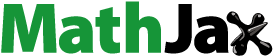
ABSTRACT
The increasing threat to human health and aquatic life caused by nitrogen pollution in surface waters requires critical attention. The membrane-aerated biofilm reactor (MABR) is a promising alternative compared to conventional technologies. In this work, a pilot-scale MABR system was employed to treat polluted surface water in continuous-flow mode. The nitrogen removal performances with the variations of the aeration parameters, hydraulic loading and C/N ratio were explored. The results showed that intra-membrane pressure plays a dominant role in improving the denitrification rate. The nitrification dynamics of the MABR system were favoured in conditions of low influent C/N ratios and hydraulic loading, but limit the denitrification process due to insufficient carbon source. A counter optimal hydraulic loading of 0.016 m3/(m2 day) (hydraulic retention time of 36 h), and a corresponding increase in the influent C/N ratio was beneficial for nitrogen removal. When the influent C/N ratio was raised to a factor of 8, the total organic carbon (TOC), ammonium and total nitrogen (TN) removal efficiency could reach 80.0%, 92.2% and 60.3%, respectively. This indicated that the MABR has a potential to remove TN by simultaneous nitrification and denitrification (SND) in polluted surface water treatment.
Introduction
Surface water is considered to be one of the most severely polluted water sources around the world [Citation1,Citation2]. Increasing discharge of unregulated sewage and municipal wastewater, industrial wastewater and over-usage of agricultural fertilizers and pesticides are major sources responsible for surface water nitrogen contamination [Citation3]. These nitrogenous pollutants (ammonia, nitrite and nitrate) in water bodies not only can cause eutrophication, which is responsible for the deterioration of water quality and the alteration of the ecological structure in freshwater, but also pose great potential hazards to human and animal health [Citation4]. It is urgent and significant to find ways to treat nitrogen-polluted surface waters to better preserve the environment, as well as human health.
Currently, various in situ bioremediation processes including ecological floating bed (EFBs) and constructed wetlands (CWs) have been developed for enhanced removal of nitrogen in polluted surface water and have achieved satisfactory results [Citation5–9]. Compared to bioreactor techniques with biofilms, however, these processes are typically time consuming, inducing secondary pollution and having great dependence on environment conditions [Citation10,Citation11]. Bioreactor techniques are a matured technology widely used in wastewater treatment and have great potential in surface-water nitrogen removal [Citation10,Citation12]. However, the choice of biofilm carriers and bioreactor designs has a great influence on the energy consumption and the treatment efficiency [Citation10]. Therefore, promotion of practical and cost-effective treatment technologies is urgent for surface water total nitrogen (TN) removal and water quality protection.
The membrane-aerated biofilm reactor (MABR) process represents a promising biofilm technology for polluted surface water treatment, and has recently gained much attention [Citation13–15]. In MABR systems, permeable hollow fiber membranes are adapted as platform for the attachment of biofilm and also as bubbleless oxygen supplier with high oxygen transfer efficiency [Citation16]. The air flows through the membrane lumen and transfers to the membrane outer layer under an appropriate pressure. The oxygen diffuses along the thickness of the biofilm towards the wastewater and is utilized by the micro-organisms. Meanwhile, the wastewater pollutants penetrate into the depth of the biofilm and thus, create a counter-diffusion mechanism suitable for both aerobic and anaerobic bacteria, in a symbiotically controlled environment enhancing nitrogen removal. It is generally accepted that the aerobic region of the membrane–biofilm interface is favourable for ammonia-oxidizing bacteria (AOB) and nitrite-oxidizing bacteria (NOB) due to the oxygen-rich conditions, while the anoxic region of the biofilm–liquid interface is more suitable for denitrifying bacteria [Citation17]. Therefore, nitrogen removal can be realized by simultaneous nitrification and denitrification using a single biofilm system [Citation18,Citation19]. Compared to the conventional co-diffusion biofilm systems through the nitrification/denitrification pathway, the simultaneous nitrification and denitrification (SND) in MABR offers considerable superiorities [Citation20], including, (i) high oxygen mass transfer efficiency and utilization leading to less energy input [Citation16,Citation21,Citation22]; (ii) less carbon source required and sludge yield reduction. The carbon source can be made the most of by the heterotrophic denitrification bacteria through the mass transfer counter-diffusion MABR system [Citation19,Citation23].
