Abstract
Acetic acid, an important by-product of L-tryptophan production by Escherichia coli, is detrimental to cell growth and tryptophan accumulation. To reduce acetic acid accumulation and further improve the production of L-tryptophan, an acetyl-coenzyme A (acetyl-CoA) synthase (ACS) with high synthetic activity is needed. In this study, E. coli ACS was found to have a superior catalytic capability compared to that of other bacterial strains. To reduce the accumulation of acetic acid by metabolic overflow, a higher expression level of ACS was obtained by promoter engineering and copy number optimisation. Furthermore, reconstruction of the tri-carboxylic acid cycle (TCA) not only enhanced the supply of phosphoenolpyruvate (PEP), which is one of the precursors required for tryptophan synthesis, but also improved bacterial growth. The final engineered strain T16 accumulated 54.6 g/L of L-tryptophan, 11.4% more than the original strain T03, with an accumulation of only 1.4 g/L acetic acid after fermentation, which reduced by 74.5% compared to T03.
Introduction
L-Tryptophan, plays a very important role in the cell, and is widely used in medicine, health care products, cosmetics, feed and other industries [Citation1,Citation2]. Its derivative 5-hydroxytryptophan can promote sleep and relieve depression [Citation3–5]. L-Tryptophan production by microbial fermentation has been extensively studied and developed due to its wide range of applications [Citation6–8]. However, the biosynthetic pathway of tryptophan is complex [Citation2,Citation9]: the glycolysis pathway, HMP pathway, shikimic acid pathway and branched acid pathway are included. Furthermore, the synthesis of tryptophan is regulated by various regulatory mechanisms, such as feedback repression, feedback inhibition and feed-forward inhibition [Citation10,Citation11] which increase the difficulty of tryptophan production. With the development of biotechnology and the proposal of new strategies, including modular metabolic engineering [Citation12,Citation13], discovery of new mutation points for removing feedback inhibition [Citation14] and cofactor engineering [Citation15], it is possible to further enhance the L-tryptophan production by Escherichia coli.
Acetic acid is the most influential by-product during tryptophan production by E. coli [Citation16,Citation17]. Metabolic imbalance is the bottleneck of tryptophan production by microbial fermentation, which causes the accumulation of acetic acid. In recent years, increasing the production of tryptophan and reducing the accumulation of the by-product acetic acid have been the focus of research. When the concentration of intracellular acetic acid exceeds 1.5 g/L, cell growth is blocked [Citation18,Citation19]. In E. coli, acetic acid can be synthesised via two pathways: pyruvate oxidase (PoxB) and acetate kinase/phosphotransacetylase (ackA-pta) [Citation20,Citation21]. The ackA-pta pathway, which is the main synthetic pathway, is a reversible reaction. Here, the reverse reaction will be activated if the acetic acid concentration is too high, and a small amount of acetic acid can be converted to acetyl-CoA. Reducing the synthesis of acetic acid is the main strategy to solve the problem of acetic acid accumulation. The absence of the PoxB pathway had no obvious effect on reducing acetic acid accumulation, indicating that PoxB is not the main route for acetic acid synthesis. The deletion of ackA-pta was found to seriously affect cell growth [Citation22]. The discovery and application of point mutations that can decrease the catalytic activity of PTA have been successfully applied in reducing the accumulation of acetic acid with no significant effect on cell growth [Citation20]. Acetyl-coenzyme A (acetyl-CoA) synthase (ACS) [Citation23,Citation24] catalyses acetic acid to synthesise acetyl-CoA via a two-step reaction, which is very sensitive to acetic acid, even if the accumulation of acetic acid is less. However, there are few reports on the application of ACS to reduce the accumulation of acetic acid during tryptophan production.
