating treatments before symptom onset while life-saving measures can still be applied.
Environmental characterization: This sampling evaluates the ambient environment for unknown pathogens in both natural and built environments. Discovery of pathogens may motivate further sampling in one of the other categories (e.g., risk assessment).
Defining the actions
Defining the purpose for an environmental sampling strategy is the first step, but understanding how the data collected will be used is just as critical to the design of an environmental sampling plan. Actions taken based on the data collected can have a variety of consequences, and both the potential actions and their inherent consequences need to be considered in the design process. In this framework, the possible actions are collected into four categories (middle ring of ):
Scientific Advancement. The action taken with the least immediate impact is to use the data to add to the scientific understanding of the problem. Sampling studies often are undertaken to refine methods, develop new techniques, or characterize a different environment. These types of studies can have more flexibility than studies where higher consequence actions may be taken.
Remediation. Study results can be used to refine or improve protocols or to inform decontamination activities. A change in safety protocol, such as deciding to move away from airborne isolation precautions, must be based on sound data and accepted methods or it puts healthcare practitioners at risk. Remediation can be costly and time consuming, and poor or poorly understood data can lead to incomplete remediation or could lead to repeated, unnecessary remediation processes.
Protect from Exposure. Rapid action may be needed to protect a population from exposure. In the military context, exposure to a biological agent, even if it can be treated or may not be fatal, can have adverse consequences. Therefore, rapid action to protect soldiers from exposure is often desirable. The consequence of the action might be low (donning protective gear or evacuating a small area), but the consequence of missing a real event could be catastrophic.
Medical Interventions. Environmental sampling activities might lead to medical interventions. In the lowest consequence, this might be hospital preparedness for emerging influenza strains or SARS-CoV-2 variants. In the highest consequence, it could mean the distribution of antibiotics in the aftermath of an anthrax attack.
Defining the hazards
Finally, defining an environmental sampling plan must addresses what is known about the disease. First, is the causative agent known? There are many cases where the cause of a disease outbreak is unknown. During the emergence of a new zoonotic disease, the outbreak of disease is always recognized before the disease is known. In these cases, many of the most sensitive detection techniques, which require an identification of the disease agent, cannot be employed effectively. In this case, environmental sampling may not be useful until the disease can be specified, although in some cases, metagenomic studies of the environment could potentially help illuminate the cause of an unknown illness.
If it is known, what is known about its transmission? This is critical to understanding how best to sample, and what type of sample may be most appropriate (inner ring of ). For example, if the disease is generally passed through the fecal-oral route, where transmission may occur during contact with contaminated fomites, the primary hazards may be on surfaces, rather than in air samples. However, it is important not to exclude potential transmission routes, since diseases may be transmitted through multiple pathways, and circumstances, such as aerosol-generating medical procedures, may influence the outcome.
Implications
While the application of environmental sampling to each situation has unique aspects, the tools developed for each purpose are similar (). The remainder of this review examines current practices and recent advances in technology that may be used for environmental sampling of infectious disease, notes limitations of those technologies, recommends best practices, and identifies directions for future research.
Real time monitoring for infectious disease aerosol
Current technology for the detection, and possible identification, of biological aerosols is near instantaneous (seconds to minutes), classification of aerosols or aerosol mass can be broken into three categories: optical spectroscopy, mass spectrometry and atomic spectroscopy. This section focuses on the application of these techniques to monitoring for infectious disease. summarizes key points.
Table 1. Real-time bioaerosol measurement technologies, their operating principals, advantage and limitations.
Optical spectroscopy
A widely used technique for real-time biological aerosol measurement is ultraviolet laser (or light) induced fluorescence (UV-LIF). UV-LIF is the fundamental principal in many sensors developed for early warning of possible presence of bio threat agents (Cabalo et al. Citation2005; Damit and Antoine Citation2021; DeFreez et al. Citation2005; Hairston, Jim, and Quant Citation1997; Kaye et al. Citation2005; Pan et al. Citation1999; Sivaprakasam et al. Citation2004), and ambient characterization of bioaerosols (Healy et al. Citation2014; Huffman et al. Citation2012; Huffman, Treutlein, and Pöschl Citation2010; Pan et al. Citation2003, Citation2007; Pinnick et al. Citation2004; Santander et al. Citation2021; Santarpia et al. Citation2013; Savage et al. Citation2017; Toprak and Schnaiter Citation2013). These instruments have been used to characterize biological aerosols in indoor environments (Addor et al. Citation2022; Bhangar, Huffman, and Nazaroff Citation2014; Li et al. Citation2022; Su, Lau, and Fang Citation2017; Tanaka Citation2021), to investigate emissions from human subjects (Bhangar et al. Citation2016; Nathu et al. Citation2022; Yang et al. Citation2021), and to characterize risk in places such as hospitals using tracer materials (Therkorn et al. Citation2019), and aircraft cabins (Kinahan et al. Citation2021).
UV-LIF devices illuminate aerosol particles (often individual particles) with ultraviolet light. UV sources range from light emitting diodes (UV-LEDs), lasers, and xenon lamps with wavelengths from 260 nm to 405 nm. This illumination excites biological molecules in particles that range from aromatic amino acids (e.g. tryptophan, tyrosine and phenylalanine) at short wavelengths (260–280 nm), nicotinamide adenine dinucleotide (NADH and NAD+) at mid wavelengths (350–370 nm), and flavin molecules (e.g., riboflavin) at 405 nm. The excited molecules then relax to a lower energy level and emit light at longer wavelengths, often in the visible spectrum. Emitted light can be characterized by wavelength and intensity to determine consistency with a biological particle. Most instruments also quantify aerodynamic or optical sizes of individual particles using a separate optical arrangement. A recent review (Huffman et al. Citation2020) examines UV-LIF technologies in greater detail.
The Ultraviolet Aerodynamic Particle Sizer (UV-APS) (Hairston, Jim, and Quant Citation1997) and a related instrument, designed for military use, the Fluorescent Aerodynamic Particle Sizer (FLAPS) (Ho Citation2002; Ho, Spence, and Hairston Citation1999) were used to characterize biological aerosols (as described above). Both instruments were discontinued (in 2008 (FLAPS) and 2014 (UV-APS)), but the data they acquired merits discussion of their operation. The UV-APS and FLAPS illuminate single particles with a 355 nm laser beam, monitoring light scattering, fluorescence, and time of flight for aerodynamic aerosol diameter similar to the Aerodynamic Particle Sizer (APS Model 3321; TSI, Inc. St. Paul, MN). The Wideband Integrated Bioaerosol Sensor (WIBS; Droplet Measurement Technologies, Boulder, CO) (Kaye et al. Citation2005) produces real-time, size-resolved, bioaerosol measurements comparable to the UV-APS. The WIBS has the advantage of multiple wavelength excitation (280 and 370 nm) which provides a wider range of fluorophores (aromatic aminos and NADH) than achieved by the UV-APS. Rather than a laser excitation at fluorescent wavelengths, the WIBS uses two xenon lamps filtered for each excitation wavelength. This has the advantage of lower costs than laser sources, but it may limit sensitivity and dynamic range of fluorescence intensity. Aerosols are sized via optical scattering, rather than aerodynamic time of flight. Other commercial bioaerosol detection instruments, such as the Instantaneous Biological Analyzer and Collector (IBAC; Teledyne FLIR, Wilsonville, OR) or the TacBio (Research International, Monroe, Washington), are marketed as detectors rather than scientific instruments. These use UV-LEDs for the excitation source (405 nm for IBAC, 275 nm for TacBio). These instruments can be used to monitor for bioaerosols, but the data available is limited to approximate classifications as biological or non-biological, based on a predetermined algorithm, and particle size.
Beyond commercial technologies, several groups have developed UV-LIF aerosol measurement tools that provide detailed UV-LIF measurements yielding significant insights into the fluorescence properties of biological aerosol particles. These include the single particle fluorescence spectrometer (SPFS; (Pinnick et al. Citation1998)), and the single-particle fluorescence analyzer (SPFA; (Eversole et al. Citation1999)). Both systems have undergone multiple laboratory iterations allowing for measurements at multiple excitation and emissions ranges (Pan et al. Citation1999; Pan, Hill et al. Citation2014; Sivaprakasam et al. Citation2011). Increased understanding of excitation and emission patterns from these instruments have advanced specificity for identifying biological agents of concern (Eversole et al. Citation1999; Pan et al. Citation2007; Pan, Hill et al. Citation2014; Pinnick et al. Citation2004) and how the spectral properties of these particles change over time (Kinahan et al. Citation2019; Pan et al. Citation2021; Pan et al. Citation2011; Pan, Santarpia et al. Citation2014; Ratnesar-Shumate et al. Citation2015; Santarpia et al. Citation2012; Santarpia, Collins et al. Citation2022).
Raman spectroscopy has also been used to classify biological aerosols (Doughty and Hill Citation2017; Kalume et al. Citation2018, Citation2018; Sengupta et al. Citation2005; Tripathi et al. Citation2009; Wang et al. Citation2015). Raman spectroscopy involves the absorption of incident radiation by chemical bonds, which causes vibrations in the bonds resulting in a wavelength shift when it is re-emitted. In this way, Raman spectroscopy can provide information about the types of bonds in a sample, thereby offering information about bioaerosol composition that complements fluorescence (Ai et al. Citation2022; Sengupta et al. Citation2005). However, the Raman signal is weak. (One in every 107 photons emitted in a scattering event is Raman shifted.) This means that collecting enough Raman scattered light to develop a spectrum from a single aerosol particle requires both an intense light source, such as laser light, and several seconds to minutes of illumination and sensing. This limits the potential for real-time analysis. One approach has been to collect aerosol on a surface over short periods with subsequent Raman analysis of the collected material (Doughty and Hill Citation2017; Sengupta et al. Citation2005; Tripathi et al. Citation2009). The downside of Raman analysis is interferences from non-biological materials that can obscure the Raman bioaerosol spectra. Although not immediately applicable to real-time analysis, trapping of single aerosol particles allows Raman analysis of single particles (Wang et al. Citation2015).
Elastic light scattering (ELS) has also been used to classify biological aerosols. Pan, Aptowicz et al. (Citation2022) reviews these techniques. Mie scattering (a form of ELS) from a particle is related to its size and is used in optical particle counters (OPC) (Hinds et al. Citation1982). Compared to aerodynamic sizers (Hinds Citation1982), OPCs are less complex, more cost-effective, and in more common use, although they require assumptions concerning particle refraction indices, shapes, and densities. Particle shape, symmetry, and surface roughness, as determined by measurements of scattering phase functions (Auger et al. Citation2007; Fu et al. Citation2017; Kaye et al. Citation2000; Li, Kattawar, and Yang Citation2004), can also help to understand bioaerosol properties, particularly when coupled with fluorescence measurements (Kaye et al. Citation2000). Elastic scattering can be used with inelastic scattering (e.g., fluorescence and Raman scattering) to provide additional information about particles that can aid in their classification. Another approach for bioaerosol classification relies on Circular Intensity Differential Scattering (CIDS) that uses circularly polarized light to measure asymmetric properties of the molecules in a sample. CIDS has been used to examine DNA orientation in sperm cells (Shapiro et al. Citation1994) and biological particles in a flow cytometer (Sloot et al. Citation1989). More recently, CIDS has been developed into a simplified system capable of real-time bioaerosol classification (Pan, Kalume et al. Citation2022).
These examples of optical bioaerosol measurements pertain to in-situ measurements. Several of these techniques can be used in “stand-off” detection, which refers to measurements made at some distance from the source. Light detection and ranging (LIDAR) has also been applied to stand-off bioaerosol detection, although it also measures non-biological aerosols. Linear depolarization (Mayor, Spuler, and Morley Citation2005; Roy Citation2010) and UV-LIF (Christesen et al. Citation1994; Richardson et al. Citation2019; Shoshanim and Baratz Citation2020) have been used to detect bioaerosol plumes. LIDAR has the distinct advantage of taking measurements over a large area and at a distance.
Mass spectrometry
Mass spectrometry (MS) can quantify many organic compounds, including those in biological particles (Fergenson et al. Citation2004; Kleefsman et al. Citation2008; van Wuijckhuijse et al. Citation2005). This section focuses on MS techniques that collect mass spectra of single aerosol particles (Fergenson et al. Citation2004; Kleefsman et al. Citation2008). MS ionizes molecules which are then passed through electric or magnetic fields that separate them by their mass to charge (m/z) ratios prior to detection. Pyrolysis, heating of the particle, was originally used as the method of ionization (Sinha et al. Citation1984). Laser desorption/ablation (Fergenson et al. Citation2004; Gard et al. Citation1997; Tobias et al. Citation2005) and matrix assisted laser desorption ionization (MALDI; (Bonk and Humeny Citation2001; Kleefsman et al. Citation2008; van Wuijckhuijse et al. Citation2005)) are more recent methods.
These techniques produce fragments with detected patterns that are related to the original molecules. Laser desorption instruments produce fragments with m/z ratios up to a few 100 (Gard et al. Citation1997; Kleefsman et al. Citation2007), while MALDI produces larger fragments up to 20,000 m/z (van Wuijckhuijse et al. Citation2005). The large m/z in MALDI-based systems allows for detecting large peptide fragments and some small proteins (Kleefsman et al. Citation2008; van Wuijckhuijse et al. Citation2005). The difficulty in MALDI MS of single particles is that matrix material must be added to the particles in flight. However, this difficulty has been overcome, so long as the matrix can be volatilized and condensed onto the particle surface (van Wuijckhuijse et al. Citation2005). The small fragments of laser desorption ionization could represent whole amino acids, particularly when in the presence of other absorbing material (Russell et al. Citation2004).
Due to the intrinsic complexity of bioaerosols, the resulting spectra can be complex, and useful signature libraries must be developed. Despite the difficulties, the ability of MS to identify and characterize bioaerosols has become quite robust. The Bioaerosol Mass Spectrometer (BAMS) has been used to detect tuberculosis in the exhaled breath of patients and single particle MALDI techniques have the potential to bring the clinical specificity of MALDI-Time of Flight (TOF)-MS to aerosol measurements (Marvin, Roberts, and Fay Citation2003). Mass spectral signatures contain more information than the optical techniques, although they are complex to interpret. Some combined systems use fluorescence detection to preselect biological particles for MS analysis (Stowers et al. Citation2006). This preselection can reduce the analysis burden and increase the likelihood of detecting bioaerosols of concern.
Atomic spectroscopy
Laser induced breakdown spectroscopy (LIBS) (Hybl, Lithgow, and Buckley Citation2003) or spark induced breakdown spectroscopy (SIBS) (Bauer and Sonnenfroh Citation2009) can be applied to a bioaerosol to create a plasma. Excited atoms and ions in the plasma emit light, which can be used to identify the elemental composition of the target particle. These characteristic emissions have been used to discriminate biological from non-biological particles based on elemental composition (Bauer and Sonnenfroh Citation2009; Boyain-Goitia et al. Citation2003; Schmidt and Ray Bauer Citation2010). Similar to MS, the spectra are used to develop signatures, which have some ability to classify and identify bacteria (Rehse Citation2019).
Limitations of real-time measurements
Despite the advantages and utility of real-time biological aerosol analysis, it has several significant drawbacks when applied to disease surveillance. No current real-time detection technology can definitively determine the species in a biological aerosol. Fluorescence, elastic scattering, Raman and atomic spectroscopy can identify signatures that are consistent with life (amino acids, nucleic acids) but they do not discriminate further. The closest exception to this limitation is bioaerosol MS, for which some degree of speciation has been shown with both the BAMS, also referred to more generally as the Single Particles Aerosol Mass Spectrometer (SPAMS) (Fergenson et al. Citation2004; Tobias et al. Citation2005) and MALDI-ToF-MS (Marvin, Roberts, and Fay Citation2003; van Wuijckhuijse et al. Citation2005). However, these techniques are not in broad use, due to their high cost and complexity.
