ABSTRACT
The betaine aldehyde dehydrogenase (BADH) gene plays a multifunctional role in plants. It is an important factor in fragrance production, abiotic stresses and antibiotic-free selection of transgenic plants. Molecular studies have presented a new picture of this critical factor involved in abiotic stress responses via the MAPK (mitogen-activated protein kinase) signalling pathway in numerous plants. Besides BADH, glycine betaine performs an important function in plant tolerance to environmental stresses. The presence of glycine betaine can help maintain the integrity of cell membranes against unexpected environmental stresses. BADH leads to production of glycine betaine through the oxidation of betaine aldehyde. Hence, BADH is considered a key regulator for glycine betaine formation. Consequently, by providing glycine betaine as a chemical interface, there is a critical role of BADH in enhancing the tolerance in an extensive range of plants subjected to different destructive abiotic stresses. The present article reviews the significant multifunctional role of the BADH gene in various plants, and also particularly argues how this important gene plays a responsive function to different destructive abiotic stresses, and its potential use in crop improvement using advanced technologies. Consequently, cloning of more BADH genes, specially from stress-tolerant plants, discovering their responsive signalling roles under environmental stresses, and validating such candidates for their potential are very helpful, and can open new windows to generate new stress-resistant crop cultivars.
Introduction
It has been evidenced that the betaine aldehyde dehydrogenase (BADH) genes family code a diverse group of multi-functional proteins catalysing by different mechanisms [Citation1]. The research on cloning and enzyme activity of BADH in plants initially carried out in spinach [Citation2,Citation3]. Various major BADH-encoding genes were then categorised with no enzyme activity and substrate specificity data according to the homology with the spinach BADH [Citation4]. Various plant species have more than one numbered BADH gene paralogs such as rice [Citation5], spinach [Citation6], soybean [Citation7] and barley [Citation8]. Despite 75% identity of such homologs at the amino acid level, the catalytic domains are 92% similar in rice [Citation4]. However, based on the plant species, the BADH isozymes localise in different sub-cellular areas including chloroplasts, peroxisomes or the cytosol [Citation9,Citation10].
In rice, the gene for BADH1 is on the fourth chromosome, whereas that for BADH2 is on the eighth chromosome [Citation4]. Additionally, rice BADH1 and BADH2 show greater affinity (Km) and higher catalytic efficiency (kcat/Km) towards amino aldehydes, such as γ-aminobutyraldehyde (GABald) and γ-guanidinobutyraldehyde (GGBald), in comparison with betaine aldehyde [Citation11]. BADH2 appears to have higher similarity to GABald having a Km of 9 µmol/L and high kcat/km of 68 m/s in comparison with BADH1 having Km of 498 μm and kcat/km of 27 m/s. Hence, this reveals that the two enzymes which are similar based on their nucleotide sequences are different in their enzyme activities. The hexaploid wheat cultivar Cadoux possesses three orthologs of BADH1 and BADH2, whereas its progenitors possess only one ortholog. The seeds and leaves of this variety express a single homeolog of both BADH paralogs expressed at two distinct time points, 14 DPA (days post anthesis) and 30 DPA. In three other varieties, there is only expression of the ‘A’ genome homeolog [Citation9]. Interestingly, BADH in barley includes BADH1 and BADH2 isozymes that both utilise NAD (nicotinamide adenine dinucleotide) instead of NADP (nicotinamide adenine dinucleotide phosphate) as a co-enzyme [Citation12]. The first enzyme has no affinity towards betaine aldehyde; instead it appears to be specific to the amino aldehyde AP-ald as well as AB-ald [Citation8]. Similalrly, AP-aldehyde and AB-aldehyde have higher activity than betaine aldehyde in tobacco transformed with sugar beet BADH [Citation13]. Pea BADH enzymes, showing high similarity with the BADH of spinach and sugar beet, do not oxidise betaine but rather oxidise omega amino aldehydes to their associating omega-amino acids [Citation14]. Both rice BADH paralogs include the tri-peptide C-terminal repeat, SKL, targeting isozymes and peroxisomes, whereas there are no such sequences in barley. BADH1 and BADH2 are targeted to the cystol and the peroxisomes, respectively. Furthermore, BADH2 catalysis generates glycine betaine, whereas BADH1 is not able to catalyse glycine betaine formation [Citation8]. This evidence suggests that they have different functions in vivo. However, the physiological importance of the differential sub-cellular localisations of both BADHs should be further investigated [Citation15].
BADH has several functions in plants. For instance, some truncated transcripts of BADH are present in several crops [Citation16]. Such truncated transcripts may cause the accumulation of 2AP (2-acetyl-1-pyrroline), which is a key aroma compound [Citation17] (). There is a possibility that inhibition of BADH function produces 2AP-based fragrance in main crops because of the existence of BADH isozymes [Citation11]. Also, BADH, being a selectable marker, can function as an antibiotic-free selection of transformed plants. Therefore, it can be a useful alternative to antibiotic resistance genes [Citation18]. Besides such important roles, BADH is considered a gene associated with abiotic stress tolerance [Citation19]. Abiotic stresses (such as drought, osmolarity, submergence, temperature, chilling, heavy metals, ozone, wounding, ultraviolet irradiation, etc) lead to alterations in plant metabolism, including photosynthesis inhibition, reactive oxygen species (ROS) generation, membrane disorganisation, and nutrient deficiency. Subsequently, such events can result in significant changes in growth, development and productivity [Citation20]. Against such stresses, plants deploy several strategies to survive in abiotic stress conditions [Citation21] (). So far, various stress-responsive genes have been detected. The products of such important genes are categorised into two classes based on their role. The first group includes proteins engaged in signal transduction regulation and stress-inducible gene expression (protein kinase and transcription factors). The second group contains proteins that play a role in direct environmental stress tolerance [Citation22]. Stress-induced signal transduction pathways have been shown to be highly complicated and to engage a host of various responses. One of the main pathways related to stress conditions is the MAPK (mitogen-activated protein kinase) cascade linking external stimuli with different cellular reactions [Citation23]. In abiotic stress conditions, the concentration of ions, for instance Na+ and Ca2+, is enhanced inside the cell. This is detectable by MAPK, phospholipase D (PLD) and several proteins attached to the plasma membrane. MAPK and abscisic acid (ABA) signaling pathways can transduce such stress signals to the members of the sucrose non-fermenting 1-related protein kinase 2 (SnRK2) subfamily. Then, they are activated through autophosphorylation. Finally, such SnRK2s can phosphorylate and subsequently activate BADH. BADH plays a significant role in the production of glycine betaine. Glycine betaine, formerly known as trimethylglycine, is the first studied betaine and is distinguished from other betaines [Citation4]. Glycine betaine is accumulated in various bacteria, animals and plants under unfavourable environmental conditions. For instance, in response to osmotic stress, glycine betaine leads to tolerance in cells through stabilising the protein structure and adjusting the osmotic potentials in the cytoplasm to maintain the water level [Citation24,Citation25]. The role of BADH in tolerating abiotic stresses can be shown in . Yu et al. [Citation26], by heterologous expression of a BADH gene from Ammopiptanthus nanus in E. coli, validated its role in abiotic tolerance. Additionally, BADH paralogs from various plants were cloned [Citation3,Citation27] and transformed into different crops [Citation28,Citation29]. Such transgenic plants overexpressing genes in charge of betaine synthesis, accumulated higher glycine betaine levels and showed enhanced tolerance to an extensive range of abiotic stresses [Citation30,Citation31]. This encouraged us to disclose different applications of BADH gene in numerous plants, and review the current knowledge on abiotic stresses responses associated with this important gene. Additionally, future perspectives for these important research fields were discussed.
Figure 2. Plant responses employed to tackle with many important environmental stresses such as drought, cold, heat, salinity, pH and submergence. Drought and heat stresses often happen simultaneously leading to additive damages.
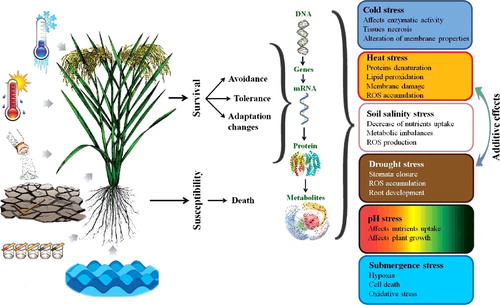
Figure 3. The function of BADH in the tolerance to abiotic stresses via the MAPK signalling pathway, and also metabolic synthesis of glycine betaine in plants (Modified from Yu et al. [Citation47]). Note: AAO, ABA-aldehyde oxidase; ABI, encoding gene for PP2C; APX, ascorbate peroxidase; CAT, catalase; CDPK, Ca2+-dependent calmodulin-independent protein kinase; CMO, choline monooxygenase; MAD, malondialdehyde; MAPK, mitogen-activated protein kinase; NAC, NAC transcription factor; NCED, 9-cis epoxycarotenoid dioxygenase gene; PA, phosphatidic acid; PLD, phospholipase D; POD, peroxidase; PP2C, protein phosphatase 2 C; REL, relative electrolyte leakage; RWC, relative water content; SnRK2, sucrose non-fermenting 1-related protein kinase 2; SOD, superoxide dismutase; WRKY, WRKY transcription factor.