In this study, the strengthening of the acetic acid metabolic pathway to reduce the accumulation of acetic acid was the core purpose. As shown in , first, the expression of ACS from different strains was explored to determine the most suitable strain. Then, the transcriptional and expression intensity of acs gene from E. coli W3110 was optimised to determine the appropriate transcriptional level and avoid metabolic flow into the acetic acid synthesis pathway. Finally, the tri-carboxylic acid (TCA) cycle was reconstructed with the purpose of further improving cell growth. It was found that a reasonable expression of ACS could reduce acetic acid accumulation during tryptophan fermentation, and the reconstruction of TCA cycle promoted tryptophan production in cells. The final engineered strain T16 was found to accumulate 54.6 g/L of tryptophan, with a final acetic acid accumulation of only 1.4 g/L.
Materials and methods
Plasmids and strains
The strains and plasmids used in the study are listed in . E. coli DH5α was used as a cloning host for plasmid construction; E. coli T03 was used as the starting strain. Genetic modification was performed using the CRISPR/Cas9 system, and Professor Chen Tao of Tianjin University, China, provided the plasmids pREDCas9 and pGRB.
Table 1. The strains and plasmids used in the experiment.
Construction of plasmids and strains
The primers used in this study are listed in . Polymerase chain reaction (PCR) was performed using PrimeSTAR® HS DNA Polymerase (TaKaRa, Dalian, China). To construct the P1 plasmid, the ptrc99A plasmid was linearised by digesting with EcoRI and SalI restriction enzymes using the Takara QuickCut Enzyme Kit (TaKaRa). The acsA sequence was then amplified by PCR from the Bacillus subtilis 168 genome using primers. Finally, the PCR products were ligated into a linearised ptrc99A plasmid using the ClonExpress II One Step Cloning Kit (Vazyme Biotech, Nanjing, China). Chromosomal DNA manipulations were performed using the CRISPR/Cas9 system [Citation25].
Table 2. Primers used in study.
Assay of enzyme activities
Bacteria were shake-flask cultured at 37 °C for 8 h. IPTG was added once the biomass reached 0.23 mg/mL. The cells were collected by centrifugation (5000 g, 6 min, 4 °C) and washed twice with Tris/HCl buffer (50 mmol/L, pH 7.5). Then, 100 mg of wet cell pellet were resuspended in 1 mL ice-cold Tris/HCl buffer and subjected to ultrasonic homogenisation in an ice bath. After centrifugation, the supernatants were used for enzyme activity assays. ACS was assayed using the hydroxylamine method [Citation26].
Measurement of cell fluorescence intensity
The cell culture was diluted to an OD600 value of 0.2–0.6 to measure the cell fluorescence intensity. AFLF-7000 ultraviolet–visible spectrophotometer (Hitachi, Beijing, China) was used to detect cell fluorescence at an excitation wavelength of 510 nm.
Measurement of relative transcriptional level of related genes
Three independent samples were selected for RNA extraction for each culture condition, with each sample in the logarithmic phase of growth. Total RNA was extracted from collected cells using the Eastep Super Total RNA Extraction Kit (Promega, Shanghai, China). The extracted RNA was reversely transcribed (RT) into cDNA using the PrimeScript RT Master Mix (TaKaRa, Dalia, China) kit, and fluorescence quantitative RT-PCR was used to identify the transcriptional levels of different genes using the cDNA as the template. RT-PCR was performed using the SYBR® Premix Ex Taq™ II (TliRNaseH Plus; TaKaRa, Dalian, China). The primers for RT-PCR are listed in . The parameter settings for the amplification of DNA (Thermal Cycler Dice™ Real Time System III) were as follows: 95 °C for 30 s, 40 cycles of 95 °C for 5 s and 60 °C for 30 s. For the dissociation stage, the parameter settings were 95 °C for 15 s, 60 °C for 60 s and 95 °C for 15 s. The transcriptional level of 16S ribosomal RNA was used as an internal reference.
Table 3. Apparent kinetic constants determined for the ACS of the different strains.
