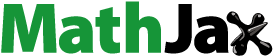
ABSTRACT
The present study was carried out to optimize the physical and nutritional parameters for cultivation of Trichoderma harzianum Rifai for production of extracellular β-glucosidase, an alternative to chemcial fungicides to control Macrophomina phaseolina, which causes charcoal rot disease in crops. Response surface methodology by the Box–Behnken design (BBD) based on four factors (temperature, carbon sources, inoculum size and pH) was used for optimization of the cultivation conditions to produce β-glucosidase using agro-industrial banana wastes. The highest β-glucosidase activity (1483.27 U/mL) was attained under optimized cultivation conditions: ∼36 ºC and pH 7.3, using carbon source and inoculum size of 10% (w/v) and 5% (w/v), respectively. It is noteworthy that the low deviation values (0.09−0.44%) in the verification experiments (R1, R2 and R3) inferred the generated model was accurate to predict optimal cultivation conditions of T. harzianum Rifai. Likewise, the obtained diameters of inhibition zones ranging from 58.00−38.66 mm following treatment with β-glucosidase was found comparable to other in vitro tests using pure T. harzianum isolates and chemical fungicides. Hence, the findings indicated that it was feasible to use β-glucosidase as a greener alternative to chemical fungicides for control of M. phaseolina infection and consequently, for protection of crops against charcoal rot disease.
Introduction
Macrophomina phaseolina (Tassi) Goid is a ubiquitous fungal phytopathogen that causes charcoal rot in more than 500 plant species belonging to over 100 families of monocots and dicots, affecting plant hosts viz. cereals, legumes, oilseed plants as well as some vegetables and fruits [Citation1]. To eradicate this debilitating plant disease, many farmers have resorted to using chemical fungicides but the technique poses other problems, e.g. toxicity to living beings and the environment as well as economic infeasibility especially for low-income small-scale farmers. Although the use of chemical fungicides may be effective as a preventive measure [Citation2], the soil-borne nature of M. phaseolina [Citation3] renders the effort futile. Aside to being expensive, the continuous utilization of chemical fungicides could interfere with the delicate balance of beneficial soil microbes [Citation4], hence giving rise to new fungicide resistant strains [Citation5].
The antagonistic efficacy of Trichoderma harzianum against M. phaseolina used for combating M. phaseolina infections has been widely described [Citation3,Citation5]. T. harzianum is one of several antagonistic fungal species found effective against this phytopathogen [Citation5,Citation6], some of which enhance the systemic resistance and improve overall plant growth [Citation7]. Apart from the well-known biocontrol mechanisms, viz. nutrient competition and antibiotic production [Citation8], T. harzianum also produces hyphae that penetrate into M. phaseolina and subsequently secrete an enzyme called β-glucosidase that digests the cell wall and kills the host [Citation9].
Interestingly, the direct use of β-glucosidase for agricultural pest management to control M. phaseolina infestation has never been considered and remains unreported. It is known that the β-glucosidase secreted by Trichoderma spp. is evolutionarily adapted to specifically hydrolyze the cell wall components of M. phaseolina. This β-specific glucosidase enzyme targets and explicitly hydrolyzes the β-linkage of amorphic β-1,3-glucan filling material of the chitin-based cell wall of M. phaseolina [Citation10]. By such virtue, it was hypothesized that direct application of the extracellular β-glucosidase to inhibit the growth of this phytopathogen could be likely feasible. Considering the extraordinary adaptability of M. phaseolina and the need to alleviate the dependency of the agricultural sector on harmful chemical fungicides, evaluation of the efficacy of extracellular β-glucosidase to control the growth of the phytopathogen via such approach deserves scientific and agricultural consideration. Moreover, the method suggested here may present a more expedient pest management alternative as well as may minimize the undesirable impacts on the environment associated with such practices.
The strategy proposed here that could improve the process production of β-glucosidase is the use of agro-industrial wastes, which decreases the initial costs of raw materials [Citation11]. To date, a number of agro-industrial wastes explored as substrate in laboratory-scale culture media include whey [Citation12], molasses [Citation13], rice straw [Citation14] and okara with sugarcane bagasse [Citation15]. Another crucial aspect is the optimization of the fungal cultivation media to produce β-glucosidase. This is because the conventional approach of optimization processes is laborious due to screening processes that involve a large number of variables [Citation16,Citation17]. Moreover, the approach is incapable of detecting the true optimum [Citation18] following its one-factor-at-a-time design, which is unable to explicitly illustrate the complete interactive effects of various factors on the response [Citation19]. Hence, response surface methodology (RSM) is one of the most efficient optimization strategies to mathematically and statistically optimize and identify the optimum operating conditions for the system [Citation11]. In fact, it has been proved beneficial and successful in optimizing the cultivation conditions of fungal cultures [Citation20–22].
This work was aimed at development and optimization of an economical medium for β-glucosidase production in batch cultures of T. harzianum Rifai based on an agro-industrial substrate, i.e. banana wastes. The optimization process uses a 3-level-4-factor Box–Behnken design (BBD) to evaluate four factors that comprise temperature, carbon sources, inoculum size and pH to provide for the highest β-glucosidase activity. Additionally, verification experiments using plate inhibition assays to confirm the efficacy of the produced β-glucosidase to inhibit the growth of M. phaseolina are also presented.
Materials and methods
Collection of T. harzianum T12 and growth conditions of fungal cultures
The colonies of T. harzianum (T12) fungal antagonists were isolated from agricultural soil samples collected from the Mazandaran province, Iran. The antagonistic efficacy of the T12 isolate utilized in this study was reported in our previous work [Citation5]. Following the method described in [Citation23], the pure culture of T. harzianum T12 was grown as detailed below. Potato dextrose agar (PDA; Oxoid Ltd, Basingstoke, England) slants were incubated in darkness at 30 ˚C for 5 days and subsequently stored at 4 ˚C prior to use. For culture growth, a 5-day old culture of T12 grown in a sterile potato dextrose broth (PDB; Oxoid Ltd, England) was inoculated into an Erlenmeyer flask containing 30 mL of sterile PDB as a carbon source. The mixture was incubated at 30 ˚C ± 1 ˚C for 5 days under stationary conditions for development of the fungal spore suspension [Citation23].