By taking advantage of this innovative technology, MABR technology has been mainly applied for the treatment of municipal wastewater [Citation24–26], industrial wastewater (landfill leachate [Citation27], pharmaceutical wastewater [Citation28], dyes [Citation29] and so on) to remove carbon and nitrogen. MABR is promising for the treatment of high strength wastewater. Biological denitrification requires available organic carbon as an electron donor. Therefore, the nitrogen removal efficiency of wastewater is considerably dependent on the carbon/nitrogen ratio (C/N) [Citation30,Citation31]. The C/N ratio of polluted surface water is, however, often significantly lower than that of domestic wastewater or severely polluted wastewater. The lack of sufficient organic carbon source always limits the nitrogen removal performance with conventional biofilm technologies [Citation32]. So far, only few studies have shown the feasibility of using MABR for surface water nitrogen removal with varying C/N ratios. Li et al. [Citation14] described an enhanced MABR system for the treatment of surface water with two phases. In phase I, halfway-aerated membrane modules were used to create simultaneous hydrolytic acidification and aerobic oxidation, which consequently enhanced the organic matter degradation. In phase II, all membrane modules were aerated and augmented with the addition of polyhydroxyalkanoates (PHA) as carbon source to increase the TN and total phosphorus (TP) removal efficiencies. Though the surface water quality was sustainably maintained, it may be difficult to realize large-scale treatment due to the complex operation process and increased cost of treatment as well as the operation mode (batch mode). Thus, it is desirable to draw on the application of MABR for nitrogen removal from polluted surface water utilizing simple operation techniques, little or no external carbon source and operation in continuous flow mode, as opposed to batch systems where the water is assumed to be in non-flow mode.
Based on our previews laboratory-scale experiment on MABR for simulated surface water treatment in sequencing batch mode, we preliminarily investigated the effects of fundamental aeration parameters (aeration intensity, air pressure and membrane surface areas) on pollutants removal [Citation33]. The results indicated that it is feasible to treat the polluted surface water by MABR and with moderately efficient TN removal capacity. However, detailed knowledge of nitrogen removal characteristics in MABR system and key technical operational parameters which govern the successful and stable application for surface water treatment are still lacking. In this work, a pilot-scale MABR system was designed to treat polluted surface water under various operating conditions. In the initial phase, the intra-membrane air flow velocity and intra-membrane air pressure were explored and controlled to create a favourable denitrification environment. Then, at the intermediate phase, the nitrogen removal performance of the MABR system under various hydraulic loading was investigated. Finally, based on the optimal conditions, the effects of C/N ratio on SND were observed and optimized to achieve high nitrogen removal efficiency of MABR. By implication, this advanced treatment process can help to achieve cost-effective treatment of nitrogenous polluted surface water with a low C/N ratio.
Materials and methods
Synthetic surface water medium
Artificially simulated polluted surface water was used as influent for the pilot-scale MABR system. Glucose and NH4Cl were used as the main carbon and nitrogen source, respectively. The concentration of NH4+-N was fixed at 25 mg/L and the total organic carbon (TOC) concentration was varied from 30 to 200 mg/L following the experimental design to obtain various C/N ratios at different operation stages. Other components of the synthetic surface water include (per liter of tap-water): 34 mg monopotassium phosphate, 55 mg magnesium sulphate, 45 mg potassium sulphate, 45 mg sodium chloride, 45 mg sodium bicarbonate and 115 mg sodium carbonate.
Experimental set-up
The pilot-scale continuous-flow MABR system was designed and used for the experiment study as shown in . The membrane-aerated bioreactor made of polyvinyl chloride had a working volume of 470 L, containing four flat-sheet curtain membranes (Supplementary Figure S1a) parallel to each other and connected by air circulating pipes. Each flat-sheet consists of a bunch of hollow-fibers with an estimated length of 1.2 m. The micro-porous fibers are made of polyvinylidene fluoride (PVDF) with average pore size of 0.1 µm, membrane wall thickness of 50 µm and porosity of 50%, belonging to high gas-permeable membranes. The total membrane surface area of the MABR reactor was 20 m2, with a specific surface area of 54.1 m2/m3. Air was supplied by an air compressor flow from down to top. The air flow velocity and transmembrane pressure were controlled by a flow meter and a pressure gauge before entry and exit of the membrane lumen, forming a flow-through membrane aeration mode. A perspex square tank was used to store the artificial wastewater with a volume of 300 L. The simulated surface water was pumped into the reactor at flow rates that provided hydraulic retention times (HRTs) of 12–48 h. The treated water was collected and analyzed at the effluent end of the reactor. Supplementary Figure S1b represents the process of MABR before influent loading.
Analytical methods
Ammonium (NH4+-N) concentration was measured using Nessler's reagent and Multiparameter Bench photometer (LH5B-3(B), Lianhua Instruments Inc., China). Given the relative stable compositions of the wastewater, fast analysis speed and non-secondary pollution, TOC was used as indicator for the effluent water quality in this work. TN and TOC concentrations were measured using TOC-VCPH analyzer (Shimadzu, Japan). Nitrate (NO3−-N) and nitrite (NO2−-N) were analyzed by ion chromatography (IC3000 with AS11/AG11 column, Dionex Corp, Sunnyvale CA, USA). Dissolved oxygen (DO) and pH were monitored using a DO meter (Hatch) equipped with an oxygen electrode. Samples were collected in triplicates and filtered through 0.45 µm nitrocellulose membrane filters prior to analysis.