Cultivation in shake flasks
The strains were cultured on agar slants at 37 °C for 12 h, and the resulting cells were transferred into 500 mL Erlenmeyer flasks containing 30 mL seed medium. After incubating for 10–12 h at 37 °C with orbital shaking at 200 rpm (9.6 g), the cells were transferred into fermentation medium with 10% inoculum when the biomass reached 3.8 mg/mL, and then, sequentially cultured at 37 °C with orbital shaking at 200 rpm (9.6 g) for 24 h. The pH was regulated at 7.0–7.2 by adding NH4OH (25%, v/v) during fermentation, while phenol red was used to determine any variations in pH. When the fermentation broth was continuously red, 1 mL of glucose (60%, m/v) was added to the medium.
The compositions of the media used are as follows: (a) the agar slant medium was composed of 1 g/L glucose, 10 g/L tryptone, 10 g/L beef extract, 5 g/L yeast extract, 2.5 g/L NaCl, and 25 g/L agar; (b) the seed medium used for L-tryptophan production was composed of 20 g/L glucose, 15 g/L yeast extract, 10 g/L (NH4)2SO4, 5 g/L sodium citrate, 5 g/L MgSO4·7H2O, 1.5 g/L KH2PO4, 0.015 g/L FeSO4·7H2O, 0.1 g/L vitamin B1; (c) the fermentation medium was composed of 20 g/L glucose, 1 g/L yeast extract, 4 g/L (NH4)2SO4, 2 g/L sodium citrate, 5 g/L MgSO4·7H2O, 2 g/L KH2PO4, and 0.1 g/L Fe2SO4·7H2O. The pH of both the seed and fermentation media was adjusted to 7.0 with 4 mol/L of NaOH. When necessary, 50 μg/mL of tetracycline (Tc) was added.
Cultivation in a 5 L bioreactor
The seed culture medium was the same as the fermentation medium in shake flasks. Seed cultures were prepared by transferring cells cultured on agar slant into a 5 L bioreactor (Baoxing, Shanghai, China) containing 3 L seed medium. The pH was maintained at 6.7–7.0 by adding NH4OH automatically. Dissolved oxygen was maintained at 20–30% by varying the stirrer speed and the aeration rate. The incubation temperature was 37 °C. The seed culture was finished once the biomass reached 6–8 mg/mL. Then, 500 mL of the culture broth was retained for the fed-batch cultures, which were carried out in a 5 L bioreactor containing 3 L of medium in total. The culture conditions were the same as those used for the seed cultures. Once the glucose in the medium was exhausted, 80% (m/v) glucose solution was fed into the culture broth at an appropriate rate to maintain the glucose in the fermentation medium of 0.1–5 g/L.
Production and organic acid analysis
The amino acid contents were measured by high performance liquid chromatography using an Agilent ZORBAX Eclipse AA column (4.6 mm × 150 mm, 5 µm; Agilent Technologies, Palo Alto, CA, USA) with detection at 360 nm. Acetate buffered acetonitrile was used as the mobile phase at a flow rate of 1 mL/min. Acetate was determined using HPLC with an Aminex HPX-87H column (300 mm × 7.8 mm; Bio-Rad, Hercules, CA) at 50 °C, with 50 mM H2SO4 as the mobile phase at a flow rate of 0.5 mL/min. The glucose concentration was measured using an SBA-40E biosensor (Institute of Shandong Academy of Sciences, Jinan, Shandong).
Results and discussion
Selection of ACS
E. coli, as one of the most commonly used host bacteria for genetic engineering, has been widely used in the synthesis of important substances [Citation27–29]. However, acetic acid concentration during fermentation inhibits cell growth and results in a loss of carbon. In E. coli. ACS converts acetic acid into acetyl-CoA by two irreversible steps. The first step involves the catalysis of acetic acid into acetyl-adenosine monophosphate (AMP) by ACS with the participation of adenosine-triphosphate (ATP). The second step involves the catalysis of acetyl-AMP by phosphotransacetylase into acetyl-CoA [Citation30]. Although E. coli itself is able to assimilate and utilise acetic acid, its ability is weak. Therefore, enhancing the utilisation of acetic acid by E. coli will reduce the accumulation of acetic acid and increase the utilisation rate of the carbon source. ACS is an ubiquitous basic enzyme in microorganisms that is able to directly convert acetic acid into acetyl-CoA, which then enters the central metabolic pathways, such as the TCA cycle.