Sporulation medium and inoculum development
Sporulation medium (composition (w/v): Trisodium citrate, 0.5%; KH2PO4, 0.5%; NH4NO3, 0.2%; (NH4)2 SO4, 0.4%; MgSO4, 0.02%; peptone, 0.1%; yeast extract, 0.2%; flucose, 2%; agar, 2.5%) was prepared and its pH was adjusted to 5.5 prior to autoclaving for 15 min at 121 ˚C. Fungal culture was transferred to sporulation slants under sterile conditions and incubated for 48 h at 35 ˚C for sporulation and subsequently stored at 4 ˚C. The inoculum medium (100 mL) was prepared according to the following composition (w/v): trisodium citrate, 0.5%; KH2PO4, 0.5%; NH4NO3, 0.2%; (NH4)2 SO4, 0.4%; MgSO4, 0.02%; peptone, 0.1%; yeast extract, 0.2%; glucose, 2%; in 500 mL Erlenmeyer flasks containing glass beads for uniform growth. The inoculum medium was adjusted to pH 5.5 prior to autoclaving for 15 min at 121˚C. A loopful of T. harzianum T12 culture from the sporulation medium was transferred aseptically into the flask and incubated on an orbital shaker (120 r/min) for 24 h at 30 ˚C [Citation24].
Production and extraction of β-glucosidase
In this study, banana waste was used to stimulate production of β-glucosidase in the broth cultures of T. harzianum Rifai. Banana waste of approximately 10 g was mixed with 2% HCl at room temperature in an Erlenmeyer flask for 2 h, autoclaved (15 min at a pressure of 15 psi and 121˚C) and the resultant slurry was filtered through a Whatman No. 1 filter paper (Whatman® Grade 1 Qualitative Filtration Paper, Sigma plot) and used as the fungal growth medium. Next, nutrient salt (NH4)2SO4, 3.0 g/L; FeSO4·7H2O, 0.005 g/L; KH2PO4, 1.0 g/L; MnSO4·H2O, 0.0016 g/L; MgSO4·7H2O, 0.5 g/L; ZnSO4·7H2O, 0.0017 g/L; CaCl2, 0.1 g/L; CoCl2, 0.002 g/L and NaCl, 0.1 g/L, was used to moisten the banana waste (10 g) prior to inoculation with the freshly prepared T. harzianum T12 spore suspension (5 mL, 5%, w/v) and incubated at 30 ˚C for 24 h [Citation25]. For the β-glucosidase extraction process, sodium citrate buffer (0.05 mol/L, pH 4.8) in a 1:10 ratio was added into the culture medium and the flask was shaken at 120 r/min for 30 min before filtering through a Muslin cloth. The filtrate was centrifuged for 10 min (10,000 g) and the supernatant was tested for β-glucosidase activity [Citation26].
Experimental design and optimization by RSM using BBD and statistical analysis
RSM was conducted using the software Design Expert Version 7.1.2 (State Ease, Statistical Made Easy, MN, USA). It was used to fit the second-order model to the independent variables using the following equation:Where Y (β-glucosidase activity (U/mL)) is the predicted response;
and
are the linear and quadratic coefficients, respectively;
and
are the uncoded independent variables; k is the number of studied factors optimized in the experiment;
is a constant coefficient;
,
and
are the interaction coefficients of linear, quadratic and second-order terms, respectively; and
is the error.
The Box–Behnken experimental design employed in this study required a total of 29 reactions to study the response pattern and to determine the optimum combination of variables. The interaction of parameters such as, A (reaction temperature), B (carbon sources), C (inoculum size) and D (pH) at three levels in the reaction process were evaluated and the percentage conversion of β-glucosidase was the response of the experimental design. The independent variables and their levels, actual values as well as coded values are presented in . The model equation was used to estimate the optimum value and subsequently to describe the interaction between the factors.
Table 1. Actual and coded independent variables in the BBD for the optimization of β-glucosidase activity in batch cultures of T. harzianum.
Statistical analysis of variance (ANOVA) was used to assess the adequacy of the generated model to describe the data and to express the quality of the fit of the polynomial model. The significance of the model was considered to be represented by a p-value of less than 0.05. The analysis included the coefficient of determination, R2, which evaluated the fitness of the model. Three-dimensional surface and contour plots were generated to assess the major and interactive effects between the dependent and independent factors. Consequently, the optimum combination of parameters was established using the optimization function of the software, based on the ridge maximum and canonical analyses.
Determination of β-glucosidase activity and protein content
The β-glucosidase activity was examined using p-nitrophenyl-β-D-glucopyranoside (pNPG, SigmaPlot) as a substrate in a microtiter plate as described in [Citation27] with some modifications. A 100-μL reaction mixture (25 μL of enzyme, 25 μL of pNPG (10 mmol/L) and 50 μL of phosphate buffer (pH 7.0)) was incubated at 45 ˚C for 30 min and the reaction was terminated by adding 100 μL of NaOH-glycine buffer (0.4 mol/L, pH 10.8). The colour developed due to liberation of p-nitrophenol (pNP) was read at 412 nm in an ELISA Reader (MULTISKAN™; Labsystems, Waltham, Massachusetts, USA) absorbance spectrometer. The concentration of liberated pNP was calculated by comparing the reading corrected for blanks against a pNP standard calibration curve. One unit (U) of β-glucosidase activity was defined as the amount of enzyme needed to liberate 1 μmol/L of pNP per minute under standard assay conditions. The experiments were repeated three times from independently grown cultures.
Results and discussion
Fitting of the response models
The optimization of process parameters can be quite complex; hence the use of RSM for identifying optimum levels of selected variables is often considered useful [Citation28] for enabling estimation of the optimum levels of process parameters [Citation24]. Our preliminary experiments showed that the factors that most influenced the production of β-galactosidase by T. harzianum were reaction temperature, carbon source, inoculum size and pH (data not shown). In this perspective, a BBD experiment using levels of factors previously determined by screening experiments to maximize the production and activity of the fungal β-galactosidase was employed. The selected model, which consisted of three levels (‒1, 0, +1), was evaluated using various statistical analysis parameters, viz. P-value, F-value, adjusted determination of coefficient (R2adj), which measures the signal-to-noise ratio, and the coefficient of determination (R2) to assess the goodness-of-fit of the developed quadratic mathematical model to the experimental data.