Many of the biological signatures used by these techniques are not stable in the particulate phase, due to chemical changes that may occur from exposure to ambient conditions. The stability of UV-LIF signatures has been studied extensively (Kinahan et al. Citation2019; Pan, Santarpia, Collins et al. Citation2014; Pan et al. Citation2021; Ratnesar-Shumate et al. Citation2015; Santarpia et al. Citation2022). Although atomic emission and MS spectra are not impacted in the same way as UV-LIF, under atmospheric conditions outside the laboratory, the signatures used by these techniques to identify biological aerosols may still be affected.
Background materials may also interfere with classification of bioaerosols. Particles of biological origin (organic carbon, unburnt fossil fuels, plant material) may have signatures that can be mistaken for potentially infectious biological material (Savage et al. Citation2017). Further, fluctuations in the non-biological background aerosol may confuse detection algorithms used by some instruments (Ratnesar-Shumate et al. Citation2011). As yet, no current real-time measurement technology can determine the viability of the measured aerosol. The signatures used for detection of these biological aerosol are not necessarily directly related to their viability.
Recent advances in the collection of infectious aerosol
Aerosol collection for biological analysis has been the subject of textbooks (Hinds Citation1982), and recent review articles (Mainelis Citation2020), so only a brief overview of the techniques is presented here, focusing on recent advances in aerosol collection techniques for the characterization of disease-causing biological agents, particularly during the COVID-19 pandemic. Suspended particles can be collected by filtration, impaction, impingement, cyclonic collection and electrostatic deposition. Some technologies employ more than one of these methods to improve collection efficiency, viability of collected material, and to simplify or automate downstream analysis.
Impingement
Impingers designed specifically for bioaerosol sampling, including various models of the SKC Biosampler (SKC, Inc., Eighty-four, PA, USA) and the All Glass Impinger (AGI; Ace Glass, Vineland, NJ, USA) are often used in bioaerosol collection to preserve viability. In these devices, sampled air is directed onto the surface of a liquid that can be composed of water, buffers, or growth media. This collection method is considered more gentle than techniques like impaction or filtration, since the particles are collected into a liquid, rather than onto a solid surface.
Filtration
Filtration involves collection of particles onto a porous membrane or a fiber-based filter, both of which can be made of varied materials (Chow et al. Citation2022). Filtration mechanisms are described in detail in Hinds (Hinds Citation1982), but it is worth noting that several mechanisms are responsible for the removal of particles during filtration, including gravitational settling, impaction, interception, and diffusion. Filters can also be charged to encourage electrostatic collection. Several studies have examined filter types for bioaerosol sampling with positive results, compared to impingers, even for fragile organisms (Burton, Grinshpun, and Reponen Citation2006; Dybwad, Skogan, and Martha Blatny Citation2014; Ratnesar-Shumate et al. Citation2021). Dybwad, Skogan, and Martha Blatny (Citation2014) compared 8 different samplers to a reference SKC Biosampler (impinger), including a gelatin filter. The gelatin filter yielded results comparable to those of the reference SKC Biosampler at preserving viability of most organisms, except Serratia marcescens, where viability was compromised. Ratnesar-Shumate et al. demonstrated that for laboratory-generated SARS-CoV-2, filter based collection (Polytetrafluoroethylene (PTFE) and gelatin membrane) was equal to PTFE filters and or nearly equal to gelatin filters, and better than other impingers (Ratnesar-Shumate et al. Citation2021). Gelatin membrane filters, which are designed to have high moisture content and to be water soluble to optimize the collection of viable bacteria, were used throughout the COVID-19 pandemic to collect, and in some cases cultivate, SARS-CoV-2 aerosols from various environments (Barksdale et al. Citation2021; Mouchtouri et al. Citation2020; Ong et al. Citation2020; Rodríguez et al. Citation2021).
Impaction
Impaction itself is also used to collect particles by accelerating them through a nozzle into a jet that is directed toward a collection surface. This surface can be a flat plate, a filter, or a culture medium. As the aerosol-laden jet approaches the collection surface the air streamlines turn to avoid the obstacle and the more massive particles do not follow streamlines and impact on the collection surface. This process can be repeated with a series of stages with smaller nozzles and faster jets removing smaller particles through the impactor stack. The final stage of most impactors contains filter to collect particles smaller than the final stage. Impactor stages are classified by a “cutoff aerodynamic diameter”, which is the size where 50% of the particles are collected. Several impactor technologies have been recently employed for bioaerosol sampling that have either been repurposed for bioaerosol sampling or have been recently developed specifically to improve the collection of bioaerosols. The Sioutas Impactor (SKC, Inc., Eighty-four, PA, USA) (Misra et al. Citation2002; Singh, Misra, and Sioutas Citation2003) consists of 5-stages with cutoff diameters of 2.5, 1.0, 0.5 and 0.25 µm when operated at the recommended 9 Lpm flow rate. The 5th stage consists of a filter that captures particles smaller than 0.25 µm. The sampler is small (8.6 × 5.6 cm) and can be operated by a small pump, allowing it to be worn for personal sampling. The small size and small pump requirements allow it to be used in a variety of situations where larger impactors may be difficult to employ. Despite not being originally designed for bioaerosol sampling, it has been used for that purpose (Nannu Shankar et al. Citation2022; Liu et al. Citation2020), with one study reporting the cultivation of SARS-CoV-2 from a collected sample (Lednicky et al. Citation2021a).
The Next Generation Impactor or Next Generation Pharmaceutical Impactor (NGI; TSI, Inc, St. Paul, MN, USA) (Marple et al. Citation2003) is a versatile, 8-stage unit that can be operated at several flow rates (15, 30, 60 and 100 Lpm), with cut-point sizes from 14.10 µm to 0.24 µmhttps://tsi.com/products/inhaler-testing-automation-and-lab-organization/ngi-and-ngi-plus/). Although originally designed for pharmaceutical applications, where it is the current industry standard for the evaluation of aerosol delivered therapeutics, it has been repurposed for bioaerosol sampling (Harris et al. Citation2022; Leung et al. Citation2005), where infectious virus recoveries have been demonstrated (Leung et al. Citation2005). Although there are limited reported uses of this system for bioaerosol studies, the versatility in terms of size range and flow rate offers advantages, depending upon the application.
The BioSpot samplers (Aerosol Devices, Ft. Collins, CO, USA) is a novel impactor technology that was developed to facilitate the collection of viable bioaerosols over a broad size range (Lednicky et al. Citation2016; Pan et al. Citation2016, Citation2018; Walls et al. Citation2016). This sampler condenses water on particle surfaces when they pass through the initial portion of the sampler. Small particles grow due to condensation making them easier to collect. Larger particles do not grow as much as small ones. The surface water layer reduces the stress of bioaerosol collection and preserves particle viability. Since particles are grown through condensation, size segregation isn’t possible, but the water condensation allows efficient collection of particles from 0.05 to 10 µm. Collection of viable viruses, bacteria and yeast has been validated by several studies (Pan et al. Citation2017, Citation2018; Walls et al. Citation2016). The sampler was used effectively during the COVID-19 pandemic to demonstrate the viability of virus exhaled by hospitalized patients (Lednicky et al. Citation2020).
Cyclonic collection
Cyclones impart a circular motion to the airstream, with centripetal forces moving heavier particles out of the outlet stream to be collected on the cyclone walls or in a hopper. Like impactors, a particle’s inability to follow the streamlines results in this removal. The cyclone walls and hopper contents are washed to recover the larger particles while the smaller particles are collected on a filter placed at the exit duct. A wetted wall cyclone uses a stream of liquid that circulates on the interior surface of the cyclone to collect particles. The NIOSH BC251 (Tisch Environmental, Cleaves, OH, USA; (Lindsley, Schmechel, and Chen Citation2006)) creates cyclonic flow using centrifuge tubes and collects particles in three size ranges: >4.1 µm, 1–4 µm, and<1 µm (on a filter stage). This sampler has been used : i) to study influenza viruses (Blachere et al. Citation2009; Lindsley et al. Citation2010), in the laboratory (Blachere et al. Citation2011), ii) to collect norovirus and respiratory syncytial virus (RSV) during outbreaks in healthcare facilities (Bonifait et al. Citation2015; Grayson et al. Citation2017), iii) to study potential infectious aerosols in agricultural (Blais Lecours et al. Citation2012; Martin et al. Citation2015) and animal care facilities (Blachere et al. Citation2018; O’Brien and Nonnenmann Citation2016), and iv) to characterize patient-generated SARS-CoV-2 aerosol during the COVID-19 pandemic (Chia et al. Citation2020) identify the size range of infectious SARS-CoV-2 produced by hospitalized patients (Santarpia, Herrera et al. Citation2022).
Electrostatic collection
Electrostatic precipitation creates an electric potential between two collection surfaces by applying high voltage to one and grounding the other. Charged particles are attracted to the oppositely charged surface for collection. Electrostatic collection does not require focusing particles using a high velocity nozzle and the lower pressure drops permit higher flow rates and less noise to achieve sample sizes adequate for subsequent analyses. Initial investigation into the use of ESPs for bioaerosol collection began over 20 years ago (Mainelis Citation1999). In the years since, several bioaerosol ESP systems were designed and evaluated (Gordon et al. Citation2015; Han et al. Citation2022; Han, Thomas, and Mainelis Citation2017, Citation2018; Mainelis et al. Citation2002; Priyamvada et al. Citation2021; Therkorn et al. Citation2017). The simplicity of ESP design lends itself to a variety of applications where other aerosol sampling devices may not be practical. ESP devices can be designed to passively sample (Gordon et al. Citation2015; Manibusan and Mainelis Citation2022; Therkorn et al. Citation2017), requiring no pump or blower, which eliminates the noise generated by most aerosol sampling devices. Particles can be charged using active techniques, such as corona discharge, or simply acquire charge as they pass through the electric field between the plates. ESPs can be used as stationary samplers (Gordon et al. Citation2015; Han et al. Citation2022; Priyamvada et al. Citation2021), or as personal samplers (Han, Thomas, and Mainelis Citation2017, Citation2018). During the COVID-19 pandemic they were deployed in public schools to detect the presence of SARS-CoV-2 aerosol (Crowe et al. Citation2021), where quiet operation was critical to the environment.
Considerations
The ideal sampling method depends on the desired outcomes (e.g., determining the presence of an infectious agent, determining the size range, determining infectiousness/viability, etc.) and technology limitations. If understanding bioaerosol concentrations as a function of size is a goal, a multistage cyclone or impactor can provide size resolution. If maintaining viability of a fragile organism is desired, more gentle collection techniques used, such as impingement, wetted wall cyclones, gelatin filters or condensation-based collectors, should be used. It is also important to understand the limitations of each technology. For instance, gelatin filters may become brittle and crack as they desiccate over long sampling periods, and they can partially dissolve at high relative humidity. Both conditions render the substrate useless for bioaerosol collection. Liquids may evaporate from an impinger and when the liquid contains a buffer or media, those components might concentrate and an inhospitable environment for some organisms.
Surface sampling
The sampling of inanimate surfaces for evidence of infectious material is a subject that has been addressed by several U.S. government agencies (Sehulster et al. Citation2004; Silvestri et al. Citation2021) and the (World Health Organization (WHO), Citation2020). Otter et al. highlights the role that contaminated surfaces play in hospital-related infections (Otter et al. Citation2013). Surface sampling refers to the active removal of existing contamination from surfaces by a variety of methods including wiping with remoistened swabs, sponge sticks, or gauze pads. This contrasts with sampling using deposition plates that quantify the gravitational settling of particles onto controlled surfaces for subsequent analysis. Surfaces contaminated with settled particles, contact with contaminated hands or gloves, and deposited or spilt fluids are considered together as surface contamination. Both the U.S. Environmental Protection Agency (EPA) and the U.S. Centers for Disease Control (CDC) publish guidance for surface pathogen measurement (Silvestri et al. Citation2021). Guidance issued by the CDC is applies to healthcare facilities for outbreak investigations, disease transmission research, environmental hazard evaluations, remediation effectiveness, and quality assurance (Sehulster et al. Citation2004). CDC guidance is not as detailed as the EPA guidance as it focuses more specifically on hospital environments.
EPA guidance (Silvestri et al. Citation2021) provides advice on the size of sample desired (swabs for sample areas up to 26 cm2, sponge sticks for sample areas up to 645 cm2, and gauze wipes for sample areas as large as 929 cm2). Various recovery media are considered (e.g., viral transport media, PBS, cell culture media). Various combinations of collection methods and recovery media, as well as the sampled surface affect how much virus is collected (Julian et al. Citation2011). Several studies have examined the impact of sampling technique, eluant, and surface on the virus recovery. Taku et al. describes three different mediums on 4 different surfaces and a variety of collection techniques relevant to feline calicivirus (FCV) in food preparation areas (FCV is a surrogate for human norovirus) (Taku et al. Citation2002). Cantu et al compared surface sample differences using two transport mediums (VTM and SDS) and two RT-qPCR analysis methods (Thermo and PerkinElmer), finding that the eluant selection made a difference in the detection sensitivity (Cantú et al. Citation2022). Park et al. compared samples taken with fiber-tipped swabs, antistatic wipes, and macrofoam swabs for norovirus, finding that macrofoam swabs had the overall best performance (Park, Chhabra, and Vinjé Citation2017). Scherer et al. collected norovirus and rotavirus from stainless steel, ceramic plate, polyethylene, and wood) surfaces, finding that the best recovery was from ceramics, followed by stainless steel and wood (Scherer et al. Citation2009). Herzog et al. tested acrylic, galvanized steel, and laminate for bacteriophage P22 recovery, which was most efficiently collected from laminate surfaces (Herzog et al. Citation2012). Downey et al. investigated relationships among of bacterial species (Escherichia coli, Bacillus cereus, Burkholderia thailandensis), eluant media (PBS and Butterfield’s buffer with and without Tween 80), and dissociation method (vortexing and sonication) on recovery efficiency, finding difference among the species and that the addition of surfactant (Tween 80) resulted in the highest recovery efficiency for each organism (Downey et al. Citation2012). Eluant media was also found to affect viral recovery for Hepatitis C (Carducci et al. Citation2001). According to EPA guidance documents, the sample type and technique should be based largely on the size of the sample area, while the choice of eluant or recovery media is specific to the organism being sampled and the downstream analysis techniques.
Surface sampling was used to investigate outbreaks of gastroenteritis due to human norovirus associated with catering companies (Boxman et al. Citation2011) potential ASFV contamination in swine feed production (Gebhardt et al. Citation2021). The potential for animal to human transmission of Influenza A from swine in confinement has also been investigated (Prost et al. Citation2019). Surface sampling played an important role in understanding the transmission of SARS-CoV-2 (Chia et al. Citation2020; Gonçalves et al. Citation2021; Mouchtouri et al. Citation2020; Pochtovyi et al. Citation2021; Santarpia et al. Citation2020).