In the study, to screen for ACS with a high activity and determine the effect of up-regulated ACS expression on E. coli growth, we selected three different genes of ACS from E. coli W3110 (acs), B. subtilis 168 (acsA) and Acetobacter pasteurium (acs1), respectively. The ptrc99A plasmid was used as the expression vector, and BL21 cells were used as the bacterial host for the recombinant plasmids. The apparent kinetic constants determined for the ACS of the different strains are shown in , and the growth of the strains is shown in . The ACS from B. subtilis had the highest catalytic activity; however, it also showed seriously hindered cell growth due to its expression intensity being too high. The ACS from A. pasteurium had poor catalytic activity, while the ACS from E. coli had little effect on cell growth with a higher catalytic activity. As a result, acs from E. coli was chosen for subsequent experiments.
Optimisation of ACS expression levels
Promoter engineering
In recent years, research has focused on increasing the production of tryptophan and reducing the accumulation of acetic acid. The rational optimisation of the transcription level of key genes in the tryptophan biosynthesis pathway directly affects the flow of the intracellular metabolism. Promoter engineering [Citation31,Citation32], the ribosome binding sites [Citation33], the copy gene number, σ factors [Citation34] and the introduction of point mutations [Citation35] will affect the transcription and expression level of genes, which will determine the transfer rate of intermediate metabolites.
Promoters are important cellular transcriptional regulators. The structure of the promoter affects its affinity with RNA polymerase, and thus affects the level of gene expression. By using different promoters, different transcriptional levels of the target gene can be achieved, and a more suitable transcriptional level for cell growth and metabolism can be obtained. Meanwhile, promoters can be divided into constitutive and inducible types [Citation36,Citation37]. Constitutive promoters continuously promote the transcription of target genes, while inducible promoters switch between ‘on’ and ‘off’ states to achieve flexible regulation of gene transcription.
To identify the transcription and expression level of acs, which could only act on acetic acid accumulated by metabolic overflow without affecting cell growth and L-tryptophan production, the transcriptional level of acs was optimised in this study. First, three promoters with different transcription intensity, the trc and ara promoters commonly used in E. coli and the promoters of acs itself, were selected. To determine the transcriptional capacity of the three promoters, green fluorescent protein (GFP) in the form of a reporter gene was used. Integration fragments Ptrc-gfp, Para-gfp and Pacs-gfp obtained by PCR were integrated into the ynci, araB and ynci sites of the strain T03. Strains T07, T08 and T09 were subsequently obtained and cultured in shake flask for 24 h. The cell density and fluorescence intensity were measured at 12 and 24 h. Considering that trc and ara were inducible promoters, IPTG and arabinose were added to the culture medium when biomass reached 0.23 mg/mL, and the results were shown in . The trc promoter was found to possess the highest transcriptional ability among the three promoters, and the acs promoter was the lowest. At the same time, the transcription level of gfp at 24 h was not significantly different from that at 12 h, indicating that the three promoters still played a strong role at the end of fermentation. The results indicated that the ability of three promoters to regulate genes differed greatly and different levels of acs transcription could be achieved.