Biofilm cultivation and formation
Fresh activated sludge was taken from Jimei Municipal Wastewater Treatment Plant, Xiamen, China, at the return sludge compartment. The characteristics of the feed sludge were measured after 4 d cultivation. The initial sludge concentration mixed liquor suspended solids (MLSS) was 3585 mg/L; the sludge volume index was 68; pH 6.09 and DO concentration of 8.16 mg/L. The cultivation continued for two more days before shutting down the bioreactor aeration. Stable gray biofilm was clearly seen attached on the holler fiber membrane surfaces after discharge of sludge. Following the biofilm culture, a 26 d period of inoculation was ensued to ensure stability of the effluent quality. During the initial phase of the experiment, influent containing concentrations of ammonia 50 mg/L and chemical oxygen demand (COD) 200 mg/L, was loaded in the MABR system for a period of 26 d while controlling the aeration pressure under the bubble point and keeping the HRT at 33 h. Consequently, the MABR achieved steady performance in terms of TOC and ammonia removal with concentrations less than 2 and 14.5 mg/L, respectively, in the effluent. The removal efficiencies were 81% and 97%, respectively. Meanwhile, a well-distributed biofilm was established based on visual observation as shown in Supplementary Figure S2 from a top-view. Due to the changes of influent loading at different stages and the biofilm intrinsic characteristics of MABR, there is an obvious variation in biofilm thickness after the startup of MABR in flow-through mode operation.
Operating process parameterization
shows the HRT, the influent pollutants loading rate, C/N ratio, hydraulic loading and aeration parameters during the MABR experimental process after the 26 d biofilm acclimatization period. During the initial phase, different intra-membrane air-flow velocity and intra-membrane air pressure values were adjusted. At the intermediate phase (stages I to IV), varying influent hydraulic loading was deployed to determine the optimal operating value by fixing the C/N ratio at 8 to achieve high nitrogen removal. In the final phase (stages V–VIII), the impact of the C/N ratio on SND was investigated.
Table 1. Operation conditions during the MABR process study.
Data analysis
Data are presented as mean values with standard deviation (±SD) from three measurements. Statistical analysis was performed using Origin software. Pollutants (TOC, NH4+-N, TN) removal efficiency (%) was calculated by the following equation:
where Cinf represents the pollutant concentration in the influent and Ceff is the pollutant concentration in the effluent.
Results and discussion
Aeration effect on MABR process
The synthetic surface water was continuously supplied at a C/N ratio of 8 and HRT of 16 h during the initial phase operation. In order to obtain a favourable bulk DO concentration (to achieve efficient denitrifying process), the oxygen supply was controlled by increasing the intra-membrane air flow rate (calculated by the air flow rate from inside the membrane to the bulk liquid phase) from 0.005 to 0.02 cm/min and decreasing the intra-membrane air pressure from 0.015 to 0.01 MPa, respectively. The hollow fiber membrane which has a length of over 1.0 m vertically submerged in the wastewater requires an air pressure value greater than the external hydrostatic head to operate the modules. The gas permeability of the PVDF hollow fiber membranes utilized in this work possesses a bubble point of 0.02 MPa and therefore, the membrane module hardly operates well at pressure values less than 0.005 MPa (due to water intruding the membrane pores, especially ruptured parts of the membrane). Thus, in this work, we adapted pressure values at 0.01 and 0.015 MPa for the investigation. The data presented in show that the DO concentrations fluctuated between 1.5 and 6 mg/L at different intra-membrane air flow rates (0.005, 0.01 and 0.02 cm/min) when the intra-membrane pressure was 0.015 MPa. The bulk DO concentration changed dramatically when the intra-membrane air pressure was decreased from 0.015 to 0.01 MPa (). Similar observations were made by Gong et al. [Citation34] in the CANON process of MABR treating ammonium-rich wastewater. Subsequently, in the latter part of the initial phase (the remaining 12 d period), the MABR which was operated continuously with intra-membrane air pressure of 0.01 MPa (at different air flow rate of 0.005, 0.01 and 0.02 cm/min, respectively) resulted in a low bulk DO concentration (0–0.5 mg/L), and thus provided favourable conditions for denitrifying bacterial growth as shown in . Moreover, it appears that the denitrification rate was more favoured at pressure values of 0.01 MPa than at 0.015 MPa. The denitrifying performance reached the highest with a rate of 0.52 g-N/(m2 day) at an intra-membrane air flow rate of 0.005 cm/min and pressure value of 0.01 MPa. This is mainly because the intra-membrane air pressure plays a dominant role in controlling the oxygen transfer efficiency [Citation27,Citation35].