![Figure 3. The function of BADH in the tolerance to abiotic stresses via the MAPK signalling pathway, and also metabolic synthesis of glycine betaine in plants (Modified from Yu et al. [Citation47]). Note: AAO, ABA-aldehyde oxidase; ABI, encoding gene for PP2C; APX, ascorbate peroxidase; CAT, catalase; CDPK, Ca2+-dependent calmodulin-independent protein kinase; CMO, choline monooxygenase; MAD, malondialdehyde; MAPK, mitogen-activated protein kinase; NAC, NAC transcription factor; NCED, 9-cis epoxycarotenoid dioxygenase gene; PA, phosphatidic acid; PLD, phospholipase D; POD, peroxidase; PP2C, protein phosphatase 2 C; REL, relative electrolyte leakage; RWC, relative water content; SnRK2, sucrose non-fermenting 1-related protein kinase 2; SOD, superoxide dismutase; WRKY, WRKY transcription factor.](/cms/asset/96b89cb0-c798-4ad7-a336-15cf80bbda4d/tbeq_a_1478748_f0003_c.jpg)
BADH in plant fragrance
Several truncated or recombinant transcripts of BADH1 and BADH2 emerging from an unusual post-transcriptional process have been found in rice (Oryza sativa), maize (Zea mays), barley (Hordeum vulgare), wheat (Triticum aestivum), etc. These events result in the insertion of exogenous gene sequences and different deletions leading to the elimination of the start codon, the loss of a functional domain and the introduction of a premature termination codon [Citation16]. Non-aromatic rice cultivars comprise a functional BADH2 gene, while aromatic rice cultivars contain a badh2 gene producing a non-functional enzyme because of a premature stop codon. Such truncated BADH2 enzyme can lead to the accumulation of 2AP, the main fragrant compound [Citation17] (). Amarawathi et al. [Citation32] could design primers from the conserved sequences that flank the 8 bp deletion in exon 7 of the gene that codes BADH2 on chromosome 8, as a reproducible marker for rice fragrance. These markers represented the role of inactive BADH2 with an 8-bp deletion in the 2AP accumulation in rice [Citation33]. However, in some varieties with no mutation, a second allele leading to loss of function of BADH2 may be in charge of the fragrance [Citation4,Citation34]. For instance, in the 10th exon of soybean BADH2, a 2-bp deletion was revealed to produce 2AP [Citation7,Citation9]. Even though 2AP formation in Bassia latifolia occurs only in flowers (fleshy corolla), in fragrant rice and plants such as Pandanus amaryllifolius and Vallaris glabra, it exists in all aerial parts [Citation35]. On the other hand, the BADH transcripts from plant species such as Arabidopsis (Arabidopsis thaliana), spinach (Spiidenacia oleracea) and tomato (Solanum lycopersicum), correctly processed the mRNA. Hence, the differentiation of post transcriptional processing in BADH genes has the potential to contribute to the variation of 2AP and the glycine betaine-synthesising capacities in different plants [Citation16].
The function of suppressive expression of BADH2 in 2AP formation has been approved by RNAi, constructed from the DNA sequence spanning exon 6 to exon 8 in the reverse direction from the related cDNA [Citation36]. Although the majority of inheritance studies have revealed that only one recessive gene, fgr (a null function gene of the BADH homologue BADH2), is the main genetic factor for 2AP synthesis in scented rice [Citation37,Citation38], numerous genes associate with the scent trait [Citation5,Citation32,Citation39,Citation40]. Based on the rice genome database for annotated functional genes, in addition to badh2 on chromosome 8, badh1 (with 92% homology of badh2) has been located on rice chromosome 4, and detected as a possible candidate gene for aroma. A functional BADH1 gene has been reported in all aromatic as well as non-aromatic rice cultivars, and its involvement in environmental stress tolerance has been proved [Citation39]. Fitzgerald et al. [Citation17] also identified another fragrance gene. They stated that the combination of the badh1 and badh2 alleles is probably the cause of high 2AP in some rice samples. Furthermore, Golestan Hashemi et al. [Citation41] comprehensively reviewed the genetic, molecular and biochemical mechanisms which result in 2AP accumulation in aromatic rice varieties.
While the aroma-responsible gene is well-characterised in rice, its orthologs in other crops are still unidentified [Citation4]. Due to the existence of BADH isozymes in major crops, it is possible that inhibition of BADH function generates 2AP-based aroma, and the desirability of this pleasant odour can be exploited in other foods. However, for having successful 2AP formation via BADH inhibition in other plants, the biochemical function of BADH should be the same as that of rice BADH2. Because BADH2 in rice is an HBH–AMADH [Citation11], 2AP production in other crop species is probably limited to those having BADHs with aminoaldehyde dehydrogenase activity [Citation4]. Hence, it seems important to identify the number of BADH homologs as well as their expression patterns in important crops, since this can determine the feasibility of replicating aroma and reveal how this can affect the crop performance. For example, so far, aroma in wheat has not been reported [Citation9].
Despite significant advancements on the biochemical and genetic bases of aroma, some issues are still ambiguous. Therefore, accumulation of further knowledge using comparative and association mapping, construction of marker-saturated maps, progressive omics approaches, detection of minor quantitative trait loci (QTLs) using chromosome segment substitution lines, and investigation of different expression quantities of the respective genes between non-fragrant and fragrant lines is required [Citation39].
BADH as an antibiotic-free selectable marker of transformants
Genetic engineering can be the best technique for introduction of favourable genes from heterologous/homologous species for improvement of agronomically valuable characteristics in various crop plants because of its benefits such as high speed, preciseness and consistency in comparison with conventional breeding that relies on stringent selection procedures for its accuracy. Genetic engineering is based on introducing foreign gene(s) into the plastid genome or plant nucleus with a selection marker gene (SMG), which is mainly either antibiotic or herbicide resistance, producing a protein that allows the growth of the transformed cells/tissues. Thus, transformants obtain a competitive benefit that can serve to identify the transformed cells from the non-transformed ones. Agrobacterium-mediated and biolistic transformation approaches have developed transgenic crops in which SMGs identify ‘true’ transformants. The popular SMG derived from prokaryotic resources poses risks when it is used in transgenic plants because of its lethal nature during the selection process. Recently, several non-lethal SMGs have been identified and utilised for selection of transformants with increased precision and high selection efficiency. Regarding the environment bio-safety issues, it seems desirable to remove the SMG for maximising the commercial success through high adoption and public acceptance of genetically modified food crops [Citation42]. However, recently, BADH as a useful alternative to antibiotic resistance genes, can be considered as a selectable marker of transgenic plants [Citation18]. For instance, BADH isolated from spinach was successfully utilised for selection of chloroplast transformation of tobacco in order to prevent the risk of transferring antibiotic resistance genes to gut microbes or the environment. Daniell et al. [Citation43] concluded that, in comparison with spectinomycin and aadA, application of 5–10 mmol/L betaine aldehyde as an antibiotic-free selection procedure of transformed plants was excellent, as calculated by the regeneration time and the transformation efficacy. Hence, BADH application as a marker for chloroplast engineering without using antibiotic can avoid transferring antibiotic genes from the plant and thus assists to allay public concern regarding genetic modifications [Citation18]. Chloroplast and plastid engineering indicates benefits for numerous applications such as agronomic features improvement [Citation44], resistance to pest and herbicide [Citation45], and vaccines and biopharmaceuticals production [Citation46]. Although there is a lack of knowledge regarding this important issue, the application of BADH for transformant selection will gain more consideration in the near future [Citation18].
BADH in abiotic stresses
Under environmental stresses, the increase of the concentration of ions (e.g. Ca2+ and Na+) can be detected by MAPK, PLD and some proteins bound to the plasma membrane. MAPK signalling pathways transduce the stress signals to the members of the SnRK2 subfamily, which are then activated via autophosphorylation. Then, such SnRK2s phosphorylate and subsequently activate BADH and ROS-scavenging enzymes, such as peroxidase (POD), catalase (CAT), superoxide dismutase (SOD), lipoxygenase (LOX), ascorbate peroxidase (APX), etc. Besides, BADH can regulate the expression of the aforementioned genes [Citation47]. Finally, BADH accelerates the oxidation of betaine aldehyde to glycine betaine [Citation4,Citation48]. In plants, chloroplasts synthesise glycine betaine by a two-step oxidation of choline via the toxic intermediate betaine aldehyde [Citation49]. The initial phase includes the particular Rieske-type iron-sulphur enzyme choline monooxygenase (CMO) [Citation50,Citation51]. A NAD-dependent BADH catalyses the next phase (). Glycine betaine, which is a kind of amino acid, functions as an osmotic protectant in plant responses to various environmental stresses. This important compound can protect the photosystem II complex, the biomembrane system, and numerous useful proteins from destructive stresses through providing osmotic adjustment. The expression of the BADH and CMO genes are greatly induced by different abiotic stresses leading to the high agglomeration of glycine betaine and consequently enhancing the tolerance to a wide range of stresses in many crops [Citation47].