Wastewater sampling
Wastewater sampling for infectious disease has been important to the efforts to eradicate polio (Hovi et al. Citation2012) and identifying the reemergence of polio in the U.S. (Russo et al. Citation2022). It has also been used throughout the SARS-CoV-2 pandemic to examine circulation in communities (Wu et al. Citation2021) and help identify emerging outbreaks (O’Reilly et al. Citation2020). In addition, wastewater has been used to create seasonal profiles of noroviruses, enteroviruses and, and adenoviruses (Katayama et al. Citation2007) in Japan. Norovirus, astrovirus, rotavirus, adenovirus, Aichi virus, parechovirus, hepatitis A virus, and hepatitis E virus have been profiled in wastewater from Sweden (Hellmér et al. Citation2014) prior to human outbreaks. A variety of bacteria pathogens, including Bacillus cereus, Clostridium perfringens, Escherichia coli O157:H7, Helicobacter pylori, Klebsiella pneumoniae, Legionella pneumophila, Listeria monocytogenes, Pseudomonas aeruginosa, Salmonella sp., and Staphylococcus aureus, can also be identified by qPCR of wastewater samples (Shannon et al. Citation2007; Ugarte-Ruiz et al. Citation2015). The effectiveness of this approach has led to national wastewater surveillance system for COVID-19 and its expansion to other disease is under consideration (Based Disease Surveillance for Public Health Action Citation2023).
In continuous flow-proportional sampling, a small amount of fluid is collected for specified volumes of liquid flowing past the collector. This method requires continuous flow measurement of the waste stream and can be costly to implement. Twenty-four hour composite samples are similar to continuous flow sampling, except that samples are collected at specified time intervals rather than based on flow volume. Both of these methods represent composites of the waste stream over a period of time and therefore temporal variations in the waste stream are not observable. Other sampling methods offer more cost effective solutions. In grab sampling a onetime sample is taken to represent a specified time period. This method can be influenced by temporal variations in the waste stream. However, it has been shown to be effective for SARS-CoV-2 monitoring if the sampling time corresponds with presence of the suspected contaminant (Augusto et al. Citation2022; Kmush et al. Citation2022). Passive sampling (Bivins et al. Citation2022; Rafiee et al. Citation2021) involves the collection of the analyte of interest on a collection medium that is submerged in the waste stream to accumulate time-integrated samples.
Sample concentration is often necessary as the contaminant concentrations are low, particularly with non-passive sampling. LaTurner et al. compared several concentration methods (Direct extraction, Filtration with bead beating, Filtration with elution, polyethylene glycol (PEG) precipitation, Ultrafiltration) in the collection of SARS-CoV-2 contaminated wastewater (LaTurner et al. Citation2021).
Analysis of samples for pathogens
When looking for pathogens in an environmental sample, there are two dominant questions: 1) What is the pathogen? and 2) Is it infectious?
In some cases, it is enough to know that the pathogen is present in the sample for it to be of concern. In other cases, the pathogen must be both identified and confirmed to be capable of causing disease (i.e., viable or infectious). Identification of either the nucleic acid or protein signature is used to identify the pathogen, and culture methods are used to determine viability.
Biological analysis techniques
Several techniques to determine the presence of an infectious agent are summarized in . Confident identification of an agent can be made by: i) polymerase chain reaction (PCR) or loop-mediated isothermal amplification (LAMP) (Notomi et al. Citation2000), where a unique fragment of the organism’s DNA or RNA is recognized by short RNA or DNA strands (Fredricks and Relman Citation1999); ii) immunoassay, where antigenic proteins of the organism are recognized by antibodies (Andreotti et al. Citation2003); iii) sequencing, where all or large fragments of the organism’s genome are read and recognized (Duan et al. Citation2021); and iv) clustered regularly interspaced short palindromic repeats (CRISPR) based assays where guide-RNAs recognize specific segments of the organisms genome and an engineered enzyme reports binding of the guides (Yin et al. Citation2021). Beyond the advancements of isothermal nucleic acid amplification techniques, like LAMP, and the modification of Cas enzymes enabling CRISPR techniques to be used for detection purposes, there have been interesting advances in PCR techniques, as well. One potentially important advance is in digital droplet PCR (ddPCR) (Hindson et al. Citation2011). In ddPCR, the PCR reaction is done in an emulsion to create tens of the thousands of individual nanoliter PCR reactions. Shrinking the individual reaction volumes to the point where single copies of the target sequence are in each droplet enables quantification of low-abundance nucleic acids. This technique has been applied to environmental samples with some success (Kokkoris et al. Citation2021).
Table 2. Overview of high-level analysis techniques for biological sampling identification. Sample recovery refers to the need to extract the sample from the collection substrate. *In some cases, bacteria and fungi can be collected onto growth media and cultivated from the collection surface. Analyte extraction refers to extracting the molecular analyte (typically nucleic acid or protein) from the recovered sample. **In some cases, proteins and nucleic acids can be detected from the collected sample without extraction or purification, particularly if surface proteins are the target or if extracellular DNA/RNA is present. Analyte conversion refers, in this case to the conversion of RNA to DNA for use in DNA amplification technologies. Analyte amplification refers to the amplification of the analyte to improve sensitivity. This applies most directly to sequencing application where a segment of DNA must be read multiple times (termed coverage) to ensure the sequence is accurate and the detection is confident. ***PCR and LAMP both implicitly amplify the analyte as a part of the detection process and can be used to amplify target DNA for downstream sequencing. Sensitivity refers to the fundamental sensitivity of the technique. Most nucleic acid detection techniques advertise single copy sensitivity, meaning that the technique can identify a single copy of the target sequence, but that does not account for instrument signal to noise, chemical inhibition or other factors that may decrease sensitivity.
Once an agent has been identified in a sample, the next step should be an attempt to cultivate it to understand the potential risk. The determination of culturability of both influenza (Milton et al. Citation2013) and SARS-CoV-2 (Lednicky et al. Citation2020; Santarpia, Herrera et al. Citation2022) from patient-generated aerosol has helped to settle the issue of transmission from fine particles, despite the detection of viral RNA from both viruses in prior studies (Blachere et al. Citation2009; Lindsley et al. Citation2010; Liu et al. Citation2020; Santarpia et al. Citation2020).
Depending on the type of pathogen (e.g., bacterial or viral) different culture techniques are used. For bacteria, samples can be cultured on an agar media preferred by the pathogen. This can be difficult for bacteria, unless selective media for the pathogen of interest is available, since many environmental bacteria and molds will grow on broad spectrum agar plates. Despite routine contamination that can occur in environmental samples, it has been estimated that more than 99% of environmental bacteria cannot be cultured in the laboratory (Sharma et al. Citation2005). There are better techniques for eliminating unwanted bacteria and molds from viral cultures. Since most viruses cannot be filtered, sterile filtration with a 0.22 µm filter can remove contaminants without reducing viral concentration. Antibiotics and anti-fungal agents may also be added to cultures to prevent growth. Culture of virus is more complicated than most bacterial cultures, since the virus requires a host, not only nutrients, for growth. Assuming that an appropriate host cell line is known, those host cells must be cultivated and prepared for viral infection. During initial isolation it is more common to introduce samples to a monolayer of cells in media and look for evidence of cell death or cytopathic effect (CPE), rather than attempting to titrate the sample directly using a plaque assay or other assay.
The impact of sample preparation on analysis sensitivity
The desired analyte extracted from the collection medium must be in sufficient quantities to be detectable by the analysis method. Each step of the process has an associated efficiency, and each analysis process has an associated sensitivity. The sensitivity depends on the sensitivity of the analytical technique and the associated efficiencies for each step of the process. This can be represented by the following expression:
where SP and SA are the sensitivities of the entire process and the analytical technique, respectively. ES, ER and EE are the efficiencies of sampling, recovery, and analyte extraction, respectively. The sample volume is VR, and the input and output volumes for analyte extraction are VEI and VEO, respectively. For qPCR, analysis, consider the efficiency of the surface sampling to be 20%, the recovery efficiency of 50% in 10 mL of buffer, and the DNA extraction efficiency is 90% with an input volume of 0.5 mL and an output volume of 0.1 mL. If the PCR assay has a sensitivity of 1 copy per reaction with a reaction volume of 5 µL, then~1.1×105 copies of the target DNA would be needed to meet the sensitivity limits of the analysis. Ideally, this process would be optimized by maximizing the efficiency at each step, minimizing the recovery volume, and maximizing the concentration achieved in analyte extraction (if possible).
Recommendation for best practices
The framework provides a basis to examine the application of environmental sampling technologies with respect to the previously identified primary categories: outbreak investigation, risk assessment, disease surveillance, biodefense, and environmental characterization. Examples are described below.
Outbreak investigations
Outbreak investigations and risk assessments are often intertwined. The primary goal of an outbreak investigation is to determine the source of an outbreak, which may also implicate the dominant mode of transmission and identify risks. In the human case of avian influenza (H7N2), a veterinarian came down with a flu-like illness after caring for a group of sick cats in a shelter (Marinova-Petkova et al. Citation2017). The disease was identified as H7N2 and had already spread to multiple shelters. To limit further transmission the affected cats were moved to a quarantine facility. An environmental sampling study was performed both to confirm the source of the outbreak and to assess the risk in caring for the affected animals, since the dominant modes of transmission were uncertain. Size resolved aerosol samples and surface samples were analyzed using RT-PCR and through culture. High percentages of the aerosol and surface samples were positive for H7N2 RNA, and infectious virus was identified in many samples of both. This confirmed the source of the human disease (infected cats) and identified transmission risk from both contaminated fomites and aerosol, thus indicating the risk to workers and explaining the rapid spread of the disease.
Risk assessments
Sampling around hospitalized patients is often done to understand modes of disease transmission and risks that might be posed to staff and other patients during care. Hospitals provide natural environments to conduct these studies, since individuals infected with most pathogens of concern will require intense medical care. Especially if the pathogen is newly emergent or poorly studied, an integrated approach to these initial sampling efforts should be undertaken. The COVID-19 pandemic brought to light misconceptions around the transmission of infectious disease by the airborne route (Morawska and Milton Citation2020). Norovirus has been found in aerosols and has been demonstrated to replicate in salivary glands (Bonifait et al. Citation2015; Ghosh et al. Citation2022), indicating that despite the belief that fecal-oral is the only means of transmission, airborne droplets produced in the oral cavity may also have a role in transmission, and the observed surface contamination may also be from those droplets (Alsved et al. Citation2020). Aerosol producing medical procedures, necessary for the care of critically ill patients, may also produce hazards from bloodborne disease that would warrant enhanced infection control procedures. Even if the disease is thought to have a bloodborne or fecal-oral transmission route, environmental samples, including air and surface should be taken around the patient.
The ideal sampling plan would begin as early as possible after the arrival of a patient and continue at regular intervals over the course of hospitalization. Infectiousness changes over the course of infection, and there is often little to no direct evidence to determine how long a person might be infectious. Data from the exhaled breath of patients with SARS-CoV-2 suggests that infectiousness is highly variable (Ma et al. Citation2021) from person to person. Given that infectiousness changes from person to person and over the course of infection, environmental monitoring should be done frequently to better understand the hazards. The methodologies most applicable to sampling around hospitalized patients are surface and aerosol sampling. Real-time detection technologies may be included, but alone they do not offer enough specificity to be useful in this environment. Sampling of the waste stream is important and can be done in the restroom, rather than the wastewater. Aerosol monitoring equipment, including both real-time and collection-based must be selected to be minimally invasive and not overly disruptive to either the patient or the caregivers.
Early in the COVID-19 pandemic risk assessments were undertaken at the National Centre for Infectious Diseases in Singapore (Chia et al. Citation2020) and at the National Quarantine Center and Nebraska Biocontainment Unit at the University of Nebraska Medical Center/Nebraska Medicine in Omaha, NE (Santarpia et al. Citation2020). Both studies began before the pandemic was declared by the World Health Organization, and the intent of both was to help inform healthcare practice, where either primary route of disease transmission was uncertain. Both studies assessed the risk of exposure from surfaces and aerosols by collecting and analyzing samples for SARS-CoV-2. High percentages of RNA-positive surface samples were observed, and RNA-positive air samples were also found in both cases. Many samples around patient toilets and on ventilation grates were also RNA positive. These assessments indicated potential risk from airborne, fomites, and fecal-oral transmission routes, with Santarpia et al. (Citation2020) providing evidence suggesting the possible, if not conclusive, culturability of both aerosol samples and surface samples from fomites. Subsequent studies (Lednicky et al. Citation2020; Santarpia, Herrera et al. Citation2022), confirmed that virus collected from air samples could be culturable, confirming the possibility of airborne transmission. The culturability of samples taken from fomites has not been consistently or conclusively demonstrated, nor has the culturability of virus found in human waste, indicating the risks from those modes of transmission are lower.
Disease surveillance
Public and animal health applications often have similar needs and approaches. Animal confinement operations and human settings (e.g. offices, schools, restaurants and large entertainment venues) share the common characteristic of large numbers of the same species in close quarters. Sampling to monitor disease transmission in both environments will have similar considerations. Following an outbreak, surface sampling of an unpopulated location can reveal key details about transmission and its likely source. During occupancy by large numbers of people or animals, aerosol and surface sampling can help identify areas where transmission is likely. Real-time bioaerosol sensors can help to map spatial and temporal variations in bioaerosol concentration. Routine wastewater monitoring can help identify emerging outbreaks and improve the implementation of aerosol and surface sampling to characterize the outbreak itself.
Untreated waste from animal confinement operations can be responsible for the contamination of surface waters (Crane et al. Citation1983), and monitoring effluents from these operations could be important to both understanding and mitigating surface water contamination and reducing human disease. One agricultural monitoring program examined effluent from five feedlot operations over six months to determine the prevalence of antibiotic-resistance genes as a function of antibiotic usage (Peak et al. Citation2007). Wastewater monitoring in Sweden has been shown to be capable of predicting disease outbreaks (Hellmér et al. Citation2014). In both of these cases, the intent of the program was to monitor for disease (or disease-causing agents) and to inform medical interventions (healthcare preparedness, in one, and antibiotic usage in animals, in the other). Both cases sampled the effluent streams for an extended period (3 and 6 months). Hellmér et al., used automated samplers already in place at the sewage treatment plant, while Peak et al. used manual sampling of the effluent. Both studies used a quantitative PCR process for identifying the pathogens or genes of interest. In both cases, the desired outcome was achieved. Monitoring for antibiotic-resistance genes over a period of time with different antibiotic usage strategies in the feedlots allowed the authors to say that the amount antibiotic use was correlated with the prevalence of antibiotic resistance in the bacterial population in the effluent, suggesting that curtailing antibiotics was necessary to prevent antibiotic-resistance genes in these bacterial populations (Peak et al. Citation2007). In Hellmér et al., wastewater monitoring identified the potential for developing a system that might predict outbreaks of enteric viruses and allow better preparedness (Hellmér et al. Citation2014).
Biodefense
Biodefense applications are more complex since they may involve entire buildings, large outdoor areas or even whole cities. The specifics of biodefense systems for military use are not often described in public literature. However, many commercial technologies were originally developed for military biodefense. Thee include many of the real-time biodetection technologies, such as UV-LIF, Raman, and single particle aerosol mass spectrometer, as well as high volume aerosol collection systems, and rapid, portable detection systems.
A combination of early warning systems with real-time, in situ, and stand-off sensors could be used to warn of a possible attack, initiate protective actions, and trigger deployment of more specific instruments to sample and detect agents of concern. In the event of an actual attack, surface sampling will be necessary to determine the extent of contamination and validate remediation activities. Wastewater sampling may be used to identify populations of infected individuals, so that they can be treated. Given the potential effects of a biological weapons attack, sampler selection should optimize detection and identification of the toxic contaminants. High volume, highly efficient air sampling coupled with sensitive detection and identification systems are needed.
Environmental characterization
Identifying sources of an emerging animal-to-human disease transmission often involves direct sampling of the animals themselves. However, studies referenced here have shown the value of aerosol and surface sampling in animal operations (Blachere et al. Citation2018; Gebhardt et al. Citation2021; O’Brien and Nonnenmann Citation2016). Routine air, surface, and wastewater monitoring around animal confinement operations can identify disease outbreaks that may become zoonotic in those environments.