Effects of hydraulic loading on nitrogen removal
Hydraulic loading in this work was defined as the wastewater treatment capacity (m3) per unit m2 of membrane in a day in the MABR process. Wei et al. [Citation35] found that increased hydraulic loading (feed flow velocity) enhanced the mass transfer of ammonium-rich wastewater treatment in facilitated transfer membrane-aerated biofilm reactor (FT-MABR) process with NH4+-N and TN removal efficiency increasing from 53.8% and 61.8%, to 85% to 95%, respectively. This demonstrated that hydraulic loading played a significant role in nitrogen removal. In this work, the hydraulic loading was increased from 0.012 to 0.047 m3/(m2 day) step-wise without changing the influent water quality (C/N ratio: 8) and aeration parameters (intra-membrane air flow velocity 0.005 cm/min, intra-membrane air pressure 0.01 MPa).
A shock hydraulic loading experiment was conducted by increasing the feed flow velocity as shown in . When the hydraulic loading varied from 0.012 to 0.024 m3/(m2 day), the effluent TOC concentration was varied between 10 and 20 mg/L. At hydraulic loading of 0.047 m3/(m2 day) as the resistance shock hydraulic loading capacity of the MABR treatment process was limited ((a)), the effluent TOC climbed to the range of 20–30 mg/L and the corresponding TOC removal efficiency dropped gradually. This may be as a result of the shock to the biofilm caused by the high volumetric loading. It was evidently observed during the experiment as parts of the biofilm were de-attached and peered off from the hollow fiber membrane surface under the higher feed water flow velocity of 0.047 m3/(m2 day).
Figure 4. Variations of TOC, NH4+-N and TN with operation time at different hydraulic loadings. TOC concentration and TOC removal efficiency (a); NH4 +-N and NH4+-N removal efficiency (b); TN and TN removal efficiency (c).
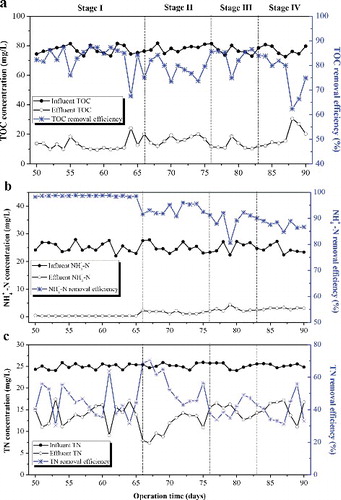
(b,c) show the time course for NH4+-N, and TN concentrations in the MABR with different hydraulic loading rates, respectively. It should be mentioned here that, though the influent ammonium concentration was controlled at 25 mg/L, there is possibility of slight fluctuation in concentration as this is commonly the case in polluted surface water. In stage I, effluent NH4+-N remained below 0.5 mg/L during the 16 day's operation and the removal efficiency was constantly high above 98% at hydraulic loading of 0.012 m3/(m2 day). Meanwhile, at the remaining stages of the intermediate phase (II, III and IV), the effluent TN was very high as shown in (b). Moreover, the effluent NH4+-N concentration appeared to increase gradually to over 3 mg/L with increasing hydraulic loading rates (0.016 to 0.047 m3/(m2 day)), while the effluent TN decreased temporarily, then gradually increased ((c)). The decreasing NH4+-N conversion rate may be caused by the limited hydraulic retention time. To verify this, the hydraulic loading rate was varied to 0.016 m3/(m2 day). Notably, a high TN removal efficiency was realized at over 40%. Though increased hydraulic loading rate promoted NH4+-N transfer from bulk to the biofilm interface, which resulted in increased NH4+-N removal in single batch treatment mode, this may be a disadvantage in continuous flow mode as the feed flow rate allowed little time of contact with the biofilm and thus, led to disparity in results obtained in this work other than those reported by other researchers [Citation35]. Based on the TN removal results, it can be concluded that an appropriate hydraulic loading rate is essential for the effective removal of nitrogen in the MABR process.
In continuous flow mode, the results showed that N species compositions, including ammonium, nitrite and nitrate, were different under different hydraulic loading rates (). At low hydraulic loading with longer HRT (48 h, 36 h), NH4+-N was efficiently removed and conversion of the nitrite intermediate into nitrate was dominantly achieved at this loading rate and further enhanced the denitrification performance. However, the inadequate TOC retention as carbon source for denitrification in longer HRT (48 h) inhibited the denitrifying process. Besides, the excessive oxygen supplied by the permeable membrane also presented a challenge to denitrifying bacteria. At 0.016 m3/(m2 day) hydraulic loading rate, a satisfactory nitrogen removal of 56% was achieved. By contrast, in high hydraulic loading with short HRT (24 h, 12 h), insufficient oxidation limited the loading capacity with respect to the specific membrane area. The unconverted ammonium and accumulated nitrite and nitrate resulted in poor nitrogen removal. The TN removal efficiency was around 33% at a hydraulic loading rate of 0.047 m3/(m2 day). This indicated that decreasing the hydraulic loading is an effective way to improve the nitrogen removal in continuous flow MABR processes.