Next, the three promoters of trc, ara and acs were selected to achieve different transcriptional levels. Single-copy strains T10, T11 and T12 were obtained by CRISPR/Cas9. Then, the transcriptional levels of acs in terms of cell growth and L-tryptophan and acetic acid accumulation were measured, as shown in . Since the pta and ackA played a role in determining the flow of acetyl-CoA to acetic acid, the transcriptional levels of these genes were also determined. Similar to the results of gfp expression, the transcriptional level of acs of strain T10 regulated by the trc promoter was the highest, with a nearly 80% increase compared to that of strain T03. Unexpectedly, the transcriptional level of ackA-pta also increased due to the enhancement of acs expression, which might be the reason for significantly affected cell growth. The final biomass was 32.1 mg/mL and L-tryptophan production was 8.6 g/L, which represent a 38.5% and 36.4% reduction compared to T03, respectively. However, acetic acid accumulated slowly, with a production of only 0.8 g/L after 14 h of fermentation. The strain T11, the acs of which was regulated by the promoter ara, had a better effect, and acetic acid began to accumulate at 8 h, with a final accumulation of 1.6 g/L, that is 61.9% less than strain T03. However, the L-tryptophan production of strain T11 was 11.1 g/L, only 15.2% less than strain T03; the relative acs transcriptional level of strain T13 regulated by its own promoter was 50% higher than that of strain T03, and its growth was less affected. L-tryptophan accumulation was not significantly reduced, and the final acetic acid production was 2.1 g/L.
Copy number optimisation
Copy number optimisation is another method of gene modification. Here, the number of gene copies determines the degree of cellular transcription and thus affects the distribution of metabolic flow.
To further optimise the transcriptional level of acs, different copies of acs with different promoters were also tested. On the basis of single-copy strains, double-copy strains T13, T14 and T15 were constructed, respectively. The results are shown in . Although the transcriptional level of acs in the double-copy strains increased again and the accumulation of acetic acid was slower, cell growth and L- tryptophan production decreased further, which was not suitable for the following chassis strains. Therefore, strain T11 was chosen as the starting strain for subsequent experiments.
Table 4. Results of shake flask cultivations of the different E. coli strains.
Modification of TCA cycle
The TCA cycle provides energy for cell growth [Citation38]. As such, any modification of the genes related to the TCA cycle might affect cell growth, such that changes to acetyl-CoA, the intermediate metabolite of glycolysis to TCA, had a significant effect. The up-regulation of acs expression might form the acetic acid–acetyl-CoA cycle, and cell growth might be hindered due to insufficient energy supply. To improve cell growth and obtain a more flexible regulation of the gene expression, the ara promoter was used to enhance the pathway of acetyl-CoA flowing into TCA via malic acid. In E. coli, oxaloacetic acid synthesises phosphoenol pyruvate (PEP), which is a major precursor of tryptophan synthesis, via pck [Citation39,Citation40]. As such, integration fragment Para-aceB-mdh-pck obtained by PCR was integrated into the pseudogene site yghx of strain T11 using large fragment integration [Citation25], and the strain T16 was obtained.
Cultivation in a 5 L bioreactor
To comprehensively evaluate the fermentation performance of the strains, strains T03, T11 and T16 were fermented in a 5 L bioreactor, as show in the results in . When the concentration of glucose was below 0.02 g/L, 80% glucose was added to the culture. The concentration of glucose in the medium was always below 0.02 g/L. At the same time, the inducer arabinose was added to the medium of strains T11 and T16 for 14 h. There was no significant difference between the three strains 14 h before fermentation. However, after 14 h, the transcriptional level of acs in strain T11 increased rapidly due to the addition of arabinose, which prevented the acetyl-CoA from flowing into acetic acid synthesis pathway. The cell growth was blocked and tryptophan production was reduced. At the end of fermentation, the yield of L-tryptophan was 45.5 g/L, which represented a 7.1% reduced compared to that of strain T03. Unexpectedly, strain T11 continued to grow while strain T03 declined after 28 h of fermentation. Furthermore, due to the up-regulation of aceB and mdh expression, the acetyl-CoA produced by acetic acid was able to enter the TCA cycle in time. Any cell growth-related issues were solved by a sufficient energy supply, and no decline was observed at the later stage of fermentation in strain T16, and the biomass reached 35.3 mg/mL, exceeding that of strain T03 by about 9.4%. Tryptophan production was also increased due to the precursor supply for tryptophan synthesis being strengthened. The final tryptophan production reached 54.6 g/L, which represented a 11.4% increase compared to that by strain T03. Although there was no significant change in the growth and L-tryptophan production of the strain, there was a significant variation in acetic acid production. Acetic acid was produced by strains T11 and T16 after about 8 h, compared to strain T03 which produced acetic acid from the beginning of fermentation. The final acetic acid accumulation of strains T11 and T16 was only 1.9 g/L and 1.4 g/L, respectively, which represented a 65.4% and 74.5% reduction compared to that of strain T03.