BADH enzymes are from the aldehyde dehydrogenase superfamily (family 10) and are basically categorised as substrate-specific oxidoreductases (E.C. 1.2.1.8). However, several BADHs are high BADH homology aminoaldehyde dehydrogenases (HBH-AMADHs) [Citation18]. Oxidation of polyamines, natural ubiquitous aliphatic polycations, can produce aminoaldehydes by FAD (flavin adenine dinucleotide)-containing polyamine oxidases (PAOs, E.C. 1.5.3.11) and copper amine oxidases (CAOs, E.C. 1.4.3.6) (). Polyamines, found in prokaryotic and eukaryotic cells, are essential for several biological activities [Citation52,Citation53] such as cell growth, proliferation, differentiation, regulation of pathogen–host interactions and stress protective genes expression, cytoprotective and pleiotropic biochemical processes. They can also function as a ROS scavenger, acid tolerance factor and chemical chaperone [Citation54]. In plants, physiological polyamines play a main role in embryogenesis, organogenesis, floral initiation and development, leaf senescence, fruit development and ripening, and biotic and abiotic stress responses. Diamine putrescine (Put), triamine spermidine (Spd) and tetramine spermine (Spm) are the most common and abundant polyamines [Citation55]. These polyamines also regulate stomatal responses by reducing their aperture and inducing closure [Citation56]. The oxidative cleavage of Spd and Spm by PAOs forms 4-(3-aminopropylamino) butyraldehyde and 4-aminobutyraldehyde (ABAL), respectively, with the associated synthesis of H2O2 and 1,3-diaminopropane [Citation57]. 3-Aminopropionaldehyde is produced by additional oxidation of 1,3-diaminopropane. Then, this β-alanine precursor is trimethylated in order to generate the osmoprotectant β-alanine betaine. Aminoaldehydes are metabolised via NAD-dependent AMADHs (E.C. 1.2.1.19) and produces GABA. Hence, plant BADH enzymes with an AMADH activity belong to the polyamine catabolism and participate in stress reactions through GABA synthesis [Citation15]. A non-functional BADH2 instead results in 2AP formation by reacting ∆1-pyrroline with an acetyl group [Citation41,Citation58].
Drought stress
Increasing global temperature along with land desertification has resulted in reduction of soil water potentials in some areas where plants might encounter water-related stresses [Citation59]. Drought is one of the deleterious abiotic stresses limiting crop yield because several important crops (e.g. rice, potato and tobacco) are susceptible to drought [Citation30]. Thus, methods for the genetic enhancement of drought resistance in crop plants seem to be necessary for an adequate and stable food supply [Citation60]. However, breeding that attempts to produce drought tolerant rice cultivars is confined because of the complexity of establishing a standard drought stress environment and the low heritability of yield component characteristics such as relative water content, spikelet sterility, root dry weight, root pulling force and harvest index under drought stress. These traits are affected by the environment, multiple genes and the interrelation between genotype and environment, and interaction with other biotic and abiotic stresses [Citation61]. While various methods have been employed to alleviate the issue of drought [Citation62], marker-assisted selection (MAS) attempts to introgress chromosomal areas that govern tolerance to drought stress in drought-tolerant donors through precise QTL and fine mapping [Citation61]. However, the physiology and genetics of drought tolerance are very complicated, and many genes are assumed to be engaged in this trait [Citation63]. These genes are involved in osmotic adjustment, signal transduction, transcriptional regulation, deep rooting, etc [Citation60].
The low quantity of polyamines in soybean, particularly Put and Spd, was associated with the high stress injury and low water content. Both Spd and Put decreased the impacts of stress. The involvement of difluromethylarginine and alpha-difluromethyl-ornithine, and the biosynthetic inhibitors of Put, cyclohexylamine, Spd, and Spm biosynthesis were in support of polyamines in mediating the differential susceptibility of soybean and chickpea to water stress [Citation64,Citation65]. Drought stress quickly induces the PLD-mediated generation of phosphatidic acid [Citation66] and the production of ROS [Citation67,Citation68]. ROS that have been highly associated with water-deficit induced stomatal closure [Citation69,Citation70] decrease the transpiration-mediated water loss. The long-term responses of plants include the remodelling of gene expression because drought conditions usually continue for a long period of time. Such responses are mediated via transcriptionally and post-translationally regulated transcription factors involving WRKYs (superfamily of transcription factors) [Citation71] and basic leucine zipper transcription factors [Citation72]. As BADH is involved in the tolerance to abiotic stresses via the MAPK signalling pathway (), MAPK regulates the drought-induced transcriptional transactivation and the initial-to-medium-term plant responses to low water availability [Citation23]. Several studies have been carried out on the function of MAPK signalling in plant responses to drought-induced water stress. MPK9 and MPK12 respond to transformations in ABA levels. Such MAPKs are expressed in stomatal guard cells, and their activities have been associated with elicitor-mediated stomatal closure, suggesting that they regulate the stomatal movements that are induced by drought stress [Citation73–75]. Unlike MPK9 and MPK12, MPK6 is activated via the accumulation of drought-induced ROS and polyamine [Citation76]. However, it does not respond to elevated ABA levels [Citation77]. Furthermore, MKK4 over-expressors have lower rates of water loss under anhydrobiotic conditions associated with wild-type plants [Citation78]. Thus, MPK3, a down-stream target of MKK4, might develop drought-induced responses. In agriculturally-important plants, drought activates diverse MAPKs, such as OsMPK3, 4, 7, 14, 20–4 and 20–5 in rice [Citation71], GhMPK2 and GhMPK16 in cotton [Citation79] and ZmMPK3 in maize [Citation80]. Such examples show extensive crosstalk, versatility and inherent redundancy of MAPK signalling in the responses to this environmental stress [Citation59]. Various investigations indicate that the glycine betaine synthesis improves the plant tolerance in drought conditions [Citation47,Citation81]. For instance, high activity of maize BADH increased the amount of glycine betaine under drought stress [Citation82]. Also, up-regulation of BADH in Sesuvium portulacastrum was observed under low water condition [Citation19]. Overexpression of SpBADH in Arabidopsis also caused higher activity of BADH and higher glycine betaine, proline, CAT, POD, SOD contents following high tolerance to drought. Also, SpBADH raises the drought tolerance through enhancement of proline and activation of antioxidative enzymes in order to improve the ROS scavenging, as the expression of ROS-related genes (such as AtSOD, AtPOD, AtCAT, AtAPX and Atpsb) was increased by the overexpression of SpBADH in Arabidopsis under drought [Citation19]. Moreover, under drought stress, the expression of BADH was induced in the leaf, stem, and root of Suaeda corniculata [Citation83].
Soil salinity stress
Generally, saline soil has exchangeable sodium of 15%. Moreover, the electrical conductivity (E.C.) of the saturation extract (ECe) in the root zone of saline soil goes beyond 4 Ds/m (∼40 mmol/L NaCl) at 25 °C [Citation84]. Land plants are usually glycophytes and cannot tolerate Na+ concentrations beyond 50 mmol/L [Citation59,Citation85]. The combination of extreme application of fertilisers and irrigation leads to soil salinity [Citation31]. Nutrient deficiency, water deficit, ion toxicity and toxic levels of cytoplasmic sodium are due to soil salinity [Citation31,Citation86]. Therefore, salt stress is considered a major abiotic stress for plants.
Lv et al. [Citation87] reported BADH as a potential salt-responsive biomarker in Triticum monococcum. Wang et al. [Citation83] also found a novel BADH gene, ScBADH, isolated from Suaeda corniculata. They reported that ScBADH has an important function in plant salinity tolerance, and BADH proteins function as osmoregulation factors and aldehyde dehydrogenase E3 isozymes. They also observed a high amount of glycine betaine, SOD and proline in ScBADH-transformed Arabidopsis plants under NaCl treatments. In addition, in Suaeda salsa, the gene expression levels of BADH, CMO, CAT and myo-inositol-1-phosphate synthase (INPS) were upregulated under osmotic stress. Furthermore, the activities of POD, CAT, SOD and glutathione peroxidase (GPx) were increased. An increase in the betaine and allantoin levels in S. salsa seedlings and the proline, sucrose and citrate levels in the root tissues were also reported. Hence, clear osmotic stress (CMO, INPS, BADH, betaine and proline) was observed in S. salsa [Citation88]. Moreover, the salinity tolerance increased in transgenic maize expressing a BADH gene from Atriplex micrantha. Such plants agglomerated higher glycine betaine under NaCl stress [Citation89]. Also, BADH from Ammopiptanthus nanus could enhance the salinity tolerance of the plant [Citation47]. Furthermore, the BADH2 protein in barley (with high similarity to non-aromatic rice BADH2) was strongly active under salt stress conditions. Salinity also increased the BADH1 gene expression level in japonica and indica non-aromatic rice cultivars. This shows the involvement of BADH1 in salt tolerance [Citation17]. Besides, RNAi-directed knock-down of BADH1 revealed the relation between BADH1 and salt tolerance in rice plants [Citation90]. Both BADH1 and BADH2 are indicated to be in charge of rice tolerance to salinity, but, probably, at different growth periods: BADH1 at the germination and seedling stage, and BADH2 at the reproductive stage [Citation91]. Hence, BADH is a positive regulator of salt treatment via MAPK pathway in plants ().