Effects of C/N ratio on nitrogen removal
In order to explore the effect of C/N on nitrogen removal for surface water treatment, a long-term operation was conducted for over 60 days under different C/N ratios (2, 3.5, 5 and 8), at a constant hydraulic loading rate (0.016 m3/(m2 day)) and intra-membrane pressure of 0.01 MPa. The influent ammonium concentration was fixed at 25 mg/L and the influent total organic carbon (TOC) was varied to provide the different C/N ratios. displays the TOC, NH4+-N and TN removal efficiencies. NH4+-N removal was notably high at C/N ratios of 2, 3 and 5. However, the denitrification process turned out to be the rate-limiting step, which resulted in an inefficient TN removal at less than 20%. Meanwhile, an increase in the C/N ratio yielded a high TOC and ammonium removal simultaneously. The plentiful electron sourced from the TOC was favourable for denitrification. It is widely recognized that the increase in the C/N ratio is beneficial for TN removal [Citation36–38].
The variation of effluent concentration under different C/N ratios is shown in . The effluent TOC concentration was not affected by the influent C/N ratio, which can be kept at around 10 mg/L ((a)). There exists a slight elevation in effluent TOC concentration with the increase of the C/N ratio from 2 to 8 step-wisely. The TOC removal efficiency throughout this study was over 70%, except for the TOC removal value at C/N ratio of 2 with TOC removal efficiency lower than 50%. The increased TOC removal efficiency observed in MABR treatment at higher C/N ratio may be a result of the increased heterotrophic growth in the biofilm. During the initial 16 days’ operation at stage V of HRT 36 h under influent C/N ratio of 2, a moderate TOC removal efficiency of 50% was obtained, and decreased sharply to 12% at day 57. This indicated that the metastasis of bacteria requires the minimal C/N ratio for the TOC removal. The insufficient carbon source restricted the denitrification and heterotrophic bacterial activity at lower C/N ratio [Citation38].
Figure 7. TOC concentration in the influent and effluent and TOC removal efficiency (a) and nitrogen concentration of effluent (b).
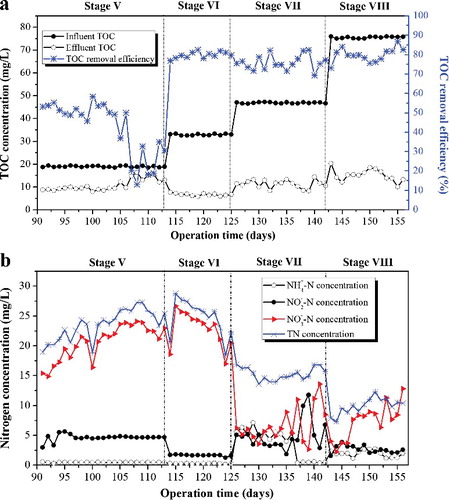
(b) displays the time-dependent variations of nitrogen species concentration to clarify the SND performance in the MABR system during the 68 days of operation. The NH4+-N concentration in the effluent was maintained near to 0 mg/L and there was no obvious NO2−-N accumulation in the effluent (lower than 5 mg/L) during the two stages (V and VI) at C/N ratio 2 and 3.5. However, a dramatic accumulation of NO3−-N was observed, indicating that the sufficient oxygen supply and poor denitrification resulted from the lack of electron donors. Such observations have also been reported by Matsumoto et al. [Citation39] and Lin et al. [Citation40]. After 36 days of operation, the feed C/N ratio was increased from 3.5 to 5 by supplementing the influent with glucose. The effluent NO3−-N decreased dramatically to less than 15 mg/L with minimum NO2−-N accumulation, which may be ascribed to the adequate carbon source as electron donor for the denitrification process, thus leading to the significant improvement of TN removal. This was further demonstrated by the enhancement in nitrogen removal by increasing the C/N ratio to 8 with effluent TN concentration reducing significantly to around 10 mg/L. Generally, the C/N ratio of surface water is low and thus, it is a common practice to augment the treatment by supplementing with external carbon source [Citation14]. However, under high influent C/N ratio, there existed a competition between nitrifying bacteria and heterotrophic bacteria for oxygen. Due to inhibition from the heterotrophic bacteria, which was unfavourable for nitrification, the concentration of NH4+-N in the effluent increased but was still maintained at low levels. The results further indicated that when the carbon source was sufficient, the C/N ratio could hardly affect the performance of nitrifying bacteria, and an effective balance between nitrification and denitrification could be obtained. By contrast, the lack of carbon source limited the denitrification process and led to significantly higher NO3−-N accumulation [Citation40]. Notably, the effluent NH4+-N and NO2−-N content decreased and the NO3−-N concentration was stably maintained without significant reduction as the influent C/N ratio increased from 5 to 8 ((b)). This also revealed that the activities of microorganisms are considerably affected by the wastewater C/N ratio. The functional bacteria (Planctomycetes sp.) for the ANAMMOX process under higher C/N ratio also exist in the MABR system [Citation40]. The NH4+-N and NO2−-N acted as the electron donor and electron acceptor, respectively, to yield nitrogen gas, which contributed to TN removal. However, the conventional aerobic nitrification–anoxic denitrification results in higher or maintained effluent NO3−-N concentration [Citation38,Citation41].