Fitting of the data to various models (linear, two-factorial, quadratic and cubic) and subsequent ANOVA revealed that the β-glucosidase activity was satisfactorily described with a quadratic polynomial model. The quality of fitting to the second-order polynomial models to justify its robustness was confirmed by the attained high values for the coefficient of determination obtained for β-glucosidase activity (R2 = 0.9491) (). A high R2 values signifies an excellent correlation [Citation29], specifically a value of R2 > 0.9 [Citation30]. Likewise, the values of adjusted coefficients of determination for enzyme activity (R2adj = 0.8983) also closely agreed with the obtained R2. The signal-to-noise ratio, (Adequate precision = 13) representing the precision of the model was desirable and could accurately predict the variation. A high value of adequate precision is preferred, as it indicates the reliability and precision of the experimental responses. Comparison between the actual and predicted values obtained in this study revealed that the values were in close agreement, as many of the experimental data points were closely scattered near the trend line ().
Table 2. Polynomial equation for the estimated coded and processed factors for β-glucosidase activity used in BBD.
Influence of experimental factors on β-glucosidase activity
The regression quadratic polynomial model for enzyme activity and the terms for coded factors are presented in . It can be seen that the factors A, B, C, D (independent) as well as AC, BC and BD (interaction) had preceding positive signs, whereas AB, AD (interaction) as well as A2 , B2, C2 and D2 (quadratic) were negative. The positive and negative signs preceding the terms indicated that these terms affected, respectively, synergistically or antagonistically the activity of β-glucosidase produced by T. harzianum. The response factor obtained in this study was the measured values in the experimental results. Pertinently, the data from the experimental results were used for generating coefficients for the quadratic polynomial equations and subsequently, for predicting the enzyme activity in the batch cultures of T. harzianum grown in banana wastes (). The actual and predicted enzyme activity in coded and actual terms obtained from the experimental and regression model are presented in .
Table 3. Compositions of the various runs of BBD in coded and actual terms for the obtained actual and predicted responses for optimizing β-glucosidase activity in batch cultures of T. harzianum.
ANOVA was used for evaluation of the statistical significance of the model as well as for individual model terms, whereby the influence of four independent factors on the enzyme activity was described through the significant coefficient ( p < 0.05) of the second-order polynomial regression equation. In all cases, a large F-value and a small P-value would imply a significant effect of the respective response factors. According to the ANOVA of factors, the model was decidedly significant due to its high F value (18.66) and very small p-value ( p < 0.0001) (). The F-value of the model (18.66) was found higher than the tabular F0.05 (14,14) = 2.48, indicating that the model was significant at the 5% confidence level. The lack of fit (F-value of 5.53) was lower than the tabulated F0.05(10,4) = 5.964, which implied that the lack of fit was insignificant in relevance to the pure error. The linear, quadratic and interaction terms were found significant (P < 0.05) in describing the production of highly active β-glucosidase, except for the interactions of carbon sources and inoculum size, BC, as well as inoculum size and pH (P > 0.05) ().
Table 4. ANOVA for the second-order polynomial model of BBD.
Table 5. ANOVA for the second order polynomial models and coefficient values for β-glucosidase activity obtained from the growth culture supernatant.
The linear term of temperature, A, and quadratic terms A2 and B2 were highly significant (P < 0.001) in influencing the β-glucosidase activity in the T. harzianum batch cultures. Although the interaction between temperature and carbon source was significant (P = 0.0279), the linear effect of temperature (F = 18.30) was more significant than the proportion of carbon source (F = 6.31) (). The interaction between incubation temperature and amount of carbon source used in the culture was found to be antagonistic (−54.58AB) (); hence, increasing both factors simultaneously may not improve the β-glucosidase activity. Likewise, the term that represented the interactions between the carbon source and pH of the culture medium, BD, as well as the corresponding linear terms were also shown to have a significant effect (P = 0.0399). Their quadratic terms were found highly significant () and a preceding positive term (+142.75BD) signified their interaction would synergistically improve the β-glucosidase activity in the batch cultures of T. harzianum.
Evaluation of the interactive effects of temperature and inoculum size, AC, on β-glucosidase activity revealed that the term was highly significant (P = 0.0051). By considering the coefficient values obtained for the corresponding linear, interactive and quadratic terms, both the linear terms (temperature and inoculum size) were found to most significantly influence the activity of β-glucosidase (F > 18.0). Correspondingly, the interaction and quadratic terms of both factors were also highly significant (P < 0.001) (). With a positive interaction term (+208.94AC) (), increasing both factors could beneficially maximize the β-glucosidase activity.
Consequently, the interactive effects for the factors temperature and pH, AD (P = 0.0226), as well as the linear term for pH were also significant, whereas the quadratic terms, A2 (P = 0.0003) and B2 (P < 0.0001) were found highly significant (). However, the preceding negative sign in the model equation (−161.43AD) () indicated that their interactions for attaining high β-glucosidase activity were inversely correlated. On the contrary, the interactive factors for carbon sources and inoculum size, BC, as well as for inoculum size and pH, CD, were found non-significant and, hence, will not be further discussed in this report. Both contour and surface plots were used to illustrate the interactions between the tested variables and the resulting responses between factors, for better comprehension and depiction of the interactive effects.
Effect of temperature and carbon source
Previous studies have described that culture conditions such as temperature and the amount of carbon source used in the cultivation media could exert significant influence on β-glucosidase activity [Citation31,Citation32]. For cultivation of T. harzianum to obtain β-glucosidase, the activity of the enzyme depends on the nature of the carbon source used in the batch culture. This is because the supplied carbon source forms the major constituent for building cellular material as well as the source of energy [Citation33,Citation34].