Salt- and hyperosmosis-induced secondary messengers are able to activate diverse signalling proteins involving different members of the MAPK family, playing significant roles in the plant responses. High salt concentrations activate a Raf-like MAP3K, which is recognised as drought hypersensitive mutant 1 in rice [Citation92]. In Arabidopsis, salt concentrations are sufficient for inducing hyperosmotic response, and sodium toxicity activates MKKK20 [Citation93]. Surprisingly, mekk1 mutants of Arabidopsis show better growth at extreme salinity. Thus, unlike MKKK20, MEKK1 might be a negative regulator of the salt response [Citation94]. At the MAP2K level, it was indicated that salt stress has various impacts on a range of proteins in rice, such as OsMKK1, 3, 4, 6 and 10–2 [Citation95]. In Arabidopsis, where salinity activates MPK4 and MPK6, MKK2 is the main activator of these MAPKs, and null mkk2 mutants with impaired MKK2 function are hypersensitive to salt. However, MKK9 attempts to activate MPK3 and MPK6 [Citation96] and seems to have a negative effect on salinity tolerance because mkk9 knockout mutants show reduced salt sensitivity [Citation97]. While MKK1 as well as MKK2 are recognised as downstream targets of MEKK1 that target MPK4 and MPK6 signalling, it is unclear whether any of them shares MEKK1's proposed role as a negative regulator of salt tolerance [Citation94]. Salinity and hyperosmotic stress cause differential activation of the three main stress-related Arabidopsis MPKs, namely MPK3, MPK4 and MPK6 [Citation98]. Particularly, in the initial phases of sodium detoxification, the activation of MPK6 plays a crucial function. Sodium efflux is produced for cytosolic acidification because the SOS1 Na+/H+ antiporter is quickly phosphorylated by MPK6 [Citation76]. Moreover, MPK6 is able to regulate the transcriptional transactivation of genes expressed under salt stress via phosphorylating the zinc-finger transcription factor ZAT6 [Citation99].
On the other hand, as previously explained, BADHs having an AMADH activity belong to the polyamine catabolism and are involved in stress responses via GABA synthesis [Citation15]. A positive correlation was observed between salt tolerance and accumulation of high levels of polyamines [Citation100]. High concentrations of Spd and Spm were observed in salinity-tolerant wheat and rice varieties, whereas salinity-sensitive varieties contained a high amount of Put [Citation101,Citation102]. In addition, reduced polyamine formation led to decreased salt tolerance because of the low activity of a polyamine biosynthesis enzyme, which is arginine decarboxylase (E.C. 4.1.1.19). Zapata et al. [Citation103] measured the polyamine levels in many crop species exposed to osmotic stress. Besides beetroot, the Put concentration was lower in seedlings grown under saline conditions. However, salinity led to a substantial increase in Spd and Spm in almost all the plant species examined. Additionally, the potential function of polyamines to eliminate the destructive impact of saltiness was reported by Mutlu and Bozcuk [Citation104], who showed an increase in free, acid-soluble bound and total spermine in sunflower leaves exposed to different concentrations of NaCl. Consequently, such results show a lucid function of Spd and Spm in protecting the plasma membrane and preventing the leakage of electrolytes and amino acids from the shoots and roots of plants exposed to salinity [Citation100].
Submergence stress
Waterlogged conditions are another deleterious stress for crops. Based on the moisture/water level in the field, other alternative names for waterlogging are also flood, submergence, soil saturation, anoxia and hypoxia. Generally, two kinds of flooding occur in the field: (1) waterlogging, where some parts of the shoot and root go under water; and (2) complete submergence, in which the entire plant goes under water. In the case of deep-water flooding, the majority of the rice cultivars display a capacity of elongation of the leaves and the stem (internode). Underwater elongation can lead to plant damage and even plant death through exhausting the energy reserve of the plant. In barley, waterlogging leads to degradation of proteins, chlorophyll and RNA [Citation105]. Moreover, it decreases the amount of nutrients such as nitrogen, metal ions, phosphorus and minerals in the shoots. The attack of flood leaf chlorosis affects the shoot and root growth and leads to a notable decrease in dry matter accumulation and consequently, in the yield. Maize is also vulnerable to waterlogging causing loss of yield in tropical and subtropical areas [Citation105]. Damages of soybean due to waterlogging include necrosis, chlorosis, defoliation, stunting, reduced nitrogen fixation and finally plant death. Furthermore, waterlogging is a crucial issue for wheat cultivation worldwide and especially in the United States, where approximately 12% of the cultivated soil is influenced by excess water. The combination impact of both decreased kernel and tiller numbers is responsible for decreasing the wheat yield in waterlogging conditions [Citation105].
In plants, acetaldehyde is generated and metabolised under submerged conditions. First, pyruvate decarboxylase transforms pyruvate to acetaldehyde. Afterwards, ethanol is formed via alcohol dehydrogenase. Finally, acetate is synthesised via mitochondrial aldehyde dehydrogenase, ALDH2a and ALDH2b. On the other hand, transferring the waterlogged plants to aerobic conditions causes the anaerobically produced ethanol to be oxidised to acetaldehyde via the reverse reaction of ADH and then transformed to acetate via ALDH2a and ALDH2b as aforementioned. In addition, ethanol is oxidised to acetaldehyde via catalase (CAT) during the conversion of H2O2 to H2O in peroxisomes. However, an acetaldehyde-oxidising enzyme is unknown in peroxisomes. Finding this enzyme is a matter of interest especially for rice researchers, because low oxygen stress tolerance is of great importance for the survival of rice plants. Thus, it was assumed hat rice peroxisomal BADHs could catalyse acetaldehyde oxidation [Citation106]. In order to explore the physiological function of BADH proteins in rice, Mitsuya et al. [Citation106] examined both recombinant OsBADH1 and OsBADH2 for having affinity to acetaldehyde and to a range of substrates investigated until now for plant BADHs. They also examined the expression pattern of OsBADH genes under submergence and following re-aeration conditions. In addition, the subcellular localisation of OsBADH proteins was determined. Zingaretti et al. [Citation21] also found an increment in the sugarcane BADH expression level under water stress. Such results proved BADH modulation in water stress condition and its relation with water stress tolerance.
Temperature stress
Many plant species in areas with moderate climates sometimes have to tackle sudden temperature changes [Citation107]. Such extreme temperatures change the properties of plasma membranes. In fact, while heat increases their fluidity, cold makes them more inflexible [Citation108]. Both very low and high temperatures lead to physiological effects that make the plants unable to adapt and survive to the transient conditions [Citation107]. The mechanisms involved in cold perception are thought to include rigidification of the plasma membrane [Citation109], Ca2+ mobilisation [Citation110], activation of histidine kinase [Citation111] and generation of bioactive phosphatidic acid [Citation112] or phosphatidyl inositol [Citation113] species. The signalling pathways emerging from these diverse secondary messengers quickly converge, triggering the activation of calcium-dependent protein kinases (CDPKs) [Citation114] and MAPKs [Citation115]. Consistent with the function of BADH in the MAPK pathway in the tolerance to environmental stress conditions (), Yu et al. [Citation47] also observed that low temperature directly enhanced not only the activity of BADH, but also the amount of glycine betaine. Similarly, a higher BADH activity and glycine betaine content and a decrease in malondialdehyde (MDA) were reported in the cold-resistant wheat cultivars. Thus, the overexpression of BADH led to glycine betaine accumulation, and consequently, to increased tolerance to low temperature stress, improving the stabilisation of the membrane [Citation116]. Under cold stress, the membrane stability of potato plants was maintained well due to the production of glycine betaine. In addition, the stabilisation of the activity of the ROS scavenging enzymes (e.g. APX and SOD in potato is due to glycine betaine [Citation81].
Moreover, the signalling pathway based on MEKK1, MKK2, MPK4 and MPK6 is important in cold stress signalling [Citation59]. In Arabidopsis plants, MKK2, MPK4 and MPK6 are fundamental signalling enzymes playing a crucial role in the cold-stress responses [Citation117,Citation118]. Protein–protein interaction research based on yeast two-hybrid experiments showed interactions between MEKK1 (MAP3K), MKK2 (MAP2K) and MPK4, as well as a direct interaction between MEKK1 and MPK4 [Citation117]. Further, MPK4 may interact with the N-terminal regulatory domain of MEKK1, while MKK1 may interact with its C-terminal kinase domain [Citation119]. MEKK1 activates MKK2 and its phosphorylation activates MPK4 as well as MPK6 [Citation118,Citation120]. Hence, such proteins include a unique fully-characterised cold-activated signal transduction module that makes MEKK1 (an upstream activator) enable to activate MKK2, whose direct downstream targets are MPK4 and MPK6 [Citation118]. MKK1 was also quickly activated after cold stress, and MPK4 was determined as a downstream target of MKK1 in plants exposed to cold treatment [Citation121]. Thus, it seems that there is temporal uncoupling of cold-induced MPK4 and MPK6 activation, since MEKK1 involves two modes of signalling: the first mode is through MKK1, only transmitting the phospho-signal to MPK4, and another is through MKK2, transmitting the phospho-signal to MPK4 as well as to MPK6. The transcription of the genes coding particular members of MAPK modules might be upregulated in reaction to cold, as observed in MPK3 and MEKK1. These MAPK modules are considerable in signal transduction during cold stress [Citation59].