The time course of the TN removal efficiency is presented in . It is clear that at low influent C/N ratio (2, 3.5) at stage I, the TN removal efficiency decreased gradually from 22% to zero and to sub-zero values. During stage II, with the increase of C/N ratio from 2 to 3.5, the TN removal efficiency increased rapidly. At C/N ratio of 5 (stage VII) and 8 (stage VIII), comparatively stable TN removal was achieved at 38% and 60.3%, respectively. This indicated that the increase of influent C/N ratio is beneficial for TN removal, which was consistent with previous research conclusions [Citation38,Citation42].
The nitrogen removal performance of various techniques in surface water treatment is summarized in . Compared with ecological engineering processes, such as EFB and CWs, which generally consist of several stages, wide area coverage and complex operation processes with HRT varying from 36 h to several days coupled with poor nitrogen removal characteristics due to natural disasters and variation of environmental conditions [Citation6,Citation43], the pilot-scale MABR investigation in this work comprises a simplified treatment and maintenance process, with less environmental footprint, and can achieve satisfactory nitrogen removal of over 60% in one single stage. Besides, the high specific surface area feature of hollow fiber membranes in MABR (54.1 m2/m3), which facilitates stable attachment of biofilm, makes it superior to other biofilm carriers and suspended carriers based bioreactors. Moreover, the degradation of biocarriers, head losses and clogging problems leading to lower nitrogen removal performance (<60%) reveals the disadvantage of biocarriers over MABR systems [Citation10,Citation44]. Similar to MABR, membrane bioreactor (MBR) has the potential to fundamentally advance the biological treatment process by taking advantage of the rapid development in membrane manufacturing. The aeration assisted biofilm reactors could achieve high NH4+-N removal efficiency over 90% within short HRT in both MABR and MBR. Nevertheless, for TN removal in MBR operated without an anoxic reactor coupling, high amounts of residual NO3−-N is produced in the effluent during treatment of polluted surface water, unlike MABR [Citation45]. However, further work is needed to improve the nitrogen removal performance of MABR in surface waters treatment, especially in the treatment of low C/N ratio surface waters. Augmenting the influent with a biodegradable carbon source to improve the denitrification performance or introducing a reflux system is necessary for the optimization of the nitrogen removal efficiency especially in low C/N ratio surface waters.
Table 2. Comparison of different techniques for nitrogen removal from surface waters.
Conclusions
A pilot-scale MABR was designed and utilized to treat surface water for nitrogen removal. The effects of aeration parameters including intra-membrane air flow velocity and intra-membrane air pressure, hydraulic loading and C/N ratio on nitrogen removal were investigated at experimental phases. The nitrogen removal performance was analysed and the relative removal mechanism was discussed. Intra-membrane air pressure proved to be a crucial factor in controlling the bulk DO concentration and denitrification performance in the MABR system. At low intra-membrane air flow rate of 0.005 cm/min and intra-membrane air pressure of 0.01 MPa, the bulk DO could reach 0.5 mg/L at the highest denitrification rate of 0.52 g-N/(m2 day). High oxygen supply and the lack of carbon source inhibited the denitrification process at low hydraulic loading in the MABR system. Therefore, at hydraulic loading of 0.016 m3/(m2 day) with HRT 36 h, sustainable ammonium conversion and TN removal was achieved. When the influent C/N ratios were 2 and 3.5, both the metabolism of heterotrophic bacteria and the denitrification process were inhibited by lack of carbon source. However, a remarkable nitrogen removal performance with TN removal efficiency of 38.8% and 60.3% was achieved at C/N ratios of 5 and 8, respectively. Fundamental understanding of the factors that affect nitrification and denitrification will help optimize the nitrogen removal performance of a full-scale MABR system for surface water treatment with low C/N ratio. The MABR treatment process is a feasible and promising technology that would significantly contribute to the improvement of water environment quality.