Zoonotic diseases are often attributed to the consumption of wild animals for sustenance, but there may be other disease transmission routes from animal to human. Given the dearth of information, it may be useful to perform limited aerosol and/or surface sampling in locations inhabited by wild animals suspected of being the reservoir of a zoonotic disease. These types of studies are infrequent, but they have potential in the early identification of potential pathogens, as well as indications of potential routes of exposure.
Future directions
Much work still needs to be done to improve our understanding of disease transmission and our ability to protect against natural outbreaks and intentional attacks alike. Improvements are needed in sampling technology, procedures, and programs are all necessary.
Real-time bioaerosol measurements are a key area where improvements could make a difference in our ability to understand disease-causing aerosol particles. A real-time bioaerosol instrument that can identify an agent, at the species level, could alter the approach to virtually every biological sampling scenario.
Improvements to sample recovery and processing would improve all areas of biological sampling simultaneously. Downstream efficiencies in the process can alter the overall measurement sensitivity. Issues with recovering and preparing samples, especially in automated systems, can be a hurdle to the development of effective and sensitive sampling. Finally, improvements in the ability to rapidly and reliably determine the infectiousness or viability of infectious agents from environmental samples are needed. Difficulties in cultivating infectious agents, and therefore determine the actual hazard, from the environment prevent conclusive and rapid assessments of risk programs.
In addition to improvements in technology, the implementation of risk assessment, persistent disease surveillance, and more robust environmental characterization programs would improve understanding of disease transmission potential, identify outbreaks, and possibly identify potential emerging pathogens. Implementation of these programs could dramatically improve our ability to manage the disease burden in both human and animal populations.
Common guidelines for measurement and identification of infectious aerosols, which are agreed upon by the research and public health communities, are needed to better inform risk. This has been highlighted by the argument around the roles of aerosol particles in the transmission of COVID-19. Despite early evidence of the presence of aerosols containing SARS-CoV-2 RNA (Chia et al. Citation2020; Guo et al. Citation2020; Ong et al. Citation2020; Santarpia et al. Citation2020), public health organizations including the U.S. CDC and the WHO remained reluctant to acknowledge the potential role of aerosols and recommend precautions that would curb the transmission of the disease by aerosols. Even reports of cultivation of virus from aerosol samples, which were later published (Lednicky et al. Citation2020; Santarpia, Herrera et al. Citation2022), and published letters from the scientific community (Morawska and Milton Citation2020) were met with skepticism. The development of accepted guidelines for infectious bioaerosol sampling would improve the acceptance of new information in public health practices and allow more effective communication of new findings to both healthcare and the public. A recent special issue of Aerosol Science and Technology (Huffman and Ratnesar-Shumate Citation2020) collected numerous articles reviewing the state of the science, as a basis for developing guidelines and best practices across multiple disciplines of biological aerosol research.
A potential guideline for infectious bioaerosol measurement in the environment
In the areas of both wastewater sampling and surface sampling, accepted guidelines and authoritative analysis of the techniques are already available. The recent work by the U.S. National Academies has highlighted the effectiveness of the U.S. National Wastewater Surveillance System and outlined plan for extending it past the COVID-19 pandemic (Based Disease Surveillance for Public Health Action Citation2023). Guidelines for surface sampling for infectious disease have been outlined in detail by the U.S. Environmental Protection Agency (Silvestri et al. Citation2021). An aim of this review is to establish a framework for selecting and integrating (when applicable) the various modalities of environmental sampling to effectively sample the environment for infectious disease. However, detailed guidelines for aerosol sampling for infectious disease that are flexible enough to be implemented in a variety of locations where sampling would be beneficial are not as readily available.
The development of uniform guidelines for the measurement of infectious bioaerosols in ambient and built environments is limited by the state of understanding of bioaerosols in those environments. Without understanding what can be expected in any aerosol sample, it can be difficult to develop an objective standard to determine if one has collected and analyzed a representative sample. The sampling system should be able to detect a concentration of agent that would be able to infect the host.
The basis of this approach for a respiratory virus would be to define the infectious dose (ID) of the agent, the typical breathing rate (QB) of the host, and the time spent in the environment of concern. The expression
will then give the airborne concentration (CI) of the agent that could reasonably pose an infectious hazard to occupants in that time frame. By combining EquationEquation 2(2)
(2) with EquationEquation 1
(1)
(1) and the basic properties of an aerosol collector (flow rate: QS; Collection Efficiency: EA; and Sampling Time: TS) the following expression can be used to establish the minimum performance characteristics of the aerosol sampling and analysis system:
where EA = ES, in Equationequation (1)(1)
(1) . If molecular techniques are used for the analysis, physical collection efficiency can be used for EA, whereas if culture techniques are used, EA should represent the biological collection efficiency (for examples of physical and biological collection efficiency measurements see Dybwad, Skogan, and Martha Blatny Citation2014; Ratnesar-Shumate et al. Citation2021). Particle size will be a factor to consider, so the EA and ID values used should be based on the particle size that is most important for infection, which may vary from agent to agent.
This standardized approach allows for flexibility in the types of both samplers and analyses. However, it requires that the efficiencies of the sample and analysis steps be well understood before implementation. For the particle collector, the size-resolved physical and biological collection efficiency, and the intake flow rate must be measured. For the analysis process, the recovery efficiency, and analyte extraction efficiency must be characterized. In addition, sampling times and liquid volumes used throughout the process must be recorded and considered.
Once the properties of the sampler and analysis platform are characterized, optimization of the other parameters can be used to improve the performance of the system. For example, samples from multiple aerosol collectors could be aggregated to increase the volume of air sampled. Samplers that collect into liquid or onto soluble material could be used to maximize the recovery efficiency. In the case where the ID is unknown, such as for a novel pathogen, an infectious dose of unity can be used, in an abundance of caution. The flexibility of this type of approach is important for ensuring data comparability between studies done with different equipment and different analysis approaches.
Conclusion
This review has established a simple framework to aid in the design or evaluation of multimodal sampling systems that may include aerosol, surface and water/wastewater sampling. Accepted guidelines for both wastewater and surface sampling exist, while aerosol sampling for infectious disease is still an area where accepted guidelines are not as well established. The review of recent advances in bioaerosol, collection, measurement and analysis demonstrates that due to the wide variety of potential technologies that may be employed to sample the air for infectious disease flexible, but generally accepted guidelines, are still needed for aerosol measurements of infectious disease. The proposal of a guideline that is based on the potential for an infectious aerosol to cause disease highlights the need to understand several parameters of every aerosol system that might be used to sample for infectious aerosol particles (air flow rate, physical and biological collection efficiency, sampling time) and careful characterization of the efficiencies in the downstream analysis process. Adoption of simple and flexible guidelines for biological sampling for infectious diseases will allow it to be more readily and effectively incorporated into actionable information for public health.
Lexicon
Aerosol: a colloidal suspension of particles in a gas, typically air
Aerosol particle: the particulate component of an aerosol. Aerosol particles can range from a few nanometers in size up to several hundred micrometers (105 nanometers).
Eluant: a fluid used to remove a substance for subsequent analysis
Fluorophore: a molecule with fluorescent properties
Fomite: materials to which infectious agents might adhere, including clothing, utensils, and furniture
Metagenomics: the study of all nucleotide sequences isolated from all of the organisms in a sample
Mie Scattering: In terms of particle measurement, it refers to elastic scattering of light by particles with a similar or larger diameter than the wavelength of the light. The Mie scattering signal is proportional to the square of the particle diameter, and so can be used to determine particle diameter.
Polymerase chain reaction (PCR and various types):a technique that can copy specific DNA segments millions of times using special enzymes (polymerases). Quantitative PCR (qPCR) is a method to quantify the initial amount of DNA using fluorescent molecules. Reverse transcriptase (RT) PCR is a method to first convert RNA to DNA, using an enzyme, and then amplify it using the PCR process.
Zoonosis or Zoonotic disease: a type of disease which can be transmitted to humans from animals
Disclosure statement
Dr. Santarpia is a paid consultant for both Inspirotec and Poppy Health, both of whom develop and provide indoor infectious disease and allergen air monitoring services and devices. The authors do not endorse any of the specific products mentioned by name in this review.
Additional information
Notes on contributors
Joshua L. Santarpia
Joshua L. Santarpia is an Associate Professor of Microbiology and Pathology, Associate Director for Academic Affairs in the Global Center for Health Security, and Program Director for Biodefense and Health Security Degree Program at the University of Nebraska Medical Center. He is also the Science and Technology Advisor for the National Strategic Research Institute at the University of Nebraska. He completed his graduate studies at Texas A&M University and has held past positions at the Edgewood Chemical and Biological Center, the Johns Hopkins University Applied Physics Laboratory, and immediately prior to joining UNMC was a distinguished staff member at the Sandia National Laboratories. His work is generally in the field of aerobiology, the study of airborne microorganisms. He has worked extensively on biological sensors, building and facility sensing networks, and has developed aerosol measurement tools, including those for unmanned aerial vehicles and for biodetection/collection activities for both DoD and DHS. He has worked extensively to understand optical and other signatures that can be used to detect and identify biological aerosol and studied how those signatures change over time. He has developed novel methods to study bioaerosol hazards in medical environments. Most recently, he has applied these methods to characterizing SARS-CoV-2 aerosol in the patient environment and characterizing aerosol risk in public spaces.
Elizabeth Klug
Elizabeth A. Klug is a Ph.D. candidate for Drs. Joshua Santarpia and St. Patrick Reid. She is pursuing a Ph.D. in the Immunology, Pathology, and Infectious Disease (IPID) program at the University of Nebraska Medical Center (UNMC). Elizabeth received her Bachelor of Science in Biology from the University of Nebraska at Omaha (UNO). Elizabeth's research experience includes viral aerosol sampling in the medical environment and investigating stability of aerosolized proteins and viruses when exposed to environmental conditions.
Ashley Ravnholdt
Ashley R. Ravnholdt is a Ph.D. student, for Dr. Joshua Santarpia. She is a pursuing a Ph.D. in the Biological Defense and Health Security program in the Global Center for Health Security at the University of Nebraska Medical Center. Ashley received her Bachelor of Science in Biology from the University of Nebraska at Omaha (UNO). Ashley's research experience includes viral aerosol sampling in the medical environment, methods to detect airborne microorganisms in indoor environments and characterize aerosols generated in biological laboratories.
Sean M. Kinahan
Sean Kinahan is a Senior Scientist at the National Strategic Research Institute and a Ph.D. candidate, for Dr. Joshua Santarpia, in the Biological Defense and Health Security program in the Global Center for Health Security at the University of Nebraska Medical Center. He received a Bachelor of Science in Biomedical Engineering from the University of Virginia and a Master of Science in Applied Biomedical Engineering from Johns Hopkins University. Sean’s research includes viable bioaerosol sampling techniques, bioaerosol fate in laboratory and outdoor environments, and how biological signatures evolve or degrade over time both in the environment and upon collection. During the COVID pandemic he studied particle fate and exposure risks on board DoD and Civilian aircraft.
References
- Addor, Y.S., D. Baumgardner, D. Hughes, N. Newman, R. Jandarov, and T. Reponen. 2022. Assessing residential indoor and outdoor bioaerosol characteristics using the ultraviolet light-induced fluorescence-based wideband integrated bioaerosol sensor. Environ. Sci. 24 (10):1790–804. doi:10.1039/D2EM00177B.
- Ai, Y., C. Wang, Y.L. Pan, and G. Videen. 2022. Characterization of single fungal aerosol particles in a reactive atmospheric environment using time-resolved Optical Trapping-Raman Spectroscopy (OT-RS). Environ. Sci. 2 (4):591–600. doi:10.1039/D2EA00030J.
- Alsved, M., C.J. Fraenkel, M. Bohgard, A. Widell, A. Söderlund-Strand, P. Lanbeck, T. Holmdahl, C. Isaxon, A. Gudmundsson, P. Medstrand, et al. 2020. Sources of airborne norovirus in hospital outbreaks. Clin. Infect. Dis. 70 (10):2023–28. doi:10.1093/cid/ciz584.
- Anderson, B.D., J. A. Lednicky, M. Torremorell, and G. C. Gray. 2017. The use of bioaerosol sampling for airborne virus surveillance in swine production facilities: A mini review. Front. Vet. Sci. 4 (July). doi: 10.3389/fvets.2017.00121.
- Andreotti, P. E., G. V. Ludwig, A. Harwood Peruski, J.J. Tuite, S. S. Morse, and L. F. Peruski. 2003. Immunoassay of infectious agents. Bio.Techniques 35 (4):850–59. doi:10.2144/03354ss02.
- Auger, J.C., K.B. Aptowicz, R.G. Pinnick, Y.L. Pan, and R.K. Chang. 2007. Angularly resolved light scattering from aerosolized spores: Observations and calculations. Opt. Lett. 32 (22):3358. doi:10.1364/OL.32.003358.
- Augusto, M.R., I. Carolina Mantovani Claro, A. Kaori Siqueira, G. Santos Sousa, C. Roberto Caldereiro, A. Feliciano Alves Duran, T.B. de Miranda, L. de Moraes Bomediano Camillo, A. Diniz Cabral, and R. de Freitas Bueno. 2022. Sampling strategies for wastewater surveillance: Evaluating the variability of SARS-COV-2 RNA concentration in composite and grab samples. J. Environ. Chem. Eng. 10:3. doi:10.1016/J.JECE.2022.107478.
- Barksdale, A.N., W.G. Zeger, J.L. Santarpia, V.L. Herrera, D. N. Ackerman, J.J. Lowe, and M.C. Wadman. 2021. Implementation of a COVID-19 cohort area resulted in no surface or air contamination in surrounding areas in one academic emergency department. Am. J. Emerg. Med. 47 (September):253–57. doi:10.1016/j.ajem.2021.04.082.
- Bauer, A.J.R., and D.M. Sonnenfroh. 2009. Spark-induced breakdown spectroscopy-based classification of bioaerosols. In 2009 IEEE international workshop on Safety, Security & Rescue Robotics (SSRR 2009), 1–4. IEEE. doi:10.1109/SSRR.2009.5424145.
- Beedham, R.J., and C.H. Davies. 2021. The UK biological-warfare program: Dual-use contributions to the field of aerobiology. Nonproliferation Review. doi:10.1080/10736700.2020.1823621.
- Bert, F., G. Scaioli, M. Rosaria Gualano, S. Passi, M. Lucia Specchia, C. Cadeddu, C. Viglianchino, and R. Siliquini. 2014. Norovirus outbreaks on commercial cruise ships: A systematic review and new targets for the public health agenda. Food Environ. Virol. 6 (2):67–74. doi:10.1007/s12560-014-9145-5.
- Bhangar, S., R.I. Adams, W. Pasut, J.A. Huffman, E.A. Arens, J.W. Taylor, T.D. Bruns, and W.W. Nazaroff. 2016. Chamber bioaerosol study: Human emissions of size‐resolved fluorescent biological aerosol particles. Indoor Air 26 (2):193–206. doi:10.1111/ina.12195.
- Bhangar, S., J.A. Huffman, and W.W. Nazaroff. 2014. Size‐resolved fluorescent biological aerosol particle concentrations and occupant emissions in a university classroom. Indoor Air 24 (6):604–17. doi:10.1111/ina.12111.
- Bivins, A., D. Kaya, W. Ahmed, J. Brown, C. Butler, J. Greaves, R. Leal, K. Maas, G. Rao, S. Sherchan, et al. 2022. Passive sampling to scale wastewater surveillance of infectious disease: Lessons learned from COVID-19. Sci. Total Environ. 835 (August):155347. doi:https://doi.org/10.1016/J.SCITOTENV.2022.155347.