The response surface and contour plots representing the interactive effect of temperature and carbon source, AB, on the activity of β-glucosidase are shown in with the inoculum size and pH held at their central values of 5% (w/v) and pH 7, respectively. The linear term for temperature (F = 18.30) had a larger influence on the β-glucosidase activity than that for the carbon source (F = 6.31). The elliptical contour plot seen here corroborated the significance of both interacting variables in yielding high β-glucosidase activity. The activity of β-glucosidase was observed to increase up to a certain extent with the increasing of both growth temperature and percentage of carbon sources. The maximum activity of 1208 U/mL was predicted by the model under conditions of 10% (w/v) carbon source and growth temperature of 36 oC, whereas further increment beyond these maximum points was futile and rendered a gradual decrease in β-glucosidase activity.
Figure 2. Response surface (a) and contour (b) plots showing the interactive effect of A: temperature and B: carbon source on the activity of β-glucosidase.
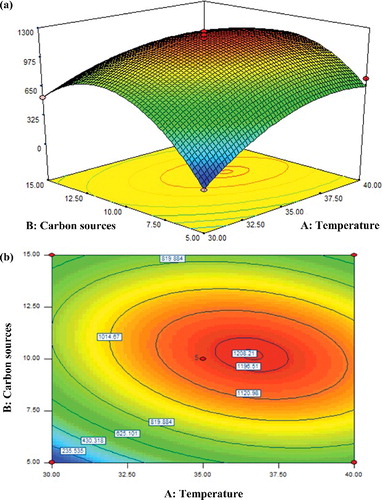
These results showed that the optimum growth temperature of 36 oC for achieving high β-glucosidase activity is typical for T. harzianum, consistent with previous findings [Citation35,Citation36]. It is known that β-glucosidase activity tends to vary under different culture temperatures and is strain dependent as well [Citation37]. Likewise, physiological analysis of the effect of various carbon sources on enzyme activity is also important. In this study, the results suggested strong correlation between the production of β-glucosidase and the amount of carbon source, i.e. banana waste, used in the batch culture media. In this study, banana wastes were selected as the source of carbon for growth of T. harzianum, so as to ensure considerably lower cost to obtain the enzyme. As a matter of fact, the use of agro-based biomass such as the low-cost banana wastes as a carbon source in fungal batch cultures may prove to be an interesting as well as useful substitute to the more expensive carbon sources. The results obtained here invariably indicated the feasibility in using banana waste as the carbon source to produce β-glucosidase showing high activity in batch cultures of T. harzianum. This could be mainly due to the banana wastes being rich in nutrients such as cellulose; hence, sufficient to stimulate growth and enhance β-glucosidase production in the fungal culture. The results from this study support the report by Reddy and Yang [Citation38], in which the pseudostem and leaves of banana wastes had exceptionally high cellulose content and served as excellent substrates for cultivation of T. harzianum.
Effect of temperature and inoculum size
As indicated in [Citation37], the maximum production of β-glucosidase would be supported by a suitable growth temperature and inoculum size. Hence, the interactive effects of temperature and inoculum size, AC, on the activity of β-glucosidase were evaluated, while the percentage of carbon sources and pH were maintained at their central values of 10% (w/v) and pH 7, respectively (). The linear terms for temperature (F = 18.30) and inoculum size (18.90) were found almost comparable in yielding high β-glucosidase activity. The activity of β-glucosidase progressively reached its maximum at 1246 U/mL, when the inoculum size increased to 6% (w/v) and the temperature, to 50 °C, before a decline was observed. Judging from the nearly perfectly centered elliptical plots, it was clear that both factors, inoculum size and temperature, were crucial in ensuring high β-glucosidase activity in the batch cultures of T. harzianum. The results also agreed with the high F-values obtained for both factors (). While it has been indicated that the use of appropriate fungal inoculum size would yield extracellular β-glucosidase showing high specific activity [Citation39], the present study found that, increasingly, an inoculum size beyond 6% was counterproductive and reduced β-glucosidase activity. A similar trend has also been reported previously [Citation39–41]. A 6% (w/v) inoculum size to afford high β-glucosidase activity is substantially small as compared to that used in a previous work in [Citation42], where inoculum sizes of Aspergillus niger >6% and 8% (v/v) were used for cultures growing on peel and pulp of pineapple, respectively. Remarkably, a higher inoculum size of A. niger at 15% (v/v) was used in [Citation43] to yield high β-glucosidase activity in growth media containing coir waste.
Figure 3. Response surface (a) and contour (b) plots showing the interactive effect of A: temperature and C: inoculum size on the activity of β-glucosidase.
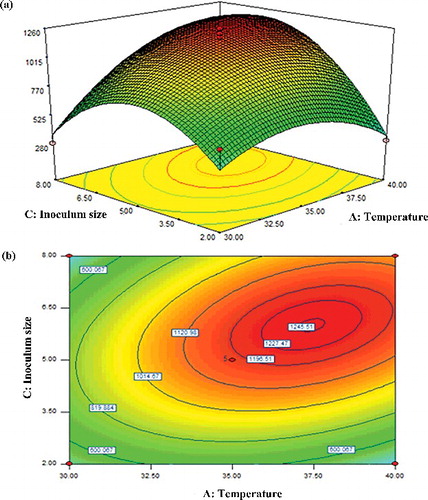
Likewise, a high inoculum size has also been linked to the production of microbial metabolites. Apart from producing β-glucosidases, microbes also secrete other types of metabolites that may interfere with the activity of the enzyme. As a matter of fact, the decline in β-glucosidase activity seen here at inoculum levels beyond 6% could likely be due to presence of higher amounts of inhibitory metabolites. Additionally, a high inoculum size would also lead to other problems, such as introduction of an unwanted large number of spores into the culture medium, which would promote rapid fungal proliferation and exhaustion of the available nutrients [Citation44]. The nutrients in the growth culture would be depleted sooner, steadily decreasing the metabolic activity of the T. harzianum biomass and, consequently, resulting in lower β-glucosidase production and enzyme activity. Moreover, the moisture level of the culture system is increased, creating conditions that promote overcrowding of fungal spores [Citation42]. Ideally, a balance between the proliferating biomass and the nutrients available for it would allow maximum fungal growth as well as maintaining enzyme synthesis. In contrast, the results also revealed that a low inoculum size <6% was unsuitable probably due to a shortened lag phase. Crucially, an adequately long lag phase is required for the fungal culture to prepare the internal machinery to produce the enzyme and attain high activity [Citation42].