Supplementary_Data.pdf
Download PDF (289.5 KB)Acknowledgments
The authors are grateful to Dr. Olusegun K. Abass (Key Laboratory of Urban Pollutant Conversion, Institute of Urban Environment, Chinese Academy of Sciences) for his advice and suggestions during this work. The authors also thank Yupei Jiao (Metabolomics Facility at Technology Center for Protein Sciences, Tsinghua University) for his contributions to the MABR system design.
Disclosure statement
No potential conflict of interest was reported by the authors.
Additional information
Funding
References
- Gilbert N. Green protests on the rise in China. Nature. 2012;488:261–262.
- Edmonds RL. China's environmental challenges. Shapiro. Cambridge, UK, and Malden, MA: Polity Press. China Quart. 2012;212:1132–1133.
- Han D, Currell MJ, Cao G. Deep challenges for China's war on water pollution. Environ Pollut. 2016;218:1222–1233.
- Sun S-P, Nàcher CPi, Merkey B, et al. Effective biological nitrogen removal treatment processes for domestic wastewaters with low C/N ratios: A review. Environ Eng Sci. 2010;27(2):111–126.
- Cao W, Wang Y, Sun L, et al. Removal of nitrogenous compounds from polluted river water by floating constructed wetlands using rice straw and ceramsite as substrates under low temperature conditions. Ecol Eng. 2016;88:77–81.
- Li X-N, Song H-L, Li W, et al. An integrated ecological floating-bed employing plant, freshwater clam and biofilm carrier for purification of eutrophic water. Ecol Eng. 2010;36(4):382–390.
- Sun L, Liu Y, Jin H. Nitrogen removal from polluted river by enhanced floating bed grown canna. Ecol Eng. 2009;35(1):135–140.
- Cao W, Zhang Y. Removal of nitrogen (N) from hypereutrophic waters by ecological floating beds (EFBs) with various substrates. Ecol Eng. 2014;62:148–152.
- Saeed T, Paul B, Afrin R, et al. Floating constructed wetland for the treatment of polluted river water: A pilot scale study on seasonal variation and shock load. Chem Eng J. 2016;287:62–73.
- Cao W, Zhang H, Wang Y, et al. Bioremediation of polluted surface water by using biofilms on filamentous bamboo. Ecol Eng. 2012;42:146–149.
- Vymazal J. The use of hybrid constructed wetlands for wastewater treatment with special attention to nitrogen removal: a review of a recent development. Water Res. 2013;47(14):4795–4811.
- Li M, Li P, Du C, et al. Pilot-scale study of an integrated membrane-aerated biofilm reactor system on urban river remediation. Ind Eng Chem Res. 2016;55(30):8373–8382.
- Lackner S, Terada A, Horn H, et al. Nitritation performance in membrane-aerated biofilm reactors differs from conventional biofilm systems. Water Res. 2010;44(20):6073–6084.
- Li P, Li M, Zhang Y, et al. The treatment of surface water with enhanced membrane-aerated biofilm reactor (MABR). Chem Eng Sci. 2016;144:267–274.
- Hou F, Li B, Xing M, et al. Surface modification of PVDF hollow fiber membrane and its application in membrane aerated biofilm reactor (MABR). Bioresource Technol. 2013;140:1–9.
- Ahmed T, Semmens MJ, Voss MA. Oxygen transfer characteristics of hollow-fiber, composite membranes. Adv Environ Res. 2004;8(3–4):637–646.
- LaPara TM, Cole AC, Shanahan JW, et al. The effects of organic carbon, ammoniacal-nitrogen, and oxygen partial pressure on the stratification of membrane-aerated biofilms. J Ind Microbiol Biotechnol. 2006;33(4):315–323.
- Timberlake DL, Strand SE, Williamson KJ. Combined aerobic heterotrophic oxidation, nitrification and denitrification in a permeable-support biofilm. Water Res. 1988;22(12):1513–1517.
- Downing LS, Nerenberg R. Total nitrogen removal in a hybrid, membrane-aerated activated sludge process. Water Res. 2008;42(14):3697–3708.
- Andrade do Canto CS, Rodrigues JA, Ratusznei SM, et al. Feasibility of nitrification/denitrification in a sequencing batch biofilm reactor with liquid circulation applied to post-treatment. Bioresource Technol. 2008;99(3):644–654.
- MABR technology increases energy efficiency. Filtration Separation. 2015;52(6):13.
- Brindle K, Stephenson T, Semmens MJ. Nitrification and oxygen utilisation in a membrane aeration bioreactor. J Membr Sci. 1998;144(1–2):197–209.
- Lackner S, Terada A, Smets BF. Heterotrophic activity compromises autotrophic nitrogen removal in membrane-aerated biofilms: results of a modeling study. Water Res. 2008;42(4–5):1102–1112.