- Blachere, F.M., G. Cao, W.G. Lindsley, J.D. Noti, and D.H. Beezhold. 2011. Enhanced detection of infectious airborne influenza virus. J. Virol. Method 176 (1–2):120–24. doi:10.1016/j.jviromet.2011.05.030.
- Blachere, F.M., W.G. Lindsley, T.A. Pearce, S.E. Anderson, M. Fisher, R. Khakoo, B.J. Meade, O. Lander, S. Davis, R. Thewlis, et al. 2009. Measurement of airborne influenza virus in a hospital emergency department. Clin. Infect. Dis. 48 (4):438–40. doi:https://doi.org/10.1086/596478.
- Blachere, F.M., W.G. Lindsley, A.M. Weber, D.H. Beezhold, R.E. Thewlis, K.R. Mead, and J.D. Noti. 2018. Detection of an avian lineage influenza A(H7N2) virus in air and surface samples at a New York City feline quarantine facility. Influenza Other Respi. Viruses 12 (5):613–22. doi:10.1111/irv.12572.
- Bonifait, L., R. Charlebois, A. Vimont, N. Turgeon, M. Veillette, Y. Longtin, J. Jean, and C. Duchaine. 2015. Detection and quantification of airborne norovirus during outbreaks in healthcare facilities. Clin. Infect. Dis. 61 (3):299–304. doi:10.1093/cid/civ321.
- Bonk, T., and A. Humeny. 2001. MALDI-TOF-MS analysis of protein and DNA. The Neurosci. 7 (1):6–12. doi:10.1177/107385840100700104.
- Boxman, I.L.A., L. Verhoef, R. Dijkman, G. Hägele, N.A.J.M. Te Loeke, and M. Koopmans. 2011. Year-round prevalence of norovirus in the environment of catering companies without a recently reported outbreak of gastroenteritis. Appl. Environ. Microbiol. 77 (9):2968–74. doi:10.1128/AEM.02354-10.
- Boyain-Goitia, A., R. David, C.S. Beddows, C.G. Ben, and H. T. Helmut. 2003. Single-pollen analysis by laser-induced breakdown spectroscopy and raman microscopy. Appl. Opt. 42 (30):6119. doi:10.1364/AO.42.006119.
- Brockwell-Staats, C., R.G. Webster, and R.J. Webby. 2009. Diversity of influenza viruses in swine and the emergence of a novel human pandemic influenza a (H1N1). Influenza Other Respi Viruses 3 (5):207–13. doi:10.1111/j.1750-2659.2009.00096.x.
- Burton, N.C., S.A. Grinshpun, and T. Reponen. 2006. Physical collection efficiency of filter materials for bacteria and viruses. Ann. Occup. Hyg. October. doi:10.1093/annhyg/mel073.
- Cabalo, J., R. Sickenberger, M. de Lucia, J. Briles, A. Poldmae, and S. David. 2005. Overview of the TAC-BIO sensor, ed. Edward M. Carapezza, 293. doi:10.1117/12.606902
- Cantú, V.J., P. Belda-Ferre, R.A. Salido, R. Tsai, B. Austin, W. Jordan, M. Asudani, A. Walster, C.G. Magallanes, H. Valentine, et al. 2022. Implementation of practical surface SARS-CoV-2 surveillance in school settings. MSystems 7 (4). doi: 10.1128/mSystems.00109-22.
- Cao, G., J.D. Noti, F.M. Blachere, W.G. Lindsley, and D.H. Beezhold. 2011. Development of an improved methodology to detect infectious airborne influenza virus using the NIOSH bioaerosol sampler. J. Environ Monitor 13 (12):3321–28. doi:10.1039/c1em10607d.
- Carducci, A., M. Verani, B. Casini, A. Giuntini, F. Mazzoni, E. Rovini, A. Passaglia, L. Giusti, A. Valenza, and R. Lombardi. 2001. Detection and potential indicators of the presence of hepatitis C virus on surfaces in hospital settings. Lett. Appl. Microbiol. 34 (3):189–93. doi:10.1046/j.1472-765x.2002.01066.x.
- Chia, P.Y., K. Kelli Coleman, Y. Kim Tan, S. Wei Xiang Ong, M. Gum, S. Kiang Lau, X. Fang Lim, A. S. Lim, S. Sutjipto, P. H. Lee, et al. 2020. Detection of air and surface contamination by SARS-CoV-2 in hospital rooms of infected patients. Nat. Commun. 11 (1):2800. doi:10.1038/s41467-020-16670-2.
- Chitnis, A., D. Rawls, and J. Moore. 2000. Origin of HIV type 1 in colonial French equatorial Africa? AIDS Res. Hum. Retroviruses 16 (1):5–8. doi:10.1089/088922200309548.
- Cho, Y.S., H. Ri Kim, K. Hyun Sik, S. Bin Jeong, B. Chan Kim, and J. Hee Jung. 2020. Continuous surveillance of bioaerosols on-site using an automated bioaerosol-monitoring system. ACS Sens. 5 (2):395–403. doi:10.1021/acssensors.9b02001.
- Chow, J.C., J.G. Watson, X. Wang, B. Abbasi, W. Randolph Reed, and D. Parks. 2022. Review of filters for air sampling and chemical analysis in mining workplaces. Minerals 12 (10):1314. doi:10.3390/min12101314.
- Christesen, S.D., C.N. Merrow, M.S. DeSha, A. Wong, M.W. Wilson, and J.C. Butler. 1994. Ultraviolet fluorescence lidar detection of bioaerosols, ed. W. A. Flood and W. B. Miller, 228. doi:10.1117/12.177988
- Clendening, L. 1942. Source book of medical history. 1st ed. New York, NY: Harper & Brothers.
- Coleman, K.K., and W.V. Sigler. 2020. Airborne influenza a virus exposure in an elementary school. Sci. Rep. 10 (1):1859. doi:10.1038/s41598-020-58588-1.
- Conant, J.B. 1957. CASE 7. Pasteur’s and Tyndall’s study of spontaneous generation. In Harvard case histories in experimental science, Vol. II, 486–539. Harvard University Press. doi:10.4159/harvard.9780674598713.c4.
- Crane, S.R., J.A. Moore, M.E. Grismer, and J.R. Miner. 1983. Bacterial pollution from agricultural sources: A review. Transactions of the ASAE 26 (3):858–66. doi:10.13031/2013.34036.
- Crowe, J., A.T. Schnaubelt, S. SchmidtBonne, K. Angell, J. Bai, T. Eske, M. Nicklin, C. Pratt, B. White, B. Crotts-Hannibal, et al. 2021. Assessment of a program for SARS-CoV-2 screening and environmental monitoring in an urban public school district. JAMA Network Open. 4 (9):e2126447. doi:https://doi.org/10.1001/jamanetworkopen.2021.26447.
- Cunha, C.B., and S.M. Opal. 2014. Middle East Respiratory Syndrome (MERS). Virulence 5 (6):650–54. doi:10.4161/viru.32077.
- Damit, B., and M. Antoine. 2021. Portable biological spectroscopy: Field applications. In Portable spectroscopy and spectrometry, 545–63. Wiley. doi:10.1002/9781119636489.ch22.
- DeFreez, R., E. Merrill, S. Albanna, B. Davis, and C. Charles. 2005. Design considerations and performance characteristics of airsentinel: A New UV-LIF Bio-aerosol threat detection trigger, ed. John C. Carrano, Arturas Zukauskas, Anthony W. Vere, James G. Grote, and François Kajzar, 59900O. doi:10.1117/12.634389
- Doughty, D.C., and S.C. Hill. 2017. Automated aerosol raman spectrometer for semi-continuous sampling of atmospheric aerosol. J. Quant. Spectrosc. Radiat. Transf. 188 (February):103–17. doi:10.1016/J.JQSRT.2016.06.042.
- Downey, A.S., S.M. Da Silva, N.D. Olson, J.J. Filliben, and J.B. Morrow. 2012. Impact of processing method on recovery of bacteria from wipes used in biological surface sampling. Appl. Environ. Microbiol. 78 (16):5872–81. doi:10.1128/AEM.00873-12.
- Duan, H., L. Xuan, A. Mei, L. Ping, Y. Liu, L. Xiaofeng, L. Weiwei, C. Wang, and S. Xie. 2021. The diagnostic value of metagenomic next⁃generation sequencing in infectious diseases. BMC Infect. Dis. 21 (1):62. doi:10.1186/s12879-020-05746-5.
- Dybwad, M., G. Skogan, and J. Martha Blatny. 2014. Comparative testing and evaluation of nine different air samplers: End-to-end sampling efficiencies as specific performance measurements for bioaerosol applications. Aerosol Sci. Technol. 48 (3):282–95. doi:10.1080/02786826.2013.871501.
- Eversole, J.D., J.J. Hardgrove, W.K. Cary, D.P. Choulas, and M. Seaver. 1999. Continuous, rapid biological aerosol detection with the use of UV fluorescence: Outdoor test results. Field. Anal Chem Technol 3 (4–5):249–59. https://doi.org/10.1002/(SICI)1520-6521(1999)3:4/5<249::AID-FACT4>3.0.CO;2-O
- Fergenson, D.P., M.E. Pitesky, H.J. Tobias, P.T. Steele, G.A. Czerwieniec, S.C. Russell, C.B. Lebrilla, J.M. Horn, K.R. Coffee, A. Srivastava, et al. 2004. Reagentless detection and classification of individual bioaerosol particles in seconds. Anal. Chem. 76 (2):373–78. doi:10.1021/ac034467e.
- Fewtrell, L., and J. Bartram. 2013. Water quality : Guidelines, standards, and Health : Assessment of risk and risk management for water-related infectious disease. doi:10.2166/9781780405889.
- Fong, M.W., N.H.L. Leung, J. Xiao, D.K.W. Chu, S.M.S. Cheng, S. Hau Chi, L. Yuguo, D.K.M. Ip, J.S. Malik Peiris, and B.J. Cowling. 2020. Presence of influenza virus on touch surfaces in kindergartens and primary schools. J. Infect. Dis. 222 (8):1329–33. doi:10.1093/infdis/jiaa114.
- Fredricks, D.N., and D.A. Relman. 1999. Application of polymerase chain reaction to the diagnosis of infectious diseases. Clin. Infect. Dis. 29 (3):475–86. http://www.jstor.org/stable/4460932.
- Fu, R., C. Wang, O. Muñoz, G. Videen, J.L. Santarpia, and Y.L. Pan. 2017. Elastic back-scattering patterns via particle surface roughness and orientation from single trapped airborne aerosol particles. J. Quant. Spectrosc. Radiat. Transf. 187 (January):224–31. doi:10.1016/j.jqsrt.2016.09.018.
- Galindo, I., and C. Alonso. 2017. African swine fever virus: A review. Viruses 9 (5):103. doi:10.3390/v9050103.
- Gard, E., J.E. Mayer, B.D. Morrical, T. Dienes, D.P. Fergenson, and K.A. Prather. 1997. Real-time analysis of individual atmospheric aerosol particles: Design and performance of a portable ATOFMS. Anal. Chem. 69 (20):4083–91. doi:10.1021/ac970540n.
- Garza, A.G., S.M. Van Cuyk, M.J. Brown, and K.M. Omberg. 2005. Detection of the urban release of a bacillus anthracis simulant by air sampling. Biosecur. Bioterror. 12:2. doi:10.1089/bsp.2013.0086.
- Gebhardt, J.T., S.S. Dritz, C. Grace Elijah, C.K. Jones, C.B. Paulk, and J.C. Woodworth. 2021. Sampling and detection of African swine fever virus within a feed manufacturing and swine production system. Transbound Emerg Dis 69 (1):103–14. doi:10.1111/tbed.14335.
- Ghosh, S., M. Kumar, M. Santiana, A. Mishra, M. Zhang, H. Labayo, A.M. Chibly. 2022. Enteric viruses replicate in salivary glands and infect through saliva. Nature 607 (7918):345–50. doi:10.1038/s41586-022-04895-8.
- Gieray, R.A., P.T.A. Reilly, M. Yang, W.B. Whitten, and J.M. Ramsey. 1997. Real-time detection of individual airborne bacteria. J. Microbiol. Methods 29 (3):191–99. doi:10.1016/S0167-7012(97)00049-3.
- Gonçalves, J., P.G. da Silva, L. Reis, M. São José Nascimento, T. Koritnik, M. Paragi, and J.R. Mesquita. 2021. Surface contamination with SARS-CoV-2: A systematic review. Sci. Total Environ. 798 (December):149231. doi:10.1016/j.scitotenv.2021.149231.
- Gordon, J., P. Gandhi, G. Shekhawat, A. Frazier, J. Hampton-Marcell, and J.A. Gilbert. 2015. A simple novel device for air sampling by electrokinetic capture. Microbiome 3 (1):79. doi:10.1186/s40168-015-0141-2.
- Grayson, S.A., P.S. Griffiths, M.K. Perez, and G. Piedimonte. 2017. Detection of airborne respiratory syncytial virus in a pediatric acute care clinic. Pediatr. Pulmonol. 52 (5):684–88. doi:10.1002/ppul.23630.
- Guo, Z.D., Z. Yi Wang, S. Feng Zhang, L. Xiao, L. Lin, L. Chao, Y. Cui, R.-B. Fu, Y.-Z. Dong, X.-Y. Chi, et al. 2020. Aerosol and surface distribution of severe acute respiratory syndrome coronavirus 2 in hospital wards, Wuhan, China, 2020. Emerging Infect. Dis. 26 (7):1583–91. doi:10.3201/EID2607.200885.
- Hairston, P.P., H. Jim, and F.R. Quant. 1997. Design of an instrument for real-time detection of bioaerosols using simultaneous measurement of particle aerodynamic size and intrinsic fluorescence. J. Aerosol. Sci. 28 (3):471–82. doi:10.1016/S0021-8502(96)00448-X.
- Han, T.T., N.T. Myers, S. Manibusan, and G. Mainelis. 2022. Development and optimization of Stationary Electrostatic Bioaerosol Sampler (SEBS) for viable and culturable airborne microorganisms. J. Aerosol. Sci. 162 (May):105951. doi:10.1016/j.jaerosci.2022.105951.
- Han, T.T., N.M. Thomas, and G. Mainelis. 2017. Design and development of a self-contained Personal Electrostatic Bioaerosol Sampler (PEBS) with a wire-to-wire charger. Aerosol Sci. Technol. 51 (8):903–15. doi:10.1080/02786826.2017.1329516.
- Han, T.T., N.M. Thomas, and G. Mainelis. 2018. Performance of Personal Electrostatic Bioaerosol Sampler (PEBS) when collecting airborne microorganisms. J. Aerosol. Sci. 124 (October):54–67. doi:10.1016/j.jaerosci.2018.07.004.
- Harris, J.C., M.S. Collins, P.H. Huang, C.M. Schramm, T. Nero, J. Yan, and T.S. Murray. 2022. Bacterial surface detachment during nebulization with contaminated reusable home nebulizers. Microbiol. Spectr. 10:1. doi:10.1128/spectrum.02535-21.
- Healy, D.A., J.A. Huffman, D.J. O’Connor, C. Pöhlker, U. Pöschl, and J.R. Sodeau. 2014. Ambient measurements of biological aerosol particles near Killarney, Ireland: A comparison between real-time fluorescence and microscopy techniques. Atmos. Chem. Phys. 14 (15):8055–69. doi:10.5194/acp-14-8055-2014.