Figure 5. Cell growth (A), tryptophan yield (B) and acetic acid production (C) of the engineered strains T03, T11 and T16 after fed-batch fermentation in a 5-L bioreactor. Note: Data are average values of at least 3 experiments.
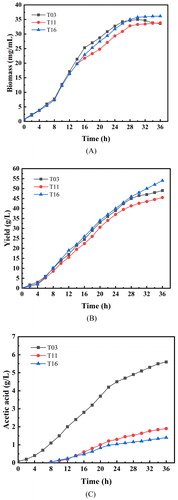
Acetic acid accumulation in E. coli can cause adverse effects on cells [Citation41], but the mechanism has not been clearly studied. In the production of tryptophan by E. coli, besides cell growth, tryptophan production, acetic acid is also a monitoring index. If acetic acid is accumulated, cell growth and tryptophan accumulation will be affected. Molecular modification is a common method to reduce the accumulation of acetic acid from the source. In the study, acetic acid accumulation was the main reason for the decrease of bacterial viability. So synthesis of acetic acid was weakened and the strain T16 was obtained. Finally, compared with that of strain T03, the acetic acid concentration of strain T16 was reduced by 4.1 g/L and kept below 1.5 g/L. Considering that there is no cell growth retardation and decreased cell viability, the growth trend of T16 was good. The results showed that the accumulation of acetic acid did cause adverse effects on cells. In addition, while acetic acid decreased by 4.1 g/L and tryptophan production increased by 5.4 g/L. Although there was no significant improvement, it successfully exceeded 50 g/L, so the strategy described in this study is promising and worth further development.
Conclusions
In this study, we successfully identified acs as the most suitable for expression in E. coli cells, and the optimal transcription level of acs in E. coli was identified by promoter engineering and copy number optimisation. The single-copy acs regulated by the inducible promoter Para met the requirement of acetic acid degradation. Secondly, the TCA cycle was remodelled to obtain a strain with more robust growth and with an enhanced supply of precursors, which was required for tryptophan production. The engineered strain T16 fermented in a 5-L fermentor was able to produce 54.6 g/L of tryptophan, which represents a 11.4% increase compared to that of the original strain T03. Furthermore, the accumulation of acetic acid was only 1.4 g/L, 74.5% less than T03.
Abbreviations | ||
acetyl-CoA | = | acetyl-coenzyme A |
ACS | = | acetyl-coenzyme A synthase |
TCA | = | tricarboxylic acid cycle |
PEP | = | phosphoenolpyruvate |
HMP | = | hexose monophosphate pathway; |
ackA | = | acetate kinase |
pta | = | phosphotransacetylase |
AMP | = | adenosine monophosphate |
ATP | = | adenosine triphosphate |
Acknowledgements
The authors thank Professor Chen Tao of Tianjin University for kindly providing the plasmids pREDCas9 and pGRB.
Disclosure statement
No potential conflict of interest was reported by the author(s).
Additional information
Funding
References
- Jing K, Tang Y, Yao C. Overproduction of L-tryptophan via simultaneous feed of glucose and anthranilic acid from recombinant E.coli W3110: kinetic modelling and process scale-up. Biotechnol Bioeng. 2018;115(2):1–11.
- Gu P, Yang F, Kang J, et al. One-step of tryptophan attenuator inactivation and promoter swapping to improve the production of L-tryptophan in Escherichia coli. Microb Cell Fact. 2012;11(1):30–39.
- Wang H, Liu W, Shi F, et al. Metabolic pathway engineering for high-level production of 5-hydroxytryptophan in Escherichia coli. Metab Eng. 2018;48(1):279–287.