Effect of temperature and pH
The pH of the medium is one of the key factors that would affect a fermentation process, influencing the microbial growth and enzyme activity [Citation45]. Considering that growth parameters involving temperature and pH could considerably affect the activity of β-glucosidase [Citation46], their interactive effects were examined and the factors inoculum size and carbon sources were kept constant at 5% and 10% (w/v), respectively. illustrates the response surface and contour plots for the effects of these two factors, AD. While the linear term for temperature (F = 18.30) was more significant than that of pH (F = 10.28) in influencing the β-glucosidase activity, inspection of the generated surface and contour plots showed that, at moderate levels of both temperature and pH, the activity of β-glucosidase was enhanced appreciably. The enzyme activity was significantly increased with conditions of elevated reaction temperature and pH, up to 36 °C and pH 7.2, respectively, with a maximum response of 1210.84 U/mL. Correspondingly, the negative interactive term (−161.43AD) () also justified the outcome seen in this study. A simultaneous increase in both temperature and pH beyond their optimum limits would not bring about improvement in β-glucosidase activity in the fungal cultures.
Figure 4. Response surface (a) and contour (b) plots showing the interactive effect of A: temperature and D: pH on the activity of β-glucosidase.
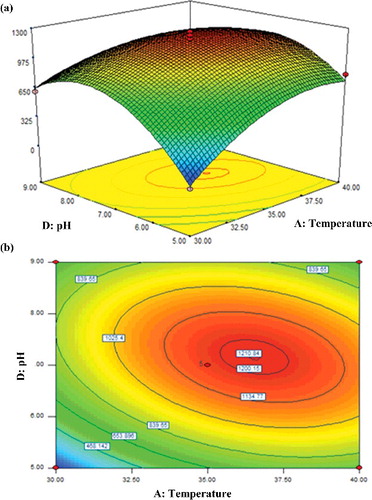
The particularly low β-glucosidase activity at low pH levels was likely brought about by the extreme low pH values that dramatically shifted the pH transition region for the unfolding of the β-glucosidase system [Citation37]. Since conditions of elevated pH and temperature have been thought to improve β-glucosidase activity [Citation47], the relatively moderate optimum growth temperature and pH that produced high β-glucosidase activity in the broth cultures of T. harzianum seen in this study were unlike those previously reported. Zimbardi et al. [Citation48] observed that maximum activities of β-glucosidases produced by T. viride and T. reesei occurred at higher optimum temperatures of 65–70 °C but at a considerably lower optimum pH, ranging from pH 4.5–5.5. This is because such fungal strains generally prefer acidic environments for their growth on cellulosic substrates, well-known examples of such strains being T. reesei [Citation19,Citation49] and Aspergillus heteromorphus [Citation50]. The studies reported that the activities of β-glucosidase produced by both these fungi peaked when the pH of the broth cultures was maintained at pH 5. In fact, the preferred neutral optimal pH in the broth cultures of T. harzianum is similar to that reported for an A. niger isolate [Citation51].
Effect of carbon sources and pH
It is acknowledged that the presence of simple and readily metabolizable carbon sources induces catabolic repression [Citation32]. In view of the situation, the interactive effect of various carbon sources and pH that affect the activity of β-glucosidase was evaluated and the results are illustrated in . In this evaluation, the inoculum size and temperature were kept constant at their central values of 5% (w/v) and 35 oC, respectively. The model indicated that their mutual interaction, BD, had a significant effect (P = 0.0399) on β-glucosidase activity (). However, with respect to the linear coefficients for pH and carbon sources, the impact of pH of the broth culture was more significant as compared to that of the carbon source. Hence, the pH value (F = 10.28) of the broth culture showed a larger F-value than the carbon sources (F = 6.31) ().
Figure 5. Response surface (a) and contour (b) plots showing the interactive effect of B: carbon sources and D: pH on the activity of β-glucosidase.
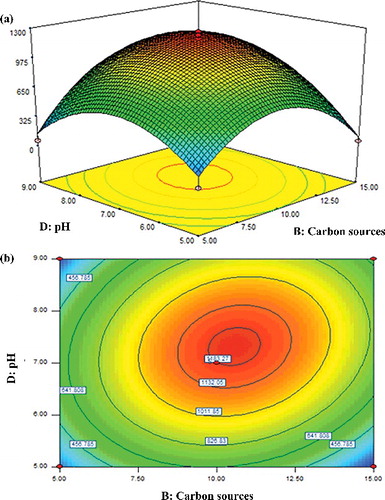
Since analysis of the surface response revealed that the stationary point for β-glucosidase activity was a saddle, the critical levels of the design factors were determined by ridge analysis to produce the maximum response. The critical values that represented the maximum β-glucosidase activity as high as 1483.27 U/mL were observed when the amount of carbon source and pH were increased up to a certain extent, at approximately 10% (w/v) and pH 7.3, respectively (). The outcome seen here is reflected in its positive value (+142.75BD) () and corroborated an earlier study by Al-Jazairi et al. [Citation52], in which the activity of β-glucosidase produced by Brettanomyces lambics was the highest when the fungus was cultured at both high pH and high content of carbon source.
