Abstract
2'-Fucosyllactose (2'-FL) is one of the most important oligosaccharides in human milk. In this study, a glucose–glycerol mixed carbon source and the deletion of the genes arcA and iclR were attempted for the first time in engineered Escherichia coli to improve 2'-FL production. The highest 2'-FL titre of 5.04 g/L, almost 1.4 times higher than that in the control strain, was obtained in the arcA mutant strain using shake-flask culture. In addition, the arcA mutant showed a significantly reduced accumulation of acetate. However, the deletion of iclR and the double deletion of arcA/iclR provided no benefit in terms of 2'-FL biosynthesis. Overall, these results suggest that deletion of arcA could efficiently increase the 2'-FL yield in E. coli and could lay a foundation for more efficient and larger-scale production. Moreover, in shake-flask culture under optimal conditions, the 2'-FL titer reached a maximum value of 7.14 g/L.
Introduction
Human milk contains a class of carbohydrates with unique structure, called human milk oligosaccharides (HMOs), which are the third most abundant component in breast milk after lactose and lipids. The existence of a correlation between these human milk oligosaccharides (HMOs) and infant health is undoubtful [Citation1,Citation2]. In particular, 2′-fucosyllactose (2′-FL) is not only the most abundant but also one of the most important HMOs involved in biological functions [Citation3,Citation4]. There are several great benefits of 2′-FL supplementation in infant care; for instance, this compound can improve digestion in infants and promote infant brain development as well as exert prebiotic, antibacterial, anti-inflammatory, and antiviral effects [Citation1,Citation5]. These properties make 2′-FL an attractive molecule for nutritional and pharmaceutical applications [Citation4,Citation6,Citation7]. Moreover, 2′-FL has attracted considerable commercial interest as an additive to infant formula [Citation8]. As a result, cost-effective synthesis of 2′-FL has become a research hotspot, since the commercial promotion of this molecule reached a climax around the world [Citation9].
Currently, some approaches [Citation10] for 2′-FL production have been reported in the literature, such as chemical [Citation11–15], chemo-enzymatic [Citation16,Citation17] and enzymatic [Citation18,Citation19]. A substantial body of work has been performed on the microbial synthesis of 2′-FL, and most studies were conducted in Escherichia coli due to the simple culture conditions and availability of advanced genetic tools [Citation20,Citation21]. In our previous research, several attempts have been undertaken to improve the 2′-FL production in E. coli C41 (DE3)ΔZ () [Citation22]. Some genes mediating the biosynthesis of by-products such as colanic acid, leading to consumption of the lactose substrate, were removed [Citation23]. In addition, some other genes enhancing the biosynthesis of guanosine 5′-diphosphate (GDP)-L-fucose were either overexpressed or removed [Citation24]. Moreover, our recombinant strains use glycerol as the main carbon source. Huang et al. [Citation25] reported using glucose as the carbon source, their engineered strain accumulated more 2′-FL than that obtained using glycerol as the carbon source. However, the presence of glucose inhibited the transcription of the E. coli lac operon in a lactose-containing medium [Citation24]. Therefore, for to improve the 2′-FL yield, we use glucose and glycerol as co-carbon sources for cell growth and maintenance and 2′-FL production. As expected, shake-flash fermentations in mixed carbon sources of glucose and glycerol improved the production of 2′-FL. However, it caused the strain to produce some acetate. Acetate excretion can affect the cell density, biomass accumulation and macromolecule biosynthesis even at concentrations as low as 0.5 g/L [Citation26,Citation27]. As previously reported [Citation28], we found the similarity in our recombinant strains which produced some acetate in the biosynthesis pathway of 2′-FL [Citation22]. Here, we describe another strategy for improving 2′-FL yield, that is, to reduce the accumulation of acetate.
Figure 1. Metabolic pathways of E. coli for 2'-Fucosyllactose (2'-FL) production and microbial growth. The intracellular enzymes are abbreviated as follows: ManB, phosphomannomutase; ManC, α-D-mannose-1-phosphate guanylyltransferase; Gmd, GDP-mannose-6-dehydrogenase; WcaJ, UDP-glucose: undecaprenyl-phosphate glucose-1-phosphate transferase; LacZ, β-galactosidase; FutC, α-1,2-fucosyltransferase.