- Hartmann E. Effects of L-tryptophan on sleepiness and on sleep. J Psychiatr Res. 1982;17(2):107–113.
- Farkas T, Dunner DL, Fieve RR. L-tryptophan in depression. Biol Psychiatry 1974;304(7888):1086–1086.
- Zhao ZJ, Zou C, Zhu YX, et al. Development of L-tryptophan production strains by defined genetic modification in Escherichia coli. J Ind Microbiol Biotechnol. 2011;38(12):1921–1929.
- Cheng L-K, Wang J, Xu Q-Y, et al. Effect of feeding strategy on L-tryptophan production by recombinant Escherichia coli. Ann Microbiol. 2012;62(4):1625–1634.
- Bang WG, Lang S, Sahm H, et al. Production L-tryptophan by Escherichia coli cells. Biotechnol Bioeng. 2010;25(4):999–1011.
- Lane AN, Kirschner K. The mechanism of binding of L-serine to tryptophan synthase from Escherichia coli. Eur J Biochem. 2005;129(3):561–570.
- Chen Y, Liu Y, Ding D, et al. Rational design and analysis of an Escherichia coli strain for high-efficiency tryptophan production. J Ind Microbiol Biotechnol. 2018;45(5):1–11.
- Chen L, Chen M, Ma C, et al. Discovery of feed-forward regulation in L-tryptophan biosynthesis and its use in metabolic engineering of E. coli for efficient tryptophan bioproduction. Metab Eng. 2018;47:434.
- Anil S, Prasad PR, Raj PA, et al. Modular pathway engineering for resveratrol and piceatannol production in engineered Escherichia coli. Appl Microbiol Biotechnol. 2018;102(22):9691–9706.
- Wu J, Liu P, Fan Y, et al. Multivariate modular metabolic engineering of Escherichia coli to produce resveratrol from L-tyrosine. J Biotechnol. 2013;167(4):404–411.
- Chen Z, Bommareddy RR, Frank D, et al. Deregulation of feedback inhibition of phosphoenolpyruvate carboxylase for improved lysine production in Corynebacterium glutamicum. Appl Environ Microbiol. 2014;80(4):1388–1393.
- Huang J, Wu Y, Wu W, et al. Cofactor recycling for co-production of 1,3-propanediol and glutamate by metabolically engineered Corynebacterium glutamicum. Sci Rep. 2017;7(42246):110.
- Xu Q, Fang B, Ning C, et al. Removing the by-products acetic acid and NH 4 + from the L-tryptophan broth by vacuum thin film evaporation during L-tryptophan production. Electron J Biotechnol. 2018;33(5):46–51.
- Wang J, Cheng LK, et al. Genetic engineering of Escherichia coli to enhance production of L-tryptophan. Appl Microbiol Biotechnol. 2013;97(17):7587–7596.
- Lee SY. High cell-density culture of Escherichia coli. Trends Biotechnol. 1996;14(3):98–105.
- Bauer KA, Ben-Bassat A, Dawson M, et al. Improved expression of human interleukin-2 in high-cell-density fermentor cultures of Escherichia coli K-12 by a phosphotransacetylase mutant. Appl Environ Microbiol. 1990;56(5):1296–1302.
- Lina L, Xuguo D, Jing W, et al. L-Tryptophan production in Escherichia coli improved by weakening the Pta-AckA pathway. PLOS One 2016;11(6):1–16.
- Xu Q, Fang B, Ning C, et al. Gene modification of the acetate biosynthesis pathway in Escherichia coli and implementation of the cell recycling technology to increase L-tryptophan production. PLOS One. 2017;12(6):1–18.
- Zhao C, Cheng LK, Wang J, et al. Impact of deletion of the genes encoding acetate kinase on production of L-tryptophan by Escherichia coli. Ann Microbiol. 2015;66(1):1–9.