On the other hand, a number of complicated defence mechanisms have also been detected for preventing protein degradation under heat stress. MAPK-dependent responses to heat treatment in tomato and alfalfa have previously been explained [Citation109]. Apart from the important function of BADH in cold stress, in Sesuvium portulacastrum, SpBADH expression was up-regulated in high temperature conditions. In the roots, shoots and leaves, the accumulation of glycine betaine was induced by high temperature [Citation19]. Furthermore, the functional AnBADH gene had a key function in the adaptation to high temperature treatment [Citation47]. During heat stress in Arabidopsis, MPK6 phosphorylates HsfA2, a key high temperature stress transcription factor, on its T249 residue [Citation122]. Immunoprecipitation indicated that a direct physical interaction occurs between HsfA2 and MPK. Because T249 is located in proximity to the nuclear localisation sequence (NLS) of HsfA2, the subcellular relocation of HsfA2 from the cytoplasm to the nucleus depends on the phosphorylation of this residue. Owing to mpk6 mutants, it was revealed that MPK6 is the kinase responsible for the nucleo-cytoplasmic shuttling of HsfA2 [Citation59]. In addition, high temperature resulted in high quantities of polyamine oxidases and arginine decarboxylase induction [Citation15].
Combination of different abiotic stresses
While, in the field, plants are exposed to various abiotic stresses, and the combination of some stresses can have a very important influence on agricultural production, the co-occurrence of different stresses is rarely considered by molecular biologists studying plant acclimation [Citation123]. In the case of simultaneous exposure to stresses, a couple of signaling pathways (defence responses, osmolyte synthesis, transcription factors and hormone signals) are involved in the plant response system [Citation124]. However, not only the metabolic but also the physiological and the molecular aspects of combined stresses have to be considered to fill this gap and to accelerate the production of plants tolerant to field stress conditions [Citation125]. For instance, in drought-stricken regions, several crops face a combination of drought, heat/cold, salinity, etc. Several genes induced by cold are also induced by drought or ABA, possibly since several cold-inducible genes encode proteins for protecting the plant from freezing stress, including dehydration [Citation126]. The most examined interactions are probably the ones between various abiotic and biotic stresses. In some examples, it has been shown that particular abiotic stress condition increases the crops tolerance to a pathogen. But, if plants are continuously exposed to abiotic stress conditions, their defence systems become weak and their susceptibility to biotic stresses increases [Citation124].
Drought and heat stress also present a good case of two specific environmental stresses occurring in combination in the nature, and causing losses of $200 billion in the USA [Citation127]. Thus, many experiments have been conducted to investigate their impacts on the growth and yield of barley, sorghum, maize, etc. In comparison with each of these individual stresses, a combination of them caused a significant decrease in both the growth and the yield of such crops [Citation128]. The physiological traits of plants exposed to drought, heat or a combination of both show that the combined stress includes different effects such as high temperature of plant leaves, stomata closure, high respiration and low photosynthesis [Citation124]. Moreover, in mitochondria, starch breakdown with energy production can be influential in plant metabolism [Citation129]. On the other hand, their transcriptome profiling research supported the physiological analysis of the plants and showed that the combined stress needs a unique reaction with involvement of more than 770 transcripts unaltered by either of the two stresses alone. Moreover, same alterations in metabolite synthesis were observed, with different metabolites, basically sugars, which accumulated specially during the combined stress [Citation130]. However, sucrose replaces proline playing an important osmoprotectant role in plants against heat and drought combined stress [Citation124]. Overall, plants have various acclimation responses to heat or drought stress and a minor overlap in transcript expression has been observed between these responses. Drought and heat in combination generally show the potential harshness of a combination of stress factors, and its unique physiological, biochemical and molecular aspects [Citation125]. Contrary to the negative influence of heat and drought stresses in combination on plants, a blend of heat and salt significantly protected tomato crops against the impact of salinity alone, and protein oxidation and H2O2 agglomeration were prevented. For the particular reaction of plants to such a combination of stress factors, glycine betaine and trehalose could be effective factors. However, in Arabidopsis, the combination of heat and salinity was as deleterious as that of heat and drought. Such contradictory observations imply that the different impacts of a stress combination depend on the plant species and the genotypes, the exposure duration and the stress intensity [Citation124]. Wang et al. [Citation83] found that ScBADH regulates the plant reactions to drought and salt stresses by an ABA-independent pathway. Furthermore, the agglomeration of glycine betaine in transgenic sweet potatoes overexpressing the SoBADH gene increased the tolerance to cold, salt and oxidative stresses [Citation131]. It is also worth mentioning that the 2AP concentration increases by exposing rice plants to drought and osmotic stresses [Citation9]. Salinity has a direct effect on 2AP synthesis through stimulation of the proline metabolism [Citation132]. Furthermore, the yield is more prone to be depressed in fragrant rice in comparison with non-fragrant rice exposed to salt. In both aromatic and non-aromatic rice cultivars under salinity, the BADH1 expression and the BADH1/BADH2 ratio highly increased, proposing the role of only BADH1 in rice responses to osmotic stress [Citation17]. However, some evidence shows that the 2AP biosynthetic pathway with BADH2 is also involved in abiotic stress tolerance [Citation9]. Additionally, there are negative and positive correlations of ROS agglomeration and antioxidant volume, respectively, with plant tolerance to simultaneous stresses [Citation124].
Being tolerant to various stresses is a complicated feature including multiple pathways and cross-talks. Although mapping genes necessary for tolerance to abiotic stresses can be challenging and expensive [Citation125], detecting the genes and QTLs and clarifying their genetic relations are necessary because the complex nature of stress tolerance traits is controlled by QTLs [Citation133]. So far, plenty of stress-responsive gene-pools and QTLs have been detected at various stages of different plants using omics technologies that can be useful for the purpose of map-based cloning and identification of stress-related genes. This can open new windows to generate new stress-resistant crop cultivars.
Conclusions
Abiotic stresses have been regarded as the main causes of plant loss around the world. However, there is still a significant gap in the biochemical and molecular knowledge of the mechanisms controlling the plant responses to environmental stress, and stress-associated metabolism. Therefore, various techniques attempt to overcome the susceptibility of crops to serious environmental stresses by employing intensive molecular-assisted traditional breeding and genetic engineering. For instance, the genome-editing technology using the CRISPR/Cas9 system can quickly induce homozygous mutations in a favourable gene leading to the segregated progeny production useful to rapidly improve crop species. In addition, resistance to a combination of various stresses should be the focus of future studies that aim to develop transgenic crops tolerant to naturally occurring environments. To this end, we need to know the important and validated genes having the potential to be applied for genetic improvement of commercial crops for abiotic stress tolerance. Hence, in the present review, we introduced a very good candidate gene, BADH, and the updated and recent knowledge regarding the significant role of this positive regulator of abiotic stresses in various plants. The function and diversity of subcellular location for the tolerance to environmental stresses among the members of the BADH gene family have been reported in various plants. The concentration of ions inside the plant cell is increased when an abiotic stress occurs. It can be detected mostly by the MAPK signalling pathway. Within the MAPK pathway, BADH is activated and catalyses the synthesis of glycine betaine conferring tolerance to abiotic stresses. In this way, BADH and glycine betaine play a critical role in protecting plants under deleterious stress conditions. Thus, cloning of more BADH genes, especially from stress-tolerant plants, discovering their responsive signalling roles under environmental stresses and validating such candidates for their potential are very helpful. In addition to such a valuable function of BADH, other potentials of this gene, such as fragrance production and antibiotic-free selection of transgenic plants, were discussed.
Acknowledgement
The authors express their appreciation to Dr. Ruzbeh Babaee, Faculty of Letters, University of Porto, Portugal, for his valuable time in proofreading this article. The authors also extend their acknowledgements of Universiti Putra Malaysia and the Ministry of Higher Education, Malaysia for providing the financial support through Long-Term Research Grant Scheme (LRGS 2011–2016) for Food Security—Enhancing Sustainable Rice Production and Higher Institution Centre of Excellence, HiCOE initiative to the Institute of Tropical Agriculture and Food Security, Universiti Putra Malaysia, Malaysia. Additionally, we are thankful to Marie-Curie COFUND postdoctoral fellowship at the University of Liege, Belgium, Co-funded by the European Union.
Disclosure statement
No conflicting relationship exists for any authors.
Additional information
Funding
References
- Matin R, Ebrahimi MA, Niazi A. Quantitative expression analysis of P5CS and BADH genes in cultivated Wheat Plants under Salt and ABA treatments. Iranian J Genet Plant Breed. 2014;3:43–48.
- Pan SM, Moreau RA, Yu C, et al. Betaine accumulation and betaine-aldehyde dehydrogenase in spinach leaves. Plant Physiol. 1981;67:1105–1108.