The use of cheaper sources of carbon in cultivation media is one of the key considerations to lowering the cost of media design. This is principally due to the cost of media constituting approximately 30−40% of production costs [Citation53]. Hence, the present study resorted to using discarded agro-based biomass, i.e. banana wastes, as a carbon source to potentially circumvent this problem. The use of discarded agro-based biomass may prove more practical to produce β-glucosidase in this study. The role of banana wastes in the batch culture of T. harzianum was to induce and promote production of β-glucosidase, in response to complex carbohydrate constituents in the banana wastes. Furthermore, some species of the heterotrophic T. harzianum have been reported to express various isoforms of β-glucosidase by varying the carbon sources [Citation54,Citation55]. The existence of multiple isoforms of the enzyme has been attributed to differences in the expression of multiple β-glucosidase enzyme proteins [Citation55]. Zhang et al. [Citation9] have also reported that the type and level of β-glucosidase expression is dependent on some properties of the substrate used in the growth media. Pertinently, we believe that such an approach may prove favourable during cultivation of T. harzianum as most likely, it would improve the ability of the enzyme to inhibit M. phaseolina growth in the plate inhibition assay. Based on the results, it can be construed that T. harzianum was capable in utilizing the complex carbohydrates in banana wastes for growth and production of β-glucosidase.
Verification of the effect of T12 β-glucosidase on the growth of M. phaseolina M2 by plate inhibition assay
To check the suitability of the generated model, sets of confirmation experiments were carried out within and outside the design space. The optimization function of Design Expert 7.1.2. was employed; three predicted optimum culture growth conditions derived from ridge analysis of RSM that would give the maximum responses were selected. In this evaluation, plate inhibition assays were used for verifying the efficacy of the β-glucosidase from the T. harzianum T12 supernatant to inhibit the growth of the most virulent strain M. phaseolina M2 (). The β-glucosidase activities and their corresponding diameters of the halo zone were determined and the results from the verification experiments are represented in . Maximum activity at 1181.01 U/mL that gave the largest halo zone was observed for R1, followed by R2 and R3, corresponding to diameters of inhibition zones of 58.00 ± 2.51 mm, 49.33 ± 0.66 mm and 38.66 ± 1.85 mm, respectively. The largest halo zone seen in R1 also showed that these culture conditions, viz. 10% (w/v) carbon source, 5% (w/v) inoculum size, pH 7.00 and 35 ˚C, gave the highest β-glucosidase activity (). Pertinently, the values of the actual and predicted enzyme activity were seen to be relatively close; thus verifying the eligibility of the predicted model. This was consistent with the significantly low deviation percentages that were well below the maximum 5% error, ranging from 0.09−0.44% calculated for the actual responses and predicted values obained in R1, R2 and R3. These values strongly implied the accuracy and reliability of the model to predict the optimal growth conditions of T. harzianum T12, yielding high β-glucosidase activity and, consequently, more effective inhibition of M. phaseolina. Interestingly, similar inhibition has been reported in in vitro tests using pure T. harzianum isolates as well as chemical fungicides [Citation56]. The exception is that the method proposed here would be more practical as it does not involve utilization and release of harmful chemicals into the environment. Since the enzyme activity is optimum at 35 ˚C, such a preparation would be highly active in hot areas in the warm season, conditions when M. phaseolina infections are particularly prevalent. Direct usage of β-glucosidase from culture supernatant for long-term sustainable protection of commercial crops against charcoal rot, however, may be met with skepticism. Nevertheless, the simplicity of the preparations as well as the observed in vitro efficacy to inhibit the growth of M. phaseolina in this study strongly imply it could be feasible as a complement to the current agronomical pest management techniques. Since the findings seen here are preliminary and are only based on in vitro evaluation, subsequent studies constituting greenhouse and open-field studies would be necessary to further confirm the efficacy of this proposed method.
Figure 6. Plate inhibition assay showing the growth of M. phaseolina (denoted by dark areas) inhibited by the β-glucosidase (halo zone) extracted from T. harzianum.
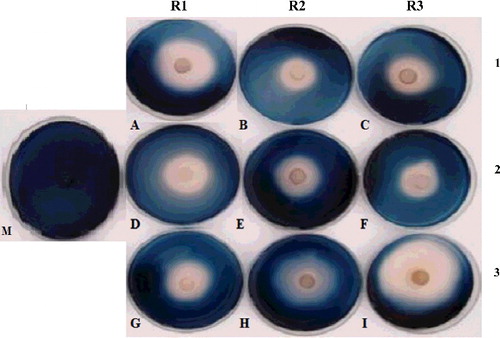
Table 6. Comparison of the predicted and experimental values for β-glucosidase activity obtained from the batch cultures of T. harzianum Rifai.
Conclusions
In this study, a high correlation was obtained for the BBD model, indicating that a quadratic polynomial model could be employed to optimize the batch cultivation of T. harzianum Rifai for maximizing β-glucosidase activity. The RSM model generated in this study satisfied all the necessary arguments for its use in the optimization. The findings revealed that the optimum conditions for the highest β-glucosidase activity (1483.27 U/mL) were at 35 ˚C, 10% (w/v) carbon source, 5% (w/v) inoculum size and pH 7.30. It is important to highlight here, that improving the β-glucosidase activity obtained from the supernatant of T. harzianum T12 would aid in its application for subsequent greenhouse and field studies. In a nutshell, the study intended to highlight the feasibility and potential applicability of direct treatment with β-glucosidase as an alternative to using chemical fungicides to manage the fungal phytopathogen M. phaseolina. Therefore, further studies should also focus on elucidating the mechanism of in situ contact inhibition of M. phaseolina by this enzyme.
Disclosure statement
No potential conflict of interest was reported by the authors.
Additional information
Funding
References
- Rayatpanah S, Nanagulyan SG, Alavi SV, et al. Pathogenic and genetic diversity among Iranian isolates of Macrophomina phaseolina. Chilean J Agric Res. 2012;72:1–40.
- Ganeshamoorthi P, Anand T, Saravanan A, et al. Cultural and genetic variability in Macrophomina phaseolina (Tassi.) Goid and incidence of Mulberry root rot. Arch Phytopath Plant Protec. 2010;43(2):123–132.
- Abbasi MW, Zaki MJ. Bioefficacy of microbial antagonists against Macrophomina phaseolina on sunflower. Pak J Bot. 2010;42(4):2935–2940.
- Aboshosha SS, Attaalla SI, El-Korany AE, et al. Characterization of Macrophomina phaseolina isolates affecting sunflower growth in El-Behera governorate. Egypt Int J Agric Biol. 2007;9(6):807–815.