- Hellmér, M., N. Paxéus, L. Magnius, L. Enache, B. Arnholm, A. Johansson, T. Bergström, and H. Norder. 2014. Detection of pathogenic viruses in sewage provided early warnings of hepatitis a virus and norovirus outbreaks. Appl. Environ. Microbiol. 80 (21):6771–81. doi:10.1128/AEM.01981-14.
- Herzog, A.B., A.K. Pandey, D. Reyes-Gastelum, C.P. Gerba, J.B. Rose, and S.A. Hashsham. 2012. Evaluation of sample recovery efficiency for bacteriophage P22 on fomites. Appl. Environ. Microbiol. 78 (22):7915–22. doi:10.1128/AEM.01370-12.
- Hinds, W.C., B.D. Beck, J.D. Brain, W.C. Hinds, S.G. Baron, and L. Weil. 1982. Aerosol technology: Properties, behavior, and measurement of airborne particles. Ann. Occup. Hyg. 26 (1–4):435–48.
- Hindson, B.J., K.D. Ness, D.A. Masquelier, P. Belgrader, N.J. Heredia, A.J. Makarewicz, I.J. Bright, M.Y. Lucero, A.L. Hiddessen, T.C. Legler, et al. 2011. High-throughput droplet digital PCR system for absolute quantitation of DNA copy number. Anal. Chem. 83 (22):8604–10. doi:10.1021/ac202028g.
- Ho, J. 2002. Future of biological aerosol detection. Anal. Chim. Acta 457 (1):125–48. doi:10.1016/S0003-2670(01)01592-6.
- Ho, J., M. Spence, and P. Hairston. 1999. Measurement of biological aerosol with a Fluorescent Aerodynamic Particle Sizer (Flaps): Correlation of optical data with biological data. Aerobiologia 15 (4):281–91. doi:10.1023/A:1007647522397.
- Hovi, T., L.M. Shulman, H. van der Avoort, J. Deshpande, M. Roivainen, and E.M. de Gourville. 2012. Role of environmental poliovirus surveillance in global polio eradication and beyond. Epidemiol. Infect. 140 (1):1–13. doi:10.1017/S095026881000316X.
- Huffman, J.A., and L.S. Joshua. 2017. Online techniques for quantification and characterization of biological aerosols. In Microbiology of aerosols, 83–114. Hoboken, NJ, USA: John Wiley & Sons, Inc. doi:10.1002/9781119132318.ch1d.
- Huffman, J.A., A.E. Perring, N.J. Savage, B. Clot, B. Crouzy, F. Tummon, O. Shoshanim, B. Damit, J. Schneider, V. Sivaprakasam, et al. 2020. Real-time sensing of bioaerosols: Review and current perspectives. Aerosol Sci. Technol. 54 (5):465–95. doi:10.1080/02786826.2019.1664724.
- Huffman, J.A., and S. Ratnesar-Shumate. 2020. Preface for special issue ‘Bioaerosol research: Methods, challenges, and perspectives.’. Aerosol Sci. Technol. 54 (5):463–64. doi:10.1080/02786826.2020.1745012.
- Huffman, J.A., B. Sinha, R.M. Garland, A. Snee-Pollmann, S.S. Gunthe, P. Artaxo, S.T. Martin, M.O. Andreae, and U. Pöschl. 2012. Size distributions and temporal variations of biological aerosol particles in the amazon rainforest characterized by microscopy and real-time UV-APS fluorescence techniques during AMAZE-08. Atmos. Chem. Phys. 12 (24): 11997–19. doi:10.5194/acp-12-11997-2012.
- Huffman, J.A., B. Treutlein, and U. Pöschl. 2010. Fluorescent biological aerosol particle concentrations and size distributions measured with an Ultraviolet Aerodynamic Particle Sizer (UV-APS) in Central Europe. Atmos. Chem. Phys. 10 (7):3215–33. doi:10.5194/acp-10-3215-2010.
- Hybl, J.D., G.A. Lithgow, and S.G. Buckley. 2003. Laser-induced breakdown spectroscopy detection and classification of biological aerosols. Appl. Spectrosc. 57 (10):1207–15. doi:10.1366/000370203769699054.
- Institute of Medicine (US) and National Research Council (US) Committee on Effectiveness of National Biosurveillance Systems: Biowatch and the Public Health System. 2011. Biowatch and public health surveillance: Evaluating systems for the early detection of biological threats. In BioWatch and public health surveillance, National Academies Press. doi:10.17226/12688
- Jamal, S.M., and G.J. Belsham. 2013. Foot-and-mouth disease: Past, present and future. Vet. Res. 44 (1):116. doi:10.1186/1297-9716-44-116.
- Julian, T.R., F.J. Tamayo, J.O. Leckie, and A.B. Boehm. 2011. Comparison of surface sampling methods for virus recovery from Fomites. Appl. Environ. Microbiol. 77 (19):6918–25. doi:10.1128/AEM.05709-11.
- Kalume, A., Z. Gong, C. Wang, J.L. Santarpia, and Y.-L. Pan. 2018. Detection and characterization of chemical and biological aerosols using laser-trapping single-particle raman spectroscopy. WIT Trans. Ecol. Environ. 230:323–29. doi:10.2495/AIR180301.
- Kalume, A., C. Wang, J.L. Santarpia, and Y.-L. Pan. 2018. Submicron position-resolved raman spectra for characterizing laser-trapped single airborne particles. In Optics InfoBase Conference Papers. Vol. Part F91T. doi:10.1364/TRANSLATIONAL.2018.JW3A.39.
- Katayama, H., E. Haramoto, K. Oguma, H. Yamashita, A. Tajima, H. Nakajima, and S. Ohgaki. 2007. One-year monthly quantitative survey of noroviruses, enteroviruses, and adenoviruses in wastewater collected from six plants in Japan. Water Res. 42 (6–7):1441–48. doi:https://doi.org/10.1016/j.watres.2007.10.029.
- Kaye, P.H., J.E. Barton, E. Hirst, and J.M. Clark. 2000. Simultaneous light scattering and intrinsic fluorescence measurement for the classification of airborne particles. Appl. Opt. 39 (21):3738. doi:10.1364/AO.39.003738.
- Kaye, P.H., W.R. Stanley, E. Hirst, E.V. Foot, K.L. Baxter, and S.J. Barrington. 2005. Single particle multichannel bio-aerosol fluorescence sensor. Opt Express 13 (10):3583. doi:10.1364/OPEX.13.003583.
- Kim, K.H., E. Kabir, and S. Ara Jahan. 2018. Airborne bioaerosols and their impact on human health. J. Environ. Sci. 67 (May):23–35. doi:10.1016/j.jes.2017.08.027.
- Kinahan, S.M., D.B. Silcott, B.E. Silcott, R.M. Silcott, P.J. Silcott, B.J. Silcott, S.L. Distelhorst, V.L. Herrera, D.N. Rivera, K.K. Crown, et al. 2021. Aerosol tracer testing in Boeing 767 and 777 aircraft to simulate exposure potential of infectious aerosol such as SARS-CoV-2. PLoS ONE. 16 (12):e0246916. doi:10.1371/journal.pone.0246916.
- Kinahan, S.M., M.S. Tezak, C.M. Siegrist, G. Lucero, B.L. Servantes, J.L. Santarpia, A. Kalume, J. Zhang, M. Felton, C.C. Williamson, et al. 2019. Changes of fluorescence spectra and viability from aging aerosolized E. Coli cells under various laboratory-controlled conditions in an advanced rotating drum. Aerosol. Sci. Technol. 53 (11):1261–76. doi:10.1080/02786826.2019.1653446.
- Kleefsman, W.A., M.A. Stowers, P.J.T. Verheijen, and J.C.M. Marijnissen. 2008. Single particle mass spectrometry–bioaerosol analysis by MALDI MS. KONA Powder Part. J. 26: 205–14. doi:10.14356/kona.2008018.
- Kleefsman, I., M.A. Stowers, P.J.T. Verheijen, A.L. van Wuijckhuijse, C.E. Kientz, and J.C.M. Marijnissen. 2007. Bioaerosol analysis by single particle mass spectrometry. Part. Part. Syst. Charact. 24 (2):85–90. doi:10.1002/ppsc.200601049.
- Kmush, B.L., D. Monk, H. Green, D.A. Sachs, T. Zeng, and D.A. Larsen. 2022. Comparability of 24-hour composite and grab samples for detection of SARS-2-CoV RNA in wastewater. FEMS Microbes 3: June. doi: 10.1093/FEMSMC/XTAC017.
- Kokkoris, V., E. Vukicevich, A. Richards, C. Thomsen, and M.M. Hart. 2021. Challenges using droplet digital PCR for environmental samples. Appl. Microbiol. 1 (1):74–88. doi:10.3390/applmicrobiol1010007.
- Kosowska, A., J.A. Barasona, S. Barroso-Arévalo, B. Rivera, L. Domínguez, and J.M. Sánchez-Vizcaíno. 2021. A new method for sampling African swine fever virus genome and its inactivation in environmental samples. Sci. Rep. 11:11. doi:10.1038/s41598-021-00552-8.
- LaTurner, Z.W., D.M. Zong, P. Kalvapalle, K. Reyes Gamas, A. Terwilliger, T. Crosby, P. Ali, V. Avadhanula, H.H. Santos, K. Weesner, et al. 2021. Evaluating recovery, cost, and throughput of different concentration methods for SARS-CoV-2 wastewater-based epidemiology. Water Res. 197 (June):117043. doi:10.1016/J.WATRES.2021.117043.
- Laumbach, R.J., G. Mainelis, K.G. Black, N.T. Myers, P. Ohman-Strickland, S. Alimokhtari, S. Hastings, A. Legard, A. de Resende, L. Calderón, et al. 2022. Presence of SARS-CoV-2 aerosol in residences of adults with COVID-19. Ann. Am. Thorac. Soc. 19 (2):338–41. doi:10.1513/AnnalsATS.202107-847RL.
- Lecours, B., M.V. Pascale, D. Marsolais, and C. Duchaine. 2012. Characterization of Bioaerosols from Dairy Barns: Reconstructing the Puzzle of Occupational Respiratory Diseases by Using Molecular Approaches. Appl. Environ. Microbiol. 78 (9):3242–48. doi:10.1128/AEM.07661-11.
- Lednicky, J.A., M. Lauzardo, M.M. Alam, M.A. Elbadry, C.J. Stephenson, J.C. Gibson, and J. Glenn Morris. 2021a. Isolation of SARS-CoV-2 from the air in a car driven by a COVID patient with mild illness. Int. J. Infect. Dis. 108 (July):212–16. doi:10.1016/j.ijid.2021.04.063.
- Lednicky, J.A., M. Lauzardo, M.M. Alam, M.A. Elbadry, C.J. Stephenson, J.C. Gibson, and J. Glenn Morris. 2021b. Isolation of SARS-CoV-2 from the air in a car driven by a COVID patient with mild illness. Int. J. Infect. Dis. 108 (July):212–16. doi:10.1016/j.ijid.2021.04.063.
- Lednicky, J.A., Z.H.F. Michael Lauzard, A. Jutla, T.B. Tilly, M. Gangwar, M. Usmani, M. Usmani, S.N. Shankar, K. Mohamed, A. Eiguren-Fernandez, et al. 2020. Viable SARS-CoV-2 in the air of a hospital room with COVID-19 patients. Int. J. Infect. Dis. 100 (November):476–82. doi:10.1016/J.IJID.2020.09.025.
- Lednicky, J., M. Pan, J. Loeb, H. Hsieh, A. Eiguren-Fernandez, Z.H.F. Susanne Hering, and W. Chang-Yu. 2016. Highly efficient collection of infectious pandemic influenza H1N1 Virus (2009) through laminar-flow water based condensation. Aerosol Sci. Technol. 50 (7): doi:10.1080/02786826.2016.1179254. i–iv.
- Leung, K., E. Louca, M. Gray, G. Tipples, and A.L. Coates. 2005. Use of the next generation pharmaceutical impactor for particle size distribution measurements of live viral aerosol vaccines. J. Aerosol. Med. 18 (4):414–26. doi:10.1089/jam.2005.18.414.
- Li, C., G.W. Kattawar, and P. Yang. 2004. Effects of surface roughness on light scattering by small particles. J. Quant. Spectrosc. Radiat. Transf. 89 (1–4):123–31. doi:10.1016/j.jqsrt.2004.05.016.
- Lindsley, W.G., F.M. Blachere, R.E. Thewlis, A. Vishnu, K.A. Davis, G. Cao, J.E. Palmer, K.E. Clark, M.A. Fisher, R. Khakoo, et al. 2010. Measurements of airborne influenza virus in aerosol particles from human coughs. PLoS ONE. 5 (11):e15100. doi:10.1371/journal.pone.0015100.
- Lindsley, W.G., D. Schmechel, and B.T. Chen. 2006. A two-stage cyclone using microcentrifuge tubes for personal bioaerosol sampling. J. Environ Monitor 8 (11):1136. doi:10.1039/b609083d.
- Liu, Y., Z. Ning, Y. Chen, M. Guo, Y. Liu, N. Kumar Gali, L. Sun, Y. Duan, J. Cai, D. Westerdahl, et al. 2020. Aerodynamic analysis of SARS-CoV-2 in two Wuhan hospitals. Nature. 582 (7813):557–60. doi:https://doi.org/10.1038/s41586-020-2271-3.
- Li, J., S. Zuraimi, S. Schiavon, M. Pun Wan, J. Xiong, and K. Wai Tham. 2022. Diurnal trends of indoor and outdoor fluorescent biological aerosol particles in a tropical urban area. Sci. Total Environ. 848 (November):157811. doi:10.1016/j.scitotenv.2022.157811.
- Machalaba, C.C., S.E. Elwood, S. Forcella, K.M. Smith, K. Hamilton, K.B. Jebara, D.E. Swayne, R.J. Webby, E. Mumford, J.A.K. Mazet, et al. 2015. Global avian influenza surveillance in wild birds: A strategy to capture viral diversity. Emerging Infect. Dis. 21:4. doi:10.3201/eid2104.141415.
- Mainelis, G. 1999. Collection of airborne microorganisms by electrostatic precipitation. Aerosol. Sci. Technol. 30 (2):127–44. doi:10.1080/027868299304732.
- Mainelis, G. 2020. Bioaerosol sampling: Classical approaches, advances, and perspectives. Aerosol. Sci. Technol. 54 (5):496–519. doi:10.1080/02786826.2019.1671950.
- Mainelis, G., K. Willeke, A. Adhikari, T. Reponen, and S.A. Grinshpun. 2002. Design and collection efficiency of a new electrostatic precipitator for bioaerosol collection. Aerosol. Sci. Technol. 36 (11):1073–85. doi:10.1080/02786820290092212.
- Manibusan, S., and G. Mainelis. 2022. Passive bioaerosol samplers: A complementary tool for bioaerosol research. A review. J. Aerosol. Sci. 163 (June):105992. doi:10.1016/j.jaerosci.2022.105992.
- Marinova-Petkova, A., J. Laplante, Y. Jang, B. Lynch, N. Zanders, M. Rodriguez, J. Jones, S. Thor, E. Hodges, J.A. De La Cruz, et al. 2017. Avian influenza A(H7N2) virus in human exposed to sick cats, New York, USA, 2016. Emerging Infect. Dis. 23 (12):12. doi:https://doi.org/10.3201/eid2312.170798.
- Marple, V.A., D.L. Roberts, F.J. Romay, N.C. Miller, K.G. Truman, M. van Oort, B. Olsson, M.J. Holroyd, J.P. Mitchell, and D. Hochrainer. 2003. Next generation pharmaceutical impactor (a new impactor for pharmaceutical inhaler testing). Part I: Design. J. Aerosol. Med. 16 (3):283–99. doi:10.1089/089426803769017659.