- Weretilnyk EA, Hanson AD. Molecular cloning of a plant betaine-aldehyde dehydrogenase, an enzyme implicated in adaptation to salinity and drought. Proc Natl Acad Sci U S A. 1990;87:2745–2749.
- Fitzgerald TL, Waters DL, Henry RJ. Betaine aldehyde dehydrogenase in plants. Plant Biol. 2008;11:119–130.
- Bradbury LM, Fitzgerald TL, Henry RJ, et al. The gene for fragrance in rice. Plant Biotechnol J. 2005;3:363–370.
- Weigel P, Weretilnyk EA, Hanson AD. Betaine aldehyde oxidation by spinach chloroplasts. Plant Physiol. 1986;82:753–759.
- Arikit S, Yoshihashi T, Wanchana S, et al. Deficiency in the amino aldehyde dehydrogenase encoded by GmAMADH2, the homologue of rice Os2AP, enhances 2-acetyl-1-pyrroline biosynthesis in soybeans (Glycine max L.). Plant Biotechnol J. 2011;9:75–87.
- Fujiwara T, Hori K, Ozaki K, et al. Enzymatic characterization of peroxisomal and cytosolic betaine aldehyde dehydrogenases in barley. Physiol Plant. 2008;134:22–30.
- Shrestha K. Analysis of betaine aldehyde dehydrogenase encoding genes in wheat [MSc thesis]. Lismore (NSW): Southern Cross University; 2011.
- Wang YB, Guan LL, Xu YW, et al. Cloning and sequence analysis of the safflower betaine aldehyde dehydrogenase gene. Genet Mol Res. 2014;13:344–353.
- Bradbury LM, Gillies SA, Brushett DJ, et al. Inactivation of an aminoaldehyde dehydrogenase is responsible for fragrance in rice. Plant Mol Biol. 2008;68:439–449.
- Arakawa K, Katayama M, Takabe T. Levels of betaine and betaine aldehyde dehydrogenase activity in the green leaves, and etiolated leaves and roots of barley. Plant Cell Physiol. 1990;31:797–803.
- Trossat C, Rathinasabapathi B, Hanson AD. Transgenically expressed betaine aldehyde dehydrogenase efficiently catalyzes oxidation of dimethylsulfoniopropionaldehyde and [omega]-aminoaldehydes. Plant Physiol. 1997;113:1457–1461.
- Brauner F, Šebela M, Snégaroff J, et al. Pea seedling aminoaldehyde dehydrogenase: primary structure and active site residues. Plant Physiol Bioch. 2003;41:1–10.
- Missihoun TD, Schmitz J, Klug R. Betaine aldehyde dehydrogenase genes from Arabidopsis with different sub-cellular localization affect stress responses. Planta. 2011;233:369–382.
- Niu X, Zhang W, Lu BR, et al. An unusual posttranscriptional processing in two betaine aldehyde dehydrogenase loci of cereal crops directed by short, direct repeats in response to stress conditions. Plant Physiol. 2007;143:1929–1942.
- Fitzgerald TL, Waters DLE, Henry RJ. The effect of salt on betaine aldehyde dehydrogenase transcript levels and 2-acetyl-1-pyrroline concentration in fragrant and non-fragrant rice (Oryza sativa). Plant Sci. 2008;175:539–546.
- Fitzgerald MA, McCouch SR, Hall RD. Not just a grain of rice: the quest for quality. Trends Plant Sci. 2009;14:133–139.
- Yang C, Zhou Y, Fan J, et al. SpBADH of the halophyte Sesuvium portulacastrum strongly confers drought tolerance through ROS scavenging in transgenic Arabidopsis. Plant Physiol Bioch. 2015;96:377–387.
- Vinocur B, Altman A. Recent advances in engineering plant tolerance to abiotic stress: achievements and limitations. Curr Opin Biotech. 2006;16:123–132.
- Zingaretti S, Demore P, Morceli T, et al. Glycine betaine biosynthesis genes differentially expressed in sugarcane under water stress. BMC Proceedings. [cited 2017 Oct 29] 2014;8:P123. [2 p.] DOI:10.1186/1753-6561-8-S4-P123
- Liu H, Yu C, Li H, et al. Overexpression of ShDHN, a dehydrin gene from Solanum habrochaites enhances tolerance to multiple abiotic stresses in tomato. Plant Sci. 2015;231:198–211.
- Sinha AK, Jaggi M, Raghuram B, et al. Mitogen-activated protein kinase signaling in plants under abiotic stress. Plant Signaling Behave. 2011;6:196–203.
- Bartels D, Sunkar R. Drought and salt tolerance in plants. Crit Rev Plant Sci. 2005;24:23–58.
- Ashraf M, Foolad M. Roles of glycine betaine and proline in improving plant abiotic stress resistance. Environ Exp Bot. 2007;59:206–216.
- Yu HQ, Wang YG, Yong TM, et al. Heterologous expression of betaine aldehyde dehydrogenase gene from Ammopiptanthus nanus confers high salt and heat tolerance to Escherichia coli. Gene. 2014;549:77–84.
- Legaria J, Rajsbaum R, Munoz-Clares R, et al. Molecular characterization of two genes encoding betaine aldehyde dehydrogenase from amaranth. Expression in leaves under short-term exposure to osmotic stress or abscisic acid. Gene. 1998;218:69–76.
- Jia GX, Zhu ZQ, Chang FQ, et al. Transformation of tomato with the BADH gene from Atriplex improves salt tolerance. Plant Cell Rep. 2002;21:141–146.
- Li QL, Gao XR, Yu XH, et al. Molecular cloning and characterization of betaine aldehyde dehydrogenase gene from Suaeda liaotungensis and its use in improved tolerance to salinity in transgenic tobacco. Biotechnol Lett. 2003;25:1431–1436.
- Zhang N, Si HJ, Wen G, et al. Enhanced drought and salinity tolerance in transgenic potato plants with a BADH gene from spinach. Plant Biotechnol Rep. 2011;5:71–77.
- Yan LP, Liu CL, Liang HM, et al. Physiological responses to salt stress of T2 alfalfa progenies carrying a transgene for betaine aldehyde dehydrogenase. Plant Cell Tissue Org Cult (PCTOC). 2012;108:191–199.
- Amarawathi Y, Singh R, Singh AK, et al. Mapping of quantitative trait loci for basmati quality traits in rice (Oryza sativa L.). Mol Breed. 2008;21:49–65.
- Golestan Hashemi FS, Rafii M, Ismail MR, et al. Opportunities of marker-assisted selection for rice fragrance through marker–trait association analysis of microsatellites and gene-based markers. Plant Biol. 2015;17:953–961.
- Shi W, Yang Y, Chen S, et al. Discovery of a new fragrance allele and the development of functional markers for the breeding of fragrant rice varieties. Mol Breed. 2008;22:185–192.
- Wakte KV, Kad TD, Zanan RL, et al. Mechanism of 2-acetyl-1-pyrroline biosynthesis in Bassia latifolia Roxb. flowers. Physiol Mol Biol Plants. 2011;17:231–237.
- Gaur A, Wani S, Pandita D, et al. Understanding the fragrance in rice. J Rice Res. [cited 2017 Oct 29] 2016;4:1000e125. [4 p.] DOI:10.4172/2375-4338.1000e125
- Lorieux M, Petrov M, Huang N, et al. Aroma in rice: genetic analysis of a quantitative trait. Theor Appl Genet. 1996;93:1145–1151.
- Jin Q, Waters D, Cordeiro GM, et al. A single nucleotide polymorphism (SNP) marker linked to the fragrance gene in rice (Oryza sativa L.). Plant Sci. 2003;165:359–364.
- Golestan Hashemi FS, Rafii M, Ismail MR, et al. The genetic and molecular origin of natural variation for the fragrance trait in an elite Malaysian aromatic rice through quantitative trait loci mapping using SSR and gene-based markers. Gene. 2015;555:101–107.
- Vemireddy R, Noor S, Satyavathi V, et al. Discovery and mapping of genomic regions governing economically important traits of Basmati rice. BMC Plant Biol. [cited 2017 Oct 29] 2015;15:207. [19 p.] DOI:10.1186/s12870-015-0575-5
- Golestan Hashemi FS, Rafii M, Ismail MR, et al. Biochemical, genetic and molecular advances of fragrance characteristics in rice. Crit Rev Plant Sci. 2013;32:445–457.
- Manimaran P, Ramkumar G, Sakthivel K, et al. Suitability of non-lethal marker and marker-free systems for development of transgenic crop plants: present status and future prospects. Biotechnol Adv. 2011;29:703–714.
- Daniell H, Muthukumar B, Lee S. Marker free transgenic plants: engineering the chloroplast genome without the use of antibiotic selection. Curr Genet. 2001;39:109–116.
- Daniell H, Ruiz ON, Dhingra A. Chloroplast genetic engineering to improve agronomic traits. In: Pena L, editor. Transgenic plants: methods and protocols. Totowa (NJ): Humana Press; 2004. p. 111–137. (Methods in Molecular Biology, vol. 286).
- Bock R. Plastid biotechnology: prospects for herbicide and insect resistance, metabolic engineering and molecular farming. Curr Opin Biotech. 2007;18:100–106.