- Khalili E, Javed MA, Huyop F, et al. Evaluation of Trichoderma isolates as potential biological control agent against soybean charcoal rot disease caused by Macrophomina phaseolina. Biotechnol Biotechnol Equip. 2016;30(3):479–488.
- Ainsworth GC, Sussman AS. The fungal population: an advanced treatise. New York (NY): Elsevier; 2013.
- Manera AP, Ores JC, Ribeiro VA, et al. Optimization of the culture medium for the production of b-galactosidase from Kluyveromyces marxianus CCT 7082. Food Technol Biotech. 2008;46(1):66–72.
- Monteiro VN, do Nascimento Silva R, Steindorff AS, et al. New insights in Trichoderma harzianum antagonism of fungal plant pathogens by secreted protein analysis. Curr Microbiol. 2010;61(4):298–305.
- Zhang Z, Liu JL, Lan JY, et al. Predominance of Trichoderma and Penicillium in cellulolytic aerobic filamentous fungi from subtropical and tropical forests in China, and their use in finding highly efficient β-glucosidase. Biotechnol Biofuels. 2014;17(7):101–107.
- Gajera HP, Bambharolia RP, Patel SV, et al. Antagonism of Trichoderma spp. against Macrophomina phaseolina: evaluation of coiling and cell wall degrading enzymatic activities. J Plant Pathol Microbiol. 2012;3:149–230.
- Coronel-León J, Marqués AM, Bastida J, et al. Optimizing the production of the biosurfactant lichenysin and its application in biofilm control. J Appl Microbiol. 2016;120(1):99–111.
- Joshi S, Bharucha C, Jha S, et al. Biosurfactant production using molasses and whey under thermophilic conditions. Bioresour Technol. 2008;99(1):195–199.
- Saimmai A, Sobhon V, Maneerat S. Molasses as a whole medium for biosurfactants production by Bacillus strains and their application. Appl Biochem Biotechnol. 2011;165(1):315–335.
- Zhu Z, Zhang F, Wei Z, et al. The usage of rice straw as a major substrate for the production of surfactin by Bacillus amyloliquefaciens XZ-173 in solid-state fermentation. J Environ Biol. 2013;30(127):96–102.
- Slivinski CT, Mallmann E, de Araújo JM, et al. Production of surfactin by Bacillus pumilus UFPEDA 448 in solid-state fermentation using a medium based on okara with sugarcane bagasse as a bulking agent. Process Biochem. 2012;47(12):1848–1855.
- Wahab RA, Basri M, Rahman RNZRA, et al. Enzymatic production of a solvent-free menthyl butyrate via response surface methodology catalyzed by a novel thermostable lipase from Geobacillus zalihae. Biotechnol Biotechnol Equip. 2014;28(6):1065–1072.
- Manan FMA, Rahman INA, Marzuki NHC, et al. Statistical modelling of eugenol benzoate synthesis using Rhizomucor miehei lipase reinforced nanobioconjugates. Process Biochem. 2016;51(2):249–262.
- Wang H, Feng JT, Zhang Q, et al. Optimization of fermentation condition for antibiotic production by Xenorhabdus nematophila with response surface methodology. J Appl Microbiol. 2008;104(3):735–744.
- Isah AA, Mahat NA, Jamalis J, et al. Synthesis of geranyl propionate in a solvent-free medium using Rhizomucor miehei lipase covalently immobilized on chitosan–graphene oxide beads. Prep Biochem Biotechnol. 2016;3:1–12.
- Tari C, Ustok FI, Harsa S. Optimization of the associative growth of novel yoghurt cultures in the production of biomass, β-galactosidase and lactic acid using response surface methodology. Prep Biochem Biotechnol. 2009;19(4):236–243.
- Sriphannam W, Unban K, Ashida H, et al. Medium component improvement for β-galactosidase production by a probiotic strain Lactobacillus fermentum CM33. Afr J Biotechnol. 2012;11(51):11242–11251.
- Li C J, Zhang X, Zhang LP, et al. Medium optimization for the production of a metagenome-derived-galactosidase by Pichia pastoris using response surface methodology. Afr J Microbiol Res. 2013;7(13):1077–1085.
- Goujon M, McWilliam H, Li W, et al. new bioinformatics analysis tools framework at EMBL–EBI. Nucleic Acids Res. 2010;38(2):W695–W699.
- Iqbal HMN, Asgher M, Bhatti HN. Optimization of physical and nutritional factors for synthesis of lignin degrading enzymes by a novel strain of Trametes versicolor. Bioresources. 2011;6(2):1273–1287.
- Shahzadi T, Anwar Z, Iqbal Z, et al. Induced production of exoglucanase, and [beta]-glucosidase from fungal co-culture of T. viride and G. lucidum. Adv Biosci Biotechnol. 2014;5:426–433.
- Irshad MN, Anwar Z, But HI, et al. The industrial applicability of purified cellulase complex indigenously produced by Trichoderma viride through solid-state bio-processing of agro-industrial and municipal paper wastes. Bioresources. 2012;8(1):145–157.
- Kaur J, Chadha BS, Kumar BA, et al. Purification and characterization of ß-glucosidase from Melanocarpus sp. MTCC 3922. Electron J Biotechnol. 2007;10(2):260–270.
- Terra VS, Homer KA, Rao SG, et al. Characterization of novel β-galactosidase activity that contributes to glycoprotein degradation and virulence in Streptococcus pneumoniae. Infect Immun. 2010;78(1):348–357.
- Job J, Sukumaran RK, Jayachandran K. Production of a highly glucose tolerant β-glucosidase by Paecilomyces variotii MG3: optimization of fermentation conditions using Plackett–Burman and Box–Behnken experimental designs. World J Microbiol Biotechnol. 2010;26(8):1385–139. 1.
- Haaland PD. Experimental design in biotechnology. Vol. 105. Boca Raton (FL): CRC Press; 1989.
- Batra J, Beri D, Mishra S. Response surface methodology based optimization of β-glucosidase production from Pichia pastoris. Appl Biochem Biotechnol. 2014;172(1):380–393.