- Martin, E., N. Dziurowitz, U. Jäckel, and J. Schäfer. 2015. Detection of airborne bacteria in a duck production facility with two different personal air sampling devices for an exposure assessment. J. Occup. Environ. Hyg. 12 (2):77–86. doi:10.1080/15459624.2014.946514.
- Marvin, L.F., M.A. Roberts, and L.B. Fay. 2003. Matrix-assisted laser desorption/ionization time-of-flight mass spectrometry in clinical chemistry. Clin. Chim. Acta 337 (1–2):11–21. doi:10.1016/j.cccn.2003.08.008.
- Ma, J., Q. Xiao, H. Chen, L. Xinyue, Z. Zhang, H. Wang, L. Sun, L. Zhang, J. Guo, L. Morawska, et al. 2021. Coronavirus disease 2019 patients in earlier stages exhaled millions of severe acute respiratory syndrome coronavirus 2 per hour. Clin. Infect. Dis. 72 (10):e652–54. doi:10.1093/CID/CIAA1283.
- Mayor, S.D., S.M. Spuler, and B.M. Morley. 2005. Scanning eye-safe depolarization lidar at 1.54 microns and potential usefulness in bioaerosol plume detection, ed. Upendr.N. Singh, 58870N. doi:10.1117/12.620361
- Milton, D.K., M. Patricia Fabian, B.J. Cowling, M.L. Grantham, and J.J. McDevitt. 2013. Influenza virus aerosols in human exhaled breath: Particle size, culturability, and effect of surgical masks. PLoS Pathog. 9 (3):e1003205. doi:10.1371/journal.ppat.1003205.
- Misra, C., M. Singh, S. Shen, C. Sioutas, and P.M. Hall. 2002. Development and evaluation of a Personal Cascade Impactor Sampler (PCIS). J. Aerosol. Sci. 33 (7):1027–47. doi:10.1016/S0021-8502(02)00055-1.
- Morawska, L., G.R. Johnson, Z.D. Ristovski, M. Hargreaves, K. Mengersen, S. Corbett, C.Y.H. Chao, Y. Li, and D. Katoshevski. 2009. Size distribution and sites of origin of droplets expelled from the human respiratory tract during expiratory activities. J. Aerosol. Sci. 40 (3):256–69. doi:10.1016/J.JAEROSCI.2008.11.002.
- Morawska, L., and D.K. Milton. 2020. It is time to address airborne transmission of coronavirus disease 2019 (COVID-19). Clin. Infect. Dis. July. doi:10.1093/cid/ciaa939.
- Mouchtouri, V.A., M. Koureas, M. Kyritsi, A. Vontas, L. Kourentis, S. Sapounas, G. Rigakos, E. Petinaki, S. Tsiodras, and C. Hadjichristodoulou. 2020. Environmental contamination of SARS-CoV-2 on surfaces, air-conditioner and ventilation systems. Int. J. Hyg. Environ. Health 230 (September):113599. doi:10.1016/j.ijheh.2020.113599.
- Mubareka, S., N. Groulx, E. Savory, T. Cutts, S. Theriault, J.A. Scott, C.J. Roy, N. Turgeon, E. Bryce, G. Astrakianakis, et al. 2019. Bioaerosols and transmission, a diverse and growing community of practice. Front. Public Health 7 (February). doi: 10.3389/fpubh.2019.00023.
- Nagda, N.L., H.E. Rector, Z. Li, and D.R. Space. 2000. Aircraft cabin air quality: A critical review of past monitoring studies. In Air quality and comfort in airliner cabins, 215-215–25. 100 Barr Harbor Drive, PO Box C700, West Conshohocken, PA 19428-2959: ASTM International. doi:10.1520/STP14496S
- Nannu Shankar, S., C.T. Witanachchi, A.F. Morea, J.A. Lednicky, J.C. Loeb, M.M. Alam, Z. Hugh Fan, A. Eiguren-Fernandez, and W. Chang-Yu. 2022. SARS-CoV-2 in residential rooms of two self-isolating persons with COVID-19. J. Aerosol. Sci. 159 (January):105870. doi:10.1016/j.jaerosci.2021.105870.
- Nathu, V.D., J. Virkutyte, M.B. Rao, M. Nieto-Caballero, M. Hernandez, and T. Reponen. 2022. Direct-read fluorescence-based measurements of bioaerosol exposure in home healthcare. Int. J. Environ. Res. Public Health 19 (6):3613. doi:10.3390/ijerph19063613.
- Notomi, T., H. Okayama, H. Masubuchi, T. Yonekawa, K. Watanabe, N. Amino, and T. Hase. 2000. Loop-mediated isothermal amplification of DNA. Nucleic Acids Res. 28 (12):63e–663. doi:10.1093/nar/28.12.e63.
- O’Brien, K.M., and M.W. Nonnenmann. 2016. Airborne influenza a is detected in the personal breathing zone of swine veterinarians. PLoS ONE 11 (2):e0149083. doi:10.1371/journal.pone.0149083.
- Ong, S.W.X., Y. Kim Tan, P. Ying Chia, T. Hong Lee, N. Oon Tek, M. Su Yen Wong, and K. Marimuthu. 2020. Air, surface environmental, and personal protective equipment contamination by severe acute respiratory syndrome coronavirus 2 (SARS-CoV-2) from a symptomatic patient. JAMA 323 (16):1610. doi:10.1001/jama.2020.3227.
- O’Reilly, K.M., D.J. Allen, P. Fine, and H. Asghar. 2020. The challenges of informative wastewater sampling for SARS-CoV-2 must be met: Lessons from polio eradication. The Lancet Microbe 1 (5):e189–90. doi:10.1016/S2666-5247(20)30100-2.
- Otter, J.A., S. Yezli, J.A.G. Salkeld, and G.L. French. 2013. Evidence that contaminated surfaces contribute to the transmission of hospital pathogens and an overview of strategies to address contaminated surfaces in hospital settings. Am. J. Infect. Control 41 (5 SUPPL):S6. doi:10.1016/J.AJIC.2012.12.004.
- Pan, Y.L., K. Aptowicz, J. Arnold, S. Cheng, A. Kalume, P. Piedra, C. Wang, J.L. Santarpia, and G. Videen. 2022. Review of elastic light scattering from single aerosol particles and application in bioaerosol detection. J. Quant. Spectrosc. Radiat. Transf. 279 (March):108067. doi:10.1016/j.jqsrt.2022.108067.
- Pan, M., T.S. Bonny, J. Loeb, X. Jiang, J.A. Lednicky, A. Eiguren-Fernandez, Z.H.F. Susanne Hering, and W. Chang-Yu. 2017. Collection of viable aerosolized influenza virus and other respiratory viruses in a student health care center through water-based condensation growth. MSphere 2:5. doi:10.1128/mSphere.00251-17.
- Pan, M., L. Carol, J.A. Lednicky, A. Eiguren-Fernandez, Z.H.F. Susanne Hering, and W. Chang-Yu. 2018. Collection of airborne bacteria and yeast through water-based condensational growth. Aerobiologia 34 (3):337–48. doi:10.1007/s10453-018-9517-7.
- Pan, M., A. Eiguren‐fernandez, H. Hsieh, N. Afshar‐mohajer, S.V. Hering, J. Lednicky, Z. Hugh Fan, and C.Y. Wu. 2016. Efficient Collection of Viable Virus Aerosol through Laminar‐flow, Water‐based Condensational Particle Growth. J. Appl. Microbiol. 120 (3):805–15. doi:10.1111/jam.13051.
- Pan, Y.L., J. Hartings, R.G. Pinnick, S.C. Hill, J. Halverson, and R.K. Chang. 2003. Single-particle fluorescence spectrometer for ambient aerosols. Aerosol Sci. Technol. 37 (8):628–39. doi:10.1080/02786820300904.
- Pan, Y.-L., S.C. Hill, R.G. Pinnick, J.L. Santarpia, N. Baker, B. Alvarez, S. Ratnesar-Shumate, B. Cottrell, and L. McKee. 2011. “Fluorescence spectra of bioaerosols exposed to Ozone in a laboratory reaction chamber to simulate atmospheric processing.” In Proceedings of SPIE - The international society for optical engineering. Vol. 8018. doi:10.1117/12.883417.
- Pan, Y.-L., S.C. Hill, J.L. Santarpia, K. Brinkley, T. Sickler, M. Coleman, C. Williamson, K. Gurton, M. Felton, R.G. Pinnick, et al. 2014. Spectrally-resolved fluorescence cross sections of aerosolized biological live agents and simulants using five excitation wavelengths in a BSL-3 Laboratory. Opt. Express. 22 (7):8165–89. doi:10.1364/OE.22.008165.
- Pan, Y.L., S. Holler, R.K. Chang, S.C. Hill, R.G. Pinnick, S. Niles, and J.R. Bottiger. 1999. Single-shot fluorescence spectra of individual micrometer-sized bioaerosols illuminated by a 351- or a 266-Nm ultraviolet laser. Opt. Lett. 24 (2):116. doi:10.1364/OL.24.000116.
- Pan, Y.-L., J.L. Santarpia, S. Ratnesar-Shumate, E. Corson, J. Eshbaugh, S.C. Hill, C.C. Williamson, M. Coleman, C. Bare, and S. Kinahan. 2014. Effects of ozone and relative humidity on fluorescence spectra of octapeptide bioaerosol particles. J. Quant. Spectrosc. Radiat. Transf. 133:538–50. doi:10.1016/j.jqsrt.2013.09.017.
- Pan, Y.L., A. Kalume, J. Arnold, L. Beresnev, C. Wang, D.N. Rivera, K.K. Crown, and J.L. Santarpia. 2022. Measurement of Circular Intensity Differential Scattering (CIDS) from single airborne aerosol particles for bioaerosol detection and identification. Opt Express 30 (2):1442. doi:10.1364/OE.448288.
- Pan, Y.L., A. Kalume, C. Wang, and J.L. Santarpia. 2021. Atmospheric aging processes of bioaerosols under laboratory-controlled conditions: A review. J. Aerosol. Sci. 155 (June):105767. doi:10.1016/j.jaerosci.2021.105767.
- Pan, Y.L., R.G. Pinnick, S.C. Hill, J.M. Rosen, and R.K. Chang. 2007. Single-particle laser-induced-fluorescence spectra of biological and other organic-carbon aerosols in the atmosphere: Measurements at New Haven, Connecticut, and Las Cruces, New Mexico. J. Geophys. Res. 112 (D24):D24S19. doi:10.1029/2007JD008741.
- Park, G.W., P. Chhabra, and J. Vinjé. 2017. Swab sampling method for the detection of human norovirus on surfaces. JoVe 2017 (120):e55205. doi:10.3791/55205-v.
- Peak, N., C.W. Knapp, R.K. Yang, M.M. Hanfelt, M.S. Smith, D.S. Aga, and D.W. Graham. 2007. Abundance of six tetracycline resistance genes in wastewater lagoons at cattle feedlots with different antibiotic use strategies. Environ. Microbiol. 9 (1):143–51. doi:10.1111/j.1462-2920.2006.01123.x.
- Pinnick, R.G., S.C. Hill, P. Nachman, G. Videen, G. Chen, and R.K. Chang. 1998. Aerosol fluorescence spectrum analyzer for rapid measurement of single micrometer-sized airborne biological particles. Aerosol Sci. Technol. 28 (2):95–104. doi:10.1080/02786829808965514.
- Pinnick, R.G., S.C. Hill, Y.L. Pan, and R.K. Chang. 2004. Fluorescence spectra of atmospheric aerosol at Adelphi, Maryland, USA: Measurement and classification of single particles containing organic carbon. Atmos. Environ. 38 (11):1657–72. doi:10.1016/j.atmosenv.2003.11.017.
- Pochtovyi, A.A., V.V. Bacalin, N.A. Kuznetsova, M.A. Nikiforova, E.V. Shidlovskaya, B.I. Verdiev, E.N. Milashenko, A.M. Shchetinin, O.A. Burgasova, L.V. Kolobukhina, et al. 2021. SARS-CoV-2 aerosol and surface contamination in health care settings: The Moscow pilot study. Aerosol. Air Qual. Res. 21 (4):200604. doi:10.4209/aaqr.200604.
- Priyamvada, H., K. Kumaragama, A. Chrzan, C. Athukorala, S. Sur, and S. Dhaniyala. 2021. Design and evaluation of a new electrostatic precipitation-based portable low-cost sampler for bioaerosol monitoring. Aerosol Sci. Technol. 55 (1):24–36. doi:10.1080/02786826.2020.1812503.
- Prost, K., H. Kloeze, S. Mukhi, K. Bozek, Z. Poljak, and S. Mubareka. 2019. Bioaerosol and surface sampling for the surveillance of influenza a virus in swine. Transbound Emerg Dis 66:1210–17. doi:10.1111/tbed.13139.
- Rafiee, M., S. Isazadeh, A. Mohseni-Bandpei, S. Reza Mohebbi, M. Jahangiri-Rad, A. Eslami, H. Dabiri, K. Roostaei, M. Tanhaei, and F. Amereh. 2021. Moore swab performs equal to composite and outperforms grab sampling for SARS-CoV-2 monitoring in wastewater. Sci. Total Environ. 790 (October). doi: 10.1016/J.SCITOTENV.2021.148205.
- Ratnesar-Shumate, S., K. Bohannon, G. Williams, B. Holland, M. Krause, B. Green, D. Freeburger, and P. Dabisch. 2021. Comparison of the performance of aerosol sampling devices for measuring infectious SARS-CoV-2 aerosols. Aerosol. Sci. Technol. 55 (8):975–86. doi:10.1080/02786826.2021.1910137.
- Ratnesar-Shumate, S., Y.-L. Pan, S.C. Hill, S. Kinahan, E. Corson, J. Eshbaugh, and L.S. Joshua. 2015. Fluorescence spectra and biological activity of aerosolized bacillus spores and MS2 bacteriophage exposed to ozone at different relative humidities in a rotating drum. J. Quant. Spectrosc. Radiat. Transf. 153:13–28. doi:10.1016/j.jqsrt.2014.10.003.
- Ratnesar-Shumate, S., M.L. Wagner, C. Kerechanin, G. House, K.M. Brinkley, C. Bare, N.A. Baker, R. Quizon, J. Quizon, A. Proescher, et al. 2011. Improved method for the evaluation of real-time biological aerosol detection technologies. Aerosol. Sci. Technol. 45 (5):635–44. doi:https://doi.org/10.1080/02786826.2010.551144.
- Rehse, S.J. 2019. A review of the use of laser-induced breakdown spectroscopy for bacterial classification, quantification, and identification. Spectrochim Acta Part B 154 (April):50–69. doi:10.1016/j.sab.2019.02.005.
- Richardson, S.C., M. Mytilinaios, R. Foskinis, C. Kyrou, A. Papayannis, I. Pyrri, E. Giannoutsou, and I.D.S. Adamakis. 2019. Bioaerosol detection over Athens, Greece using the laser induced fluorescence technique. Sci. Total Environ. 696 (December):133906. doi:10.1016/j.scitotenv.2019.133906.
- Rodríguez, M., M. Llanos Palop, S. Seseña, and A. Rodríguez. 2021. Are the Portable Air Cleaners (PAC) really effective to terminate airborne SARS-CoV-2? Sci. Total Environ. 785 (September):147300. doi:10.1016/j.scitotenv.2021.147300.
- Roy, G. 2010. Lidar polarization discrimination of bioaerosols. Opt. Eng. 49 (11):116201. doi:10.1117/1.3505877.