- Hibiya K, Terada A, Tsuneda S, et al. Simultaneous nitrification and denitrification by controlling vertical and horizontal microenvironment in a membrane-aerated biofilm reactor. J Biotechnol. 2003;100(1):23–32.
- Satoh H, Ono H, Rulin B, et al. Macroscale and microscale analyses of nitrification and denitrification in biofilms attached on membrane aerated biofilm reactors. Water Res. 2004;38(6):1633–1641.
- Semmens MJ, Dahm K, Shanahan J, et al. COD and nitrogen removal by biofilms growing on gas permeable membranes. Water Res. 2003;37(18):4343–4350.
- Syron E, Semmens MJ, Casey E. Performance analysis of a pilot-scale membrane aerated biofilm reactor for the treatment of landfill leachate. Chem Eng J. 2015;273:120–129.
- Wei X, Li B, Zhao S, et al. Mixed pharmaceutical wastewater treatment by integrated membrane-aerated biofilm reactor (MABR) system–a pilot-scale study. Bioresource Technol. 2012;122:189–195.
- Wang J, Liu G-F, Lu H, et al. Biodegradation of Acid Orange 7 and its auto-oxidative decolorization product in membrane-aerated biofilm reactor. Int Biodeter Biodegr. 2012;67:73–77.
- Varrla E, Backes C, Paton KR, et al. Large-scale production of size-controlled MoS2Nanosheets by shear exfoliation. Chem Mater. 2015;27(3):1129–1139.
- Kujawa K, Klapwijk B. A method to estimate denitrification potential for predenitrification systems using NUR batch test. Water Res. 1999;33(10):2291–2300.
- Ryu H-D, Lee S-I. Comparison of 4-Stage biological aerated filter (BAF) with MLE process in nitrogen removal from low carbon-to-nitrogen wastewater. Environ Engin Sci. 2009;26(1):163–170.
- Jiao YP, Li Y, Liu QM, et al. Treatment effect of membrane- aerated biofilm reactor (MABR) on polluted surface water. Chin J Environ Eng. 2017;11(1):85–92.
- Gong Z, Yang F, Liu S, et al. Feasibility of a membrane-aerated biofilm reactor to achieve single-stage autotrophic nitrogen removal based on Anammox. Chemosphere. 2007;69(5):776–784.
- Wei X, Li B, Zhao S, et al. COD and nitrogen removal in facilitated transfer membrane-aerated biofilm reactor (FT-MABR). J Membr Sci. 2012;389:257–264.
- Terada A, Yamamoto T, Tsuneda S, et al. Sequencing batch membrane biofilm reactor for simultaneous nitrogen and phosphorus removal: novel application of membrane-aerated biofilm. Biotechnol Bioeng. 2006;94(4):730–739.
- Wang R, Terada A, Lackner S, et al. Nitritation performance and biofilm development of co- and counter-diffusion biofilm reactors: modeling and experimental comparison. Water Res. 2009;43(10):2699–2709.
- Fu Z, Yang F, Zhou F, et al. Control of COD/N ratio for nutrient removal in a modified membrane bioreactor (MBR) treating high strength wastewater. Bioresource Technol. 2009;100(1):136–141.
- Matsumoto S, Terada A, Tsuneda S. Modeling of membrane-aerated biofilm: Effects of C/N ratio, biofilm thickness and surface loading of oxygen on feasibility of simultaneous nitrification and denitrification. Biochem Eng J. 2007;37(1):98–107.
- Lin J, Zhang P, Li G, et al. Effect of COD/N ratio on nitrogen removal in a membrane-aerated biofilm reactor. Int Biodeter Biodegr. 2016;113:74–79.
- Lin J, Zhang P, Yin J, et al. Nitrogen removal performances of a polyvinylidene fluoride membrane-aerated biofilm reactor. Int Biodeter Biodegr. 2015;102:49–55.
- Sun L, Wang Z, Wei X, et al. Enhanced biological nitrogen and phosphorus removal using sequencing batch membrane-aerated biofilm reactor. Chem Eng Sci. 2015;135:559–565.
- Zhi W, Ji G. Quantitative response relationships between nitrogen transformation rates and nitrogen functional genes in a tidal flow constructed wetland under C/N ratio constraints. Water Res. 2014;64:32–41.
- Zhang S, Wang Y, He W, et al. Responses of biofilm characteristics to variations in temperature and NH4(+)-N loading in a moving-bed biofilm reactor treating micro-polluted raw water. Bioresource Technol. 2013;131:365–373.
- Li X-y, Chu HP. Membrane bioreactor for the drinking water treatment of polluted surface water supplies. Water Res. 2003;37(19):4781–4791.