- Al-Jazairi M, Abou-Ghorra S, Bakri Y, et al. Optimization of β-galactosidase production by response surface methodology using locally isolated Kluyveromyces marxianus. Int Food Res J. 2015;22(4):1361–1367.
- Zahoor S, Javed MM, Aftab S, et al. Metabolic engineering and thermodynamic characterization of an extracellular β-glucosidase produced by Aspergillus niger. Afr J Biotechnol. 2011;10(41):8107–8116.
- Park AR, Park JH, Ahn HJ, et al. Enhancement of β-glucosidase activity from a brown rot fungus Fomitopsis pinicola KCTC 6208 by medium optimization. Mycobiol. 2015;43(1):57–62.
- Deka D, Bhargavi P, Sharma A, et al. Enhancement of cellulase activity from a new strain of Bacillus subtilis by medium optimization and analysis with various cellulosic substrates. Enzy Res. 2011 [ cited March 1]; [8 p.]. DOI:10.4061/2011/151656.
- Wang Y, Xu Y, Li JA. Novel extracellular β-glucosidase from Trichosporon asahii: Yield prediction, evaluation and application for aroma enhancement of cabernet sauvignon. J Food Sci. 2012;77(8):505–515.
- Olajuyigbe FM, Nlekerem CM, Ogunyewo OA. Production and characterization of highly thermostable β-glucosidase during the biodegradation of methyl cellulose by Fusarium oxysporum. Biomed Res Int. 2016 [ cited February 24]; DOI:10.1155/2016/3978124
- Reddy N, Yang Y. Biofibres from agricultural byproducts for industrial application. Trends Biotechnol. 2005;23(1):22–27.
- Chauhan PS, Bharadwaj A, Puri N, et al. Optimization of medium composition for alkali-thermostable mannanase production by Bacillus nealsonii PN-11 in submerged fermentation. Int J Curr Microbiol Appl Sci. 2014;3(10):1033–1045.
- Mahajan PM., Desai KM, Lele SS. Production of cell membrane-bound α-and β-glucosidase by Lactobacillus acidophilus. Food Bioprocess Tech. 2012;5(2):706–718.
- Ali MB, Irshad M, Anwar Z, et al. Screening and statistical optimization of physiochemical parameters for the production of xylanases from agro-industrial wastes. Adv Space Res. 2016;4:1–20.
- Iqbal S, Nguyen TH, Nguyen TT, et al. β-Galactosidase from Lactobacillus plantarum WCFS1: biochemical characterization and formation of prebiotic galacto-oligosaccharides. Carbohydr Res. 2010;345(10):1408–1416.
- Mahapatra S, Vickram AS, Sridharan TB, et al. Screening, production, optimization and characterization of β-glucosidase using microbes from shellfish waste. Biotechnology. 2016;6(2):198–213.
- Vikash K, Irshita V, Deepak K, et al. Cellulase and β-glucosidase production by Trichoderma viride and Aspergillus wentii in sub-merged fermentation utilizing pretreated lignocellulosic biomass. J Microbiol Biotechnol. 2013;3(5):63–78.
- Shata HM, El-Deen AMN, Nawwar GA, et al. β-Glucosidase production by mixed culture using crude hemicellulose from rice straw black liquor and peat moss as an inert support. Egypt Pharmaceut J. 2014;1(13):121–129.
- Teixeira da Silva VDC, de Souza Coto AL, de Carvalho Souza R, et al. Effect of pH, temperature, and chemicals on the endoglucanases and β-glucosidases from the thermophilic fungus Myceliophthora heterothallica F. 2.1. 4. obtained by solid-state and submerged cultivation. Biochem Res Int. 2016 [cited 2017 March 3]; [9781216]. DOI:10.1155/2016/9781216.
- Garcia NFL, Santos FRDS, Gonçalves FA, et al. Production of β-glucosidase on solid-state fermentation by Lichtheimia ramosa in agroindustrial residues: characterization and catalytic properties of the enzymatic extract. Electron J Biotechnol . 2015;18(4):314–319.
- Zimbardi AL, Sehn C, Meleiro LP, et al. Optimization of β-glucosidase, β-xylosidase and xylanase production by Colletotrichum graminicola under solid-state fermentation and application in raw sugarcane trash saccharification. Int J Mol Sci. 2013;14(2):2875–2902.
- Razak MNA, Ibrahim MF, Yee PL, et al. Utilization of oil palm decanter cake for cellulase and polyoses production. Biotechnol Bioprocess Eng. 2012;17(3):547–555.
- Da Silva Delabona P, Pirota RDPB, Codima CA, et al. Effect of initial moisture content on two Amazon rainforest Aspergillus strains cultivated on agro-industrial residues: Biomass-degrading enzymes production and characterization. Ind Crops Prod. 2013;42:236–242.
- Abdella A, El-Sayed Mazeed T, Yang ST, et al. Production of β-glucosidase by Aspergillus niger on wheat bran and glycerol in submerged culture: factorial experimental design and process optimization. Curr Opin Biotechnol. 2014;51(10):1331–1337.
- Hmad IB, Abdeljalil S, Saibi W, et al. Medium initial pH and carbon source stimulate differential alkaline cellulase time course production in Stachybotrys microspora. Appl Biochem Biotechnol. 2014;172(5):2640–2649.
- Saibi W, Gargouri A. Purification and biochemical characterization of an atypical β-glucosidase from Stachybotrys microspora. J Mol Catal B: Enzym. 2011;72(3):107–115.
- Nakazawa H, Kawai T, Ida N, et al. Construction of a recombinant Trichoderma reesei strain expressing Aspergillus aculeatus β-glucosidase 1 for efficient biomass conversion. Biotechnol Bioeng. 2012;109(1): 92–99.
- Singhania RR, Patel AK, Sukumaran RK, et al. Role and significance of beta-glucosidases in the hydrolysis of cellulose for bioethanol production. Bioresour Technol. 2013;127:500–507.
- Abdullah MT, Ali NY, Suleman P. Biological control of Sclerotinia sclerotiorum (Lib.) de Bary with Trichoderma harzianum and Bacillus amyloliquefaciens. Crop Prot. 2008;27(10):1354–1359.