- Russell, S.C., G. Czerwieniec, C. Lebrilla, H. Tobias, D.P. Fergenson, P. Steele, M. Pitesky, J. Horn, A. Srivastava, M. Frank, et al. 2004. Toward understanding the ionization of biomarkers from micrometer particles by bio-aerosol mass spectrometry. J. Am. Soc. Mass Spectrom. 15 (6):900–09. doi:10.1016/j.jasms.2004.02.013.
- Russo, G.B., T. Goyal, K. Tyler, and K.T. Thakur. 2022. Re‐emergence of poliovirus in the United States: Considerations and implications. Ann. Neurol. 92 (5):725–28. doi:10.1002/ana.26504.
- Saéz, M., S.W. Almudena, K. Nowak, V. Lapeyre, F. Zimmermann, A. Düx, H.S. Kühl, M. Kaba, S. Regnaut, K. Merkel, et al. 2015. Investigating the zoonotic origin of the West African Ebola epidemic. EMBO Mol. Med. 7 (1):17–23. doi:10.15252/emmm.201404792.
- Santander, M.V., B.A. Mitts, M.A. Pendergraft, J. Dinasquet, C. Lee, A.N. Moore, L.B. Cancelada, K.A. Kimble, F. Malfatti, and K.A. Prather. 2021. Tandem fluorescence measurements of organic matter and bacteria released in sea spray aerosols. Environ. Sci. Technol. 55 (8):5171–79. doi:10.1021/acs.est.0c05493.
- Santarpia, J.L. 2016. Bioaerosols in the environment: Populations, measurement and processes. Issues Toxicol. Vol. 2016 Janua. doi:10.1039/9781849737913-00219.
- Santarpia, J.L., D.R. Collins, S.A. Ratnesar-Shumate, C.C. Glen, A.L. Sanchez, C.G. Antonietti, J. Taylor, N.F. Taylor, C.A. Bare, S.M. Kinahan, et al. 2022. Changes in the fluorescence of biological particles exposed to environmental conditions in the national capitol region. Atmosphere. 13 (9):1358. doi:10.3390/atmos13091358.
- Santarpia, J.L., V.L. Herrera, D.N. Rivera, S. Ratnesar-Shumate, S. Patrick Reid, D.N. Ackerman, P.W. Denton, J.W.S. Martens, Y. Fang, N. Conoan, et al. 2022. The size and culturability of patient-generated SARS-CoV-2 aerosol. J. Expo. Sci. Environ. Epidemiol. 32 (5):706–11. doi:10.1038/s41370-021-00376-8.
- Santarpia, J.L., Y.-L. Pan, S.C. Hill, N. Baker, B. Cottrell, L. McKee, S. Ratnesar-Shumate, and R.G. Pinnick. 2012. Changes in fluorescence spectra of bioaerosols exposed to ozone in a laboratory reaction chamber to simulate atmospheric aging. Opt. Express. 20 (28):29867–81. doi:10.1364/OE.20.029867.
- Santarpia, J.L., S. Ratnesar-Shumate, J.U. Gilberry, and J.J. Quizon. 2013. Relationship between biologically fluorescent aerosol and local meteorological conditions. Aerosol Sci. Technol. 47 (6):655–61. doi:10.1080/02786826.2013.781263.
- Santarpia, J.L., D.N. Rivera, V.L. Herrera, M. Jane Morwitzer, H.M. Creager, G.W. Santarpia, K.K. Crown, D.M. Brett-Major, E.R. Schnaubelt, M.J. Broadhurst, et al. 2020. Aerosol and surface contamination of SARS-CoV-2 observed in quarantine and isolation care. Sci Rep. 10 (1):12732. doi:10.1038/s41598-020-69286-3.
- Šantl-Temkiv, T., B. Sikoparija, T. Maki, F. Carotenuto, P. Amato, M. Yao, C.E. Morris, R. Schnell, R. Jaenicke, C. Pöhlker, et al. 2020. Bioaerosol field measurements: Challenges and perspectives in outdoor studies. Aerosol Sci. Technol. 54 (5):520–46. doi:10.1080/02786826.2019.1676395.
- Savage, N.J., C.E. Krentz, T. Könemann, T.T. Han, G. Mainelis, C. Pöhlker, and J. Alex Huffman. 2017. Systematic characterization and fluorescence threshold strategies for the Wideband Integrated Bioaerosol Sensor (WIBS) using size-resolved biological and interfering particles. Atmos. Meas. Tech. 10 (11):4279–302. doi:10.5194/amt-10-4279-2017.
- Scherer, K., A.D. Mäde, A.L. Ellerbroek, A.J. Schulenburg, A.R. Johne, and A.G. Klein. 2009. Application of a swab sampling method for the detection of norovirus and rotavirus on artificially contaminated food and environmental surfaces. Food Environ. Virol. 1 (1):42–49. doi:https://doi.org/10.1007/s12560-008-9007-0.
- Schmidt, M.S., and A.J. Ray Bauer. 2010. Preliminary correlations of feature strength in spark-induced breakdown spectroscopy of bioaerosols with concentrations measured in laboratory analyses. Appl. Opt. 49 (13):C101. doi:10.1364/AO.49.00C101.
- Sehulster, L.M., R.Y.W. Chinn, M.J. Arduino, J. Carpenter, R. Donlan, D. Ashford, R. Besser. 2004. Guidelines for environmental infection control in health-care facilities. Recommendations from CDC and the Healthcare Infection Control Practices Advisory Committee (HICPAC). Chicago IL. https://www.cdc.gov/infectioncontrol/guidelines/environmental/index.html
- Sengupta, A., M.L. Laucks, N. Dildine, E. Drapala, and E.J. Davis. 2005. Bioaerosol characterization by Surface-Enhanced Raman Spectroscopy (SERS). J. Aerosol. Sci. 36 (5–6):651–64. doi:10.1016/j.jaerosci.2004.11.001.
- Shammi, M., M. Mostafizur Rahman, and S. Mohammad Tareq. 2021. Distribution of bioaerosols in association with particulate matter: A review on emerging public health threat in Asian Megacities. Front. Environ. Sci. 9 (August). doi: 10.3389/fenvs.2021.698215.
- Shannon, K.E., D.Y. Lee, J.T. Trevors, and L.A. Beaudette. 2007. Application of real-time quantitative PCR for the detection of selected bacterial pathogens during municipal wastewater treatment. Sci. Total Environ. 382 (1):121–29. doi:10.1016/J.SCITOTENV.2007.02.039.
- Shapiro, D.B., M.F. Maestre, W.M. McClain, P.G. Hull, Y. Shi, M.S. Quinby-Hunt, J.E. Hearst, and A.J. Hunt. 1994. Determination of the average orientation of DNA in the octopus sperm Eledone cirrhossa through polarized light scattering. Appl. Opt. 33 (24):5733. doi:10.1364/AO.33.005733.
- Sharma, R., R. Ranjan, R. Kishor Kapardar, and A. Grover. 2005. ‘Unculturable’ bacterial diversity: An untapped resource. Curr. Sci. 89 (1): 72–77.
- Shoshanim, O., and A. Baratz. 2020. Daytime measurements of bioaerosol simulants using a hyperspectral laser-induced fluorescence LIDAR for biosphere research. J. Environ. Chem. Eng. 8 (5):104392. doi:10.1016/j.jece.2020.104392.
- Silvestri, E., Y. Chambers-Velarde, J. Chandler, J. Cuddeback, W. Calfee, J. Archer, and S. Shah. 2021. Collection of surface samples potentially contaminated with microbiological agents using swabs, sponge sticks and wipes. Washington, DC. https://cfpub.epa.gov/si/si_public_record_report.cfm?dirEntryId=352038&Lab=CESER.
- Singh, M., C. Misra, and C. Sioutas. 2003. Field evaluation of a Personal Cascade Impactor Sampler (PCIS). Atmos. Environ. 37 (34):4781–93. doi:10.1016/j.atmosenv.2003.08.013.
- Sinha, M.P., R.M. Platz, V.L. Vilker, and S.K. Friedlander. 1984. Analysis of individual biological particles by mass spectrometry. Int. J. Mass Spectrom. Ion Process. 57 (1):125–33. doi:10.1016/0168-1176(84)85070-3.
- Sivaprakasam, V., A.L. Huston, C. Scotto, and J.D. Eversole. 2004. Multiple UV wavelength excitation and fluorescence of bioaerosols. Opt. Express 12 (19):4457. doi:10.1364/OPEX.12.004457.
- Sivaprakasam, V., H.B. Lin, A.L. Huston, and J.D. Eversole. 2011. Spectral characterization of biological aerosol particles using two-wavelength excited laser-induced fluorescence and elastic scattering measurements. Opt. Express 19 (7):6191. doi:10.1364/OE.19.006191.
- Sloot, P.M.A., A.G. Hoekstra, H. van der Liet, and C.G. Figdor. 1989. Scattering matrix elements of biological particles measured in a flow through system: Theory and practice. Appl. Opt. 28 (10):1752. doi:10.1364/AO.28.001752.
- Stowers, M.A., A.L. van Wuijckhuijse, J.C.M. Marijnissen, C.E. Kientz, and T. Ciach. 2006. Fluorescence preselection of bioaerosol for single-particle mass spectrometry. Appl. Opt. 45 (33):8531. doi:10.1364/AO.45.008531.
- Su, C., J. Lau, and Y. Fang. 2017. A case study of upper-room UVGI in densely-occupied elementary classrooms by real-time fluorescent bioaerosol measurements. Int. J. Environ. Res. Public Health 14 (1):51. doi:10.3390/ijerph14010051.
- Taku, A., B.R. Gulati, P.B. Allwood, K. Palazzi, C.W. Hedberg, and S.M. Goyal. 2002. Concentration and detection of caliciviruses from food contact surfaces. J. Food Prot. 65 (6):999–1004. http://meridian.allenpress.com/jfp/article-pdf/65/6/999/1672215/0362-028x-65_6_999.pdf.
- Tanaka, N. 2021. Real-time monitoring of bioaerosol in a residential property in Central Tokyo. Asian J. Atmos. Environ. 15 (3):45–55. doi:10.5572/ajae.2021.055.
- Tang, S., Y. Mao, R.M. Jones, Q. Tan, J.S. Ji, N. Li, J. Shen, Y. Lv, L. Pan, P. Ding, et al. 2020. Aerosol transmission of SARS-CoV-2? Evidence, prevention and control. Environ Int. 144 (November):106039. doi:10.1016/j.envint.2020.106039.
- Therkorn, J., D. Drewry III, T. Pilholski, K. Shaw‐saliba, G. Bova, L.L. Maragakis, B. Garibaldi, and L. Sauer. 2019. Impact of air‐handling system exhaust failure on dissemination pattern of simulant pathogen particles in a clinical biocontainment unit. Indoor Air 29 (1):143–55. doi:10.1111/ina.12506.
- Therkorn, J., N. Thomas, J. Scheinbeim, and G. Mainelis. 2017. Field performance of a novel passive bioaerosol sampler using polarized ferroelectric polymer films. Aerosol Sci. Technol. 51 (7):787–800. doi:10.1080/02786826.2017.1316830.
- Tobias, H.J., M.P. Schafer, M. Pitesky, D.P. Fergenson, J. Horn, M. Frank, and E.E. Gard. 2005. Bioaerosol mass spectrometry for rapid detection of individual airborne Mycobacterium Tuberculosis H37Ra particles. Appl. Environ. Microbiol. 71 (10):6086–95. doi:10.1128/AEM.71.10.6086-6095.2005.
- Toprak, E., and M. Schnaiter. 2013. Fluorescent biological aerosol particles measured with the waveband integrated bioaerosol sensor WIBS-4: Laboratory tests combined with a one year field study. Atmos. Chem. Phys. 13 (1):225–43. doi:10.5194/acp-13-225-2013.
- Tripathi, A., R.E. Jabbour, J.A. Guicheteau, S.D. Christesen, D.K. Emge, A.W. Fountain, J.R. Bottiger, E.D. Emmons, and A. Peter Snyder. 2009. Bioaerosol analysis with raman chemical imaging microspectroscopy. Anal. Chem. 81 (16):6981–90. doi:10.1021/ac901074c.
- Ugarte-Ruiz, M., D. Florez-Cuadrado, T.M. Wassenaar, M. Concepción Porrero, and L. Domínguez. 2015. Method comparison for enhanced recovery, isolation and qualitative detection of C. Jejuni and C. Coli from Wastewater Effluent Samples. Int. J. Environ. Res. Public Health 12:2749–64. doi:10.3390/ijerph120302749.
- U.S. Environmental Protection Agency. Region VII. 1974. Wastewater sampling methodologies and flow measurement techniques - United States. Environ. Prot. Agency Region VII. - Google Books. https://books.google.com/books?hl=en&lr=&id=SF3L6PsBGRcC&oi=fnd&pg=PR7&dq=wastewater+sampling&ots=FklsBFP0c1&sig=85RlGsLvr47zLV7CXXXydrqrnT8#v=onepage&q=wastewater%20sampling&f=false
- van Wuijckhuijse, A.L., M.A. Stowers, W.A. Kleefsman, B.L.M. van Baar, C.E. Kientz, and J.C.M. Marijnissen. 2005. Matrix-assisted laser desorption/ionisation aerosol time-of-flight mass spectrometry for the analysis of bioaerosols: Development of a fast detector for airborne biological pathogens. J. Aerosol. Sci. 36 (5–6):677–87. doi:10.1016/j.jaerosci.2004.11.003.
- Walls, H.J., D.S. Ensor, L.A. Harvey, J.H. Kim, R.T. Chartier, S.V. Hering, S.R. Spielman, and G.S. Lewis. 2016. Generation and sampling of nanoscale infectious viral aerosols. Aerosol Sci. Technol. 50 (8):802–11. doi:10.1080/02786826.2016.1191617.
- Wang, C., Y.L. Pan, S.C. Hill, and B. Redding. 2015. Photophoretic trapping-raman spectroscopy for single pollens and fungal spores trapped in air. J. Quant. Spectrosc. Radiat. Transf. 153 (March):4–12. doi:10.1016/j.jqsrt.2014.11.004.
- Wastewater-Based Disease Surveillance for Public Health Action. 2023. Washington, D.C: National Academies Press. doi:10.17226/26767.
- World Health Organization (WHO). 2020. Surface sampling of coronavirus disease (COVID-19): A practical ‘how to’ protocol for health care and public health professionals.
- The World Organisation for Animal Health. 2022. Terrestrial animal health code: Chapter 1.4. Anim. Health Surv. 1:1–10.
- Wu, F., A. Xiao, J. Zhang, K. Moniz, N. Endo, F. Armas, M. Bushman. 2021. Wastewater surveillance of SARS-CoV-2 across 40 U.S. States from February to June 2020. Water Res. 202 (September):117400. doi:10.1016/j.watres.2021.117400.
- Yamagishi, T., M. Ohnishi, N. Matsunaga, K. Kakimoto, H. Kamiya, K. Okamoto, M. Suzuki, Y. Gu, M. Sakaguchi, T. Tajima, et al. 2020. Environmental sampling for severe acute respiratory syndrome coronavirus 2 during a COVID-19 outbreak on the diamond princess cruise ship. Brief Rep. J. Infect. Dis.® 2020:222. doi:10.1093/infdis/jiaa437.
- Yang, S., G. Bekö, P. Wargocki, J. Williams, and D. Licina. 2021. Human emissions of size-resolved fluorescent aerosol particles: Influence of personal and environmental factors. Environ. Sci. Technol. 55 (1):509–18. doi:10.1021/acs.est.0c06304.
- Yin, L., S. Man, Y. Shengying, G. Liu, and M. Long. 2021. CRISPR-Cas based virus detection: Recent advances and perspectives. Biosens. Bioelectron. 193 (December):113541. doi:10.1016/j.bios.2021.113541.