- Daniell H. Production of biopharmaceuticals and vaccines in plants via the chloroplast genome. Biotechnol J. 2006;1:1071–1079.
- Yu HQ, Zhou XY, Wang YG, et al. A betaine aldehyde dehydrogenase gene from Ammopiptanthus nanus enhances tolerance of Arabidopsis to high salt and drought stresses. Plant Growth Regul. 2017;83:265–276.
- Bao Y, Zhao R, Li F, et al. Simultaneous expression of Spinacia oleracea chloroplast choline monooxygenase (CMO) and betaine aldehyde dehydrogenase (BADH) genes contribute to dwarfism in transgenic Lolium perenne. Plant Mol Biol Rep. 2011;29:379–388.
- Takabe T, Rai V, Hibino T, Metabolic engineering of glycinebetaine. In: Rai AK, Takabe T, editors. Abiotic stress tolerance in plants. Dordrecht (Netherland): Springer; 2006. p. 137–151.
- Burnet M, Lafontaine PJ, Hanson AD. Assay, purification, and partial characterization of choline monooxygenase from spinach. Plant Physiol. 1995;108:581–588.
- Rathinasabapathi B, Burnet M, Russell BL, et al. Choline monooxygenase, an unusual iron-sulfur enzyme catalyzing the first step of glycine betaine synthesis in plants: prosthetic group characterization and cDNA cloning. Proc Natl Acad Sci U S A. 1997;94:3454–3458.
- Pál M, Szalai G, Janda T. Speculation: polyamines are important in abiotic stress signaling. Plant Sci. 2015;237:16–23.
- Sánchez-Rodríguez E, Romero L, Ruiz J. Accumulation on free polyamines enhanced antioxidant response in fruit of grafting tomato plants under water stress. J Plant Physiol. 2016;190:72–78.
- Rhee H, Kim EJ, Lee J. Physiological polyamines: simple primordial stress molecules. J Cell Mol Med. 2007;11:685–703.
- Ashraf M, Akram NA, Al-Qurainy F, et al. Drought tolerance: roles of organic osmolytes, growth regulators and mineral nutrients. Adv Agron. 2011;111:249–296.
- Alcázar R, Altabella T, Marco F, et al. Polyamine: molecules with regulatory functions in plant abiotic stress tolerance. Planta. 2010;23:1237–1249.
- Šebela M, Brauner F, Radová A, et al. Characterisation of a homogeneous plant aminoaldehyde dehydrogenase. Biochim Biophys Acta (BBA)-Protein Struct Mol Enzymol. 2000;1480:329–341.
- Mo Z, Huang J, Xiao D, et al. Supplementation of 2-Ap, Zn and La improves 2-acetyl-1-pyrroline concentrations in detached aromatic rice panicles in vitro. PloS One. [cited 2017 Oct 29] 2016;11:e0149523. [15 p.] DOI:10.1371/journal.pone.0149523
- Smékalová V, Doskočilová A, Komis G, et al. Crosstalk between secondary messengers, hormones and MAPK modules during abiotic stress signalling in plants. Biotechnol Adv. 2014;32:2–11.
- Uga Y, Yamamoto E, Kanno N, et al. A major QTL controlling deep rooting on rice chromosome 4. Sci Rep. 2013;3:3040.
- Shamsudin NAA, Swamy BM, Ratnam W, et al. Marker assisted pyramiding of drought yield QTLs into a popular Malaysian rice cultivar, MR219. BMC Genet. [cited 2017 Oct 29] 2016;17:1. [14 p.] DOI:10.1186/s12863-016-0334-0
- Ashraf M. Inducing drought tolerance in plants: recent advances. Biotechnol Adv. 2010;28:169–183.
- Jena K, Mackill D. Molecular markers and their use in marker-assisted selection in rice. Crop Sci. 2008;48:1266–1276.
- Nayyar H, Satwinder K, Kumar S, et al. Involvement of polyamines in the contrasting sensitivity of chickpea (Cicer arietinum L.) and soybean (Glycine max (L.) Merrill.) to water deficit stress. Bot Bull Acad Sinica. 2005;46:333–338.
- Groppa M, Benavides M. Polyamines and abiotic stress: recent advances. Amino Acids. 2008;34:35–45.
- Hong Y, Zhang W, Wang X. Phospholipase D and phosphatidic acid signalling in plant response to drought and salinity. Plant Cell Environ. 2010;33:627–635.
- Choudhury S, Panda P, Sahoo L, et al. Reactive oxygen species signaling in plants under abiotic stress. Plant Signaling Behav. [cited 2017 Oct 29] 2013;8:e23681. [7 p.] DOI:10.4161/psb.23681
- Yao Y, Liu X, Li Z, et al. Drought-induced H2O2 accumulation in subsidiary cells is involved in regulatory signaling of stomatal closure in maize leaves. Planta. 2013;238:217–227.
- Miura K, Okamoto H, Okuma E, et al. SIZ1 deficiency causes reduced stomatal aperture and enhanced drought tolerance via controlling salicylic acid-induced accumulation of reactive oxygen species in Arabidopsis. Plant J. 2013;73:91–104.
- Zhou XF, Jin YH, Yoo CY, et al. CYCLIN H; 1 regulates drought stress responses and blue light-induced stomatal opening by inhibiting reactive oxygen species accumulation in Arabidopsis. Plant Physiol. 2013;162:1030–1041.
- Shen H, Liu C, Zhang Y, et al. OsWRKY30 is activated by MAP kinases to confer drought tolerance in rice. Plant Mol Biol. 2010;80:241–253.
- Tang N, Zhang H, Li X, et al. Constitutive activation of transcription factor OsbZIP46 improves drought tolerance in rice. Plant Physiol. 2012;158:1755–1768.
- Jammes F, Yang X, Xiao S, et al. Two Arabidopsis guard cell-preferential MAPK genes, MPK9 and MPK12, function in biotic stress response. Plant Signaling Behav. 2011;6:1875–1877.
- Salam MA, Jammes F, Hossain MA, et al. MAP kinases, MPK9 and MPK12, regulate chitosan-induced stomatal closure. Biosci Biotech Bioch. 2012;76:1785–1787.
- Salam MA, Jammes F, Hossain MA, et al. Two guard cell-preferential MAPKs, MPK9 and MPK12, regulate YEL signalling in Arabidopsis guard cells. Plant Biol. 2013;15:436–442.
- Yu L, Nie J, Cao C, et al. Phosphatidic acid mediates salt stress response by regulation of MPK6 in Arabidopsis thaliana. New Phytol. 2010;188:762–773.
- Tsugama D, Liu S, Takano T. Drought-induced activation and rehydration-induced inactivation of MPK6 in Arabidopsis. Biochem Biophys Res Commun. 2012;426:626–629.
- Kim SH, Woo DH, Kim JM, et al. Arabidopsis MKK4 mediates osmotic-stress response via its regulation of MPK3 activity. Biochem Biophys Res Commun. 2011;412:150–154.
- Shi J, Zhang L, An H, et al. GhMPK16, a novel stress-responsive group D MAPK gene from cotton, is involved in disease resistance and drought sensitivity. BMC Mol Biol. [cited 2017 Oct 29] 2011;12:1. [15 p.] DOI:10.1186/1471-2199-12-22
- Wang J, Ding H, Zhang A, et al. A novel mitogen-activated protein kinase gene in maize (Zea mays), ZmMPK3, is involved in response to diverse environmental cues. J Integr Plant Biol. 2010;52:442–452.
- Ahmad R, Hussain J, Jamil M, et al. Glycinebetaine synthesizing transgenic potato plants exhibit enhanced tolerance to salt and cold stresses. Pak J Bot. 2014;46:1987–1993.
- Zhang L, Gao M, Hu J, et al. Modulation role of abscisic acid (ABA) on growth, water relations and glycinebetaine metabolism in two maize (Zea mays L.) cultivars under drought stress. Int J Mol Sci. 2012;13:3189–3202.
- Wang ML, Guo C, Wang N, et al. Cloning and characterization of a novel betaine aldehyde dehydrogenase gene from Suaeda corniculata. Genet Mol Res. [cited 2017 Oct 29] 2016;15:15027848. [14 p.] DOI:https://doi.org/10.4238/gmr.15027848
- Jamil A, Riaz S, Ashraf M, et al. Gene expression profiling of plants under salt stress. Crit Rev Plant Sci. 2011;30:435–458.
- Munns R, Tester M. Mechanisms of salinity tolerance. Annu Rev Plant Biol. 2008;59:651–681.
- Ward JM, Hirschi KD, Sze H. Plants pass the salt. Trends Plant Sci. 2003;8:200–201.
- Lv DW, Zhu GR, Zhu D, et al. Proteomic and phosphoproteomic analysis reveals the response and defense mechanism in leaves of diploid wheat T. monococcum under salt stress and recovery. J Proteomics. 2016;143:93–105.
- Wu H, Liu X, You L, et al. Effects of salinity on metabolic profiles, gene expressions, and antioxidant enzymes in halophyte Suaeda salsa. J Plant Growth Regul. 2012;31:332–341.