- Chen F, Zhou J, Shi Z, et al. Effect of acetyl-CoA synthase gene overexpression on physiological function of saccharomyces cerevisiae. Wei Sheng Wu Xue Bao. 2010;50(9):1172–1179.
- Kumari S, Tishel R, Eisenbach M, et al. Cloning, characterization, and functional expression of acs, the gene which encodes acetyl coenzyme A synthetase in Escherichia coli. J Bacteriol. 1995;177(10):2878–2886.
- Heyun W, Yanjun L, Qian M, et al. Metabolic engineering of Escherichia coli for high-yield uridine production. Metab Eng. 2018;49(1):248–256.
- Brown TDK, Jones-Mortimer MC, Kornberg HL. The enzymic interconversion of acetate and acetyl-coenzyme A in Escherichia coli. J Gen Microbiol. 1977;102(2):327–336.
- Zhang X, Zhang D, Zhu J. High-yield production of L-serine from glycerol by engineered Escherichia coli. J Ind Microbiol Biotechnol. 2019;46(2):1–10.
- Li M, Chen H, Liu C, et al. Improvement of isoprene production in Escherichia coli by rational optimization of RBSs and key enzymes screening. Microb Cell Fact. 2019;18(1):1–12.
- Guo JY, Hu KL, Bi CH, et al. Construction of an alternative glycerol-utilization pathway for improved β-carotene production in Escherichia coli. J Ind Microbiol Biotechnol. 2018;45(8):697–705.
- Gordon JI, Duronio RJ, Rudnick DA, et al. Protein N-myristoylation. J Biol Chem. 1991;266(14):8647–8650.
- Guan C, Cui W, Cheng J, et al. Development of an efficient autoinducible expression system by promoter engineering in Bacillus subtilis. Microb Cell Fact. 2016;15(1):66–78.
- Xu N, Wei L, Liu J. Recent advances in the applications of promoter engineering for the optimization of metabolite biosynthesis. World J Microbiol Biotechnol. 2019;35(2):1–10.
- Shi F, Luan M, Li Y. Ribosomal binding site sequences and promoters for expressing glutamate decarboxylase and producing γ-aminobutyrate in Corynebacterium glutamicum. AMB Expr. 2018;8(1):61–78.
- Colland F, Barth M, Hengge AR, et al. σ factor selectivity of Escherichia coli RNA polymerase: role for CRP, IHF and Lrp transcription factors. EMBO J. 2000;19(12):3028–3037.
- Lin S, Meng X, Jiang J, et al. Site-directed mutagenesis and over expression of aroG gene of Escherichia coli K-12. Int J Biol Macromol. 2012;51(5):915–924.
- Wei H, Ma Y, Chen Q, et al. Identification and application of a novel strong constitutive promoter in Corynebacterium glutamicum. Ann Microbiol. 2018;68(6):375–382.
- Qin JY, Li Z, Clift KL, et al. Systematic comparison of constitutive promoters and the doxycycline-inducible promoter. Plos One. 2010;5(5):e10611–e10614. [cited 2019 Jul 29],
- Fernie AR, Carrari F, Sweetlove LJ. Respiratory metabolism: glycolysis, the TCA cycle and mitochondrial electron transport. Curr Opin Plant Biol. 2004;7(3):254–261.
- Klaffl S, Brocker M, Kalinowski J, et al. Complex regulation of the phosphoenolpyruvate carboxykinase gene pck and characterization of its GntR-type regulator IolR as a repressor of myo-inositol utilization genes in Corynebacterium glutamicum. J Bacteriol. 2013;195(18):4283–4296.
- Jian-Zhong X, Ze-Hua W, Shi-Jun G, et al. Rational modification of tricarboxylic acid cycle for improving L-lysine production in Corynebacterium glutamicum. Microb Cell Fact. 2018;17(1):105–118.
- Veeravalli K, Schindler T, Dong E, et al. Strain engineering to reduce acetate accumulation during microaerobic growth conditions in Escherichia coli. Biotechnol Prog. 2018;34(2):303–314.