- Di H, Tian Y, Zu H, et al. Enhanced salinity tolerance in transgenic maize plants expressing a BADH gene from Atriplex micrantha. Euphytica. 2015;206:775–783.
- Tang W, Sun J, Liu J, et al. RNAi-directed downregulation of betaine aldehyde dehydrogenase 1 (OsBADH1) results in decreased stress tolerance and increased oxidative markers without affecting glycine betaine biosynthesis in rice (Oryza sativa). Plant Mol Biol. 2014;86:443–454.
- He Q, Yu J, Kim TS, et al. Resequencing reveals different domestication rate for BADH1 and BADH2 in rice (Oryza sativa). PloS One. [cited 2017 Oct 29] 2015;10:e0134801. [12 p.] DOI:10.1371/journal.pone.0134801
- Ning J, Li X, Hicks LM, et al. A Raf-like MAPKKK gene DSM1 mediates drought resistance through reactive oxygen species scavenging in rice. Plant Physiol. 2010;152:876–890.
- Kim JM, Woo DH, Kim SH, et al. Arabidopsis MKKK20 is involved in osmotic stress response via regulation of MPK6 activity. Plant Cell Rep. 2012;31:217–224.
- Su SH, Suarez-Rodriguez MC, Krysan P. Genetic interaction and phenotypic analysis of the Arabidopsis MAP kinase pathway mutations mekk1 and mpk4 suggests signaling pathway complexity. FEBS Lett. 2007;581:3171–3177.
- Kumar K, Rao KP, Sharma P, et al. Differential regulation of rice mitogen activated protein kinase kinase (MKK) by abiotic stress. Plant Physiol Bioch. 2008;46:891–897.
- Zhao Q, Guo HW. Paradigms and paradox in the ethylene signaling pathway and interaction network. Mol Plant. 2011;4:626–634.
- Xu J, Li Y, Wang Y, et al. Activation of MAPK kinase 9 induces ethylene and camalexin biosynthesis and enhances sensitivity to salt stress in Arabidopsis. J Biol Chem. 2008;283:26996–27006.
- Persak H, Pitzschke A. Tight interconnection and multi-level control of Arabidopsis MYB44 in MAPK cascade signalling. PloS One. [cited 2017 Oct 29] 2013;8:e57547. [14 p.] DOI:10.1371/journal.pone.0057547
- Liu XM, Nguyen XC, Kim KE, et al. Phosphorylation of the zinc finger transcriptional regulator ZAT6 by MPK6 regulates Arabidopsis seed germination under salt and osmotic stress. Biochem Biophys Res Commun. 2013;430:1054–1059.
- Chattopadhayay MK, Tiwari BS, Chattopadhyay G, et al. Protective role of exogenous polyamines on salinity-stressed rice (Oryza sativa) plants. Physiol. Plantarum. 2002;116:192–199.
- Basu R, Ghosh B. Polyamines in various rice (Oryza sativa) genotypes with respect to sodium chloride salinity. Physiol Plant. 1991;82:575–581.
- El-Shintinawy F. Photosynthesis in two wheat cultivars differing in salt susceptibility. Photosynthetica. 2001;38:615–620.
- Zapata PJ, Serrano M, Pretel MT, et al. Polyamines and ethylene changes during germination of different plant species under salinity. Plant Sci. 2004;167:781–788.
- Mutlu F, Bozcuk S. Effects of salinity on the contents of polyamines and some other compounds in sunflower plants differing in salt tolerance. Russ J Plant Physiol. 2005;52:29–34.
- Ahmed F, Rafii M, Ismail MR, et al. Waterlogging tolerance of crops: breeding, mechanism of tolerance, molecular approaches, and future prospects. BioMed Res Int. 2013;2013:1–10.
- Mitsuya S, Yokota Y, Fujiwara T, et al. OsBADH1 is possibly involved in acetaldehyde oxidation in rice plant peroxisomes. FEBS Lett. 2009;583:3625–3629.
- Theocharis A, Clément C, Barka EA. Physiological and molecular changes in plants grown at low temperatures. Planta. 2012;235:1091–1105.
- Mittler R, Finka A, Goloubinoff P. How do plants feel the heat ? Trends Biochem Sci. 2012;37:118–125.
- Sangwan V, Dhindsa RS. In vivo and in vitro activation of temperature-responsive plant map kinases. FEBS Lett. 2002;531:561–564.
- Zhu X, Feng Y, Liang G, et al. Aequorin-based luminescence imaging reveals stimulus-and tissue-specific Ca 2+ dynamics in Arabidopsis plants. Mol Plant. 2013;6:444–455.
- Nongpiur R, Soni P, Karan R, et al. Histidine kinases in plants: cross talk between hormone and stress responses. Plant Signaling Behav. 2012;7:1230–1237.
- Arisz SA, Wijk R, Roels W, et al. Rapid phosphatidic acid accumulation in response to low temperature stress in Arabidopsis is generated through diacylglycerol kinase. Front Plant Sci. 2013;4:1–15.
- Delage E, Ruelland E, Guillas I, et al. Arabidopsis type-III phosphatidylinositol 4-kinases beta1 and beta2 are upstream of the phospholipase C pathway triggered by cold exposure. Plant Cell Physiol. 2012;53:565–576.
- Ludwig AA, Romeis T, Jones JD. CDPK-mediated signalling pathways: specificity and cross-talk. J Exp Bot. 2004;55:181–188.
- Solanke AU, Sharma AK. Signal transduction during cold stress in plants. Physiol Mol Biol Plant. 2008;14:69–79.
- Sun Y, Fu L, Chen L, et al. Characterization of two winter wheat varieties' responses to freezing in a frigid region of the People's Republic of China. Can J Plant Sci. 2017;97:808–815.
- Ichimura K, Mizoguchi T, Yoshida R, et al. Various abiotic stresses rapidly activate Arabidopsis MAP kinases ATMPK4 and ATMPK6. Plant J. 2000;24:655–665.
- Teige M, Scheikl E, Eulgem T, et al. The MKK2 pathway mediates cold and salt stress signaling in Arabidopsis. Mol Cell. 2004;15:141–152.
- Ichimura K, Mizoguchi T, Irie K, et al. Isolation of ATMEKK1 (a MAP Kinase Kinase Kinase)-interacting proteins and analysis of a MAP kinase cascade in Arabidopsis. Biochem Biophys Res Commun. 1998;253:532–543.
- Pitzschke A, Schikora A, Hirt H. MAPK cascade signalling networks in plant defence. Curr Opin Plant Biol. 2009;12:421–426.
- Matsuoka D, Nanmori T, Sato KI, et al. Activation of AtMEK1, an Arabidopsis mitogen-activated protein kinase kinase, in vitro and in vivo: analysis of active mutants expressed in E. coli and generation of the active form in stress response in seedlings. Plant J. 2002;29:637–647.
- Evrard A, Kumar M, Lecourieux D, et al. Regulation of the heat stress response in Arabidopsis by MPK6-targeted phosphorylation of the heat stress factor HsfA2. Peer J. [cited 2017 Oct 29] 2013;1:e59. [21 p.] DOI:10.7717/peerj.59
- Singer SD, Zou J, Weselake RJ. Abiotic factors influence plant storage lipid accumulation and composition. Plant Sci. 2016;243:1–9.
- Suzuki N, Rivero RM, Shulaev V, et al. Abiotic and biotic stress combinations. New Phytol. 2014;203:32–43.
- Mittler R. Abiotic stress, the field environment and stress combination. Trends Plant Sci. 2006;11:15–19.
- Knight H, Knight MR. Abiotic stress signalling pathways: specificity and cross-talk. Trends Plant Sci. 2001;6:262–267.
- Wani SH, Kumar V, Shriram V, et al. Phytohormones and their metabolic engineering for abiotic stress tolerance in crop plants. Crop J. 2016;4:162–176.
- Wang Z, Huang B. Physiological recovery of Kentucky bluegrass from simultaneous drought and heat stress. Crop Sci. 2004;44:1729–1736.
- Rizhsky L, Liang H, Mittler R. The combined effect of drought stress and heat shock on gene expression in tobacco. Plant Physiol. 2002;130:1143–1151.
- Rizhsky L, Liang H, Shuman J, et al. When defense pathways collide. The response of Arabidopsis to a combination of drought and heat stress. Plant Physiol. 2004;134:1683–1696.
- Fan W, Zhang M, Zhang H, et al. Improved tolerance to various abiotic stresses in transgenic sweet potato (Ipomoea batatas) expressing spinach betaine aldehyde dehydrogenase. PLoS One. [cited 2017 Oct 29] 2012;7:e37344. [14 p.] DOI:10.1371/journal.pone.0037344
- Gay F, Maraval I, Roques S, et al. Effect of salinity on yield and 2-acetyl-1-pyrroline content in the grains of three fragrant rice cultivars (Oryza sativa L.) in Camargue (France). Field Crops Res. 2010;117:154–160.
- Cheng LR, Wang JM, Uzokwe V, et al. Genetic analysis of cold tolerance at seedling stage and heat tolerance at anthesis in rice (Oryza sativa L.). J Integr Agric. 2012;11:359–367.