Note: The acronyms, DHA, G3P, DHAP, PEP and TCA, refer to dihydroxyacetone, glyceraldehyde 3-phosphate, dihydroxyacetone phosphate, phosphoenolpyruvate and tricarboxylic acid, respectively. Genes involved in those pathways are in italics.
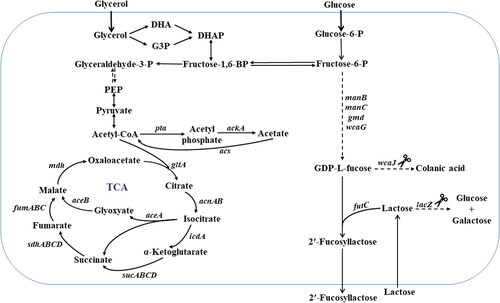
Anaerobic redox control (ArcA) is an important global regulator in E. coli. In particular, phosphorylated ArcA has an inhibitory effect on the expression of related genes (gltA, acnA, icdA, sucABCD, sdhCDAB, fumAC, mdh and aceBAK) in the TCA cycle and the glyoxylate shunt () [Citation29–32]. Knockout of arcA alleviates the repression of the TCA cycle, decreases acetate formation and enhances the expression of heterologous genes [Citation33–35]. Isocitrate lyase regulator (IclR) is a local regulator is a local regulator in E. coli. IclR are negatively regulates the gene expression of the aceBAK operon that codes for the glyoxylate pathway enzymes () [Citation36,Citation37]. The glyoxylate pathway might be activated by the knockout of iclR, resulting in a decrease in acetate formation [Citation38–40]. Acetate overflow was significantly decreased and the production of target products such as D-lactate, Poly(3-hydroxyalkanoates), 1,3-propanediol and l-threonine was increased by deleting arcA or iclR [Citation34,Citation35,Citation41–43]. The effects of single deletions of genes encoding global regulators such as ArcA have been widely studied [Citation44]. However, the outcomes of their combined knockouts have rarely been investigated, although some reports have highlighted that the combined deletion of arcA and iclR has profound effects on metabolism [Citation45–47]. It is, therefore, plausible to enhance production of 2′-FL by arcA or iclR deletion.
In this work, arcA, iclR, and arcA/iclR double mutant strains were constructed using λ Red homologous recombination [Citation48], and the production of 2′-FL in these mutant strains was investigated using shake-flask fermentation. As expected, the engineered strain with arcA mutation hardly produced acetate and presented an increase of 38.8% in the 2′-FL production compared with the control strains. The work presented here demonstrates for the first time the use of arcA deletion mutant to improve the biosynthesis of 2′-FL. Finally, to maximize the production performance of the selected high-yield strain, we systematically optimized the medium components and induction conditions. In shake-flask culture under optimal conditions, the 2′-FL titer reached a maximum value of 6.95 g/L.
Materials and methods
Bacterial strains and culture conditions
The strains used for this study are listed in . All strains were grown at 37 or 30 °C in Luria-Bertani (LB) medium (5 g/L yeast extract, 10 g/L tryptone, and 10 g/L NaCl) with rotary shaking at 230 rpm. Media were supplemented with kanamycin (50 mg/L), spectinomycin (50 mg/L), isopropyl-β-d-thiogalactopyranoside (IPTG; 0.3 mmol/L), or arabinose (10 mmol/L) when necessary.
Table 1. Strains, plasmids and primers in this study.
Gene deletions
The complete list of primers used for this study is shown in . The gene arcA was deleted from the genome of the E. coli FL-B2 strain through a λ Red recombinase protocol using the primers listed in . First, a knockout cassette (a kanamycin resistance gene flanked by FLP recognition sites and an arcA-targeting region) was generated using polymerase chain reaction (PCR) with the primers arcA-Kan-F/R, using the kanamycin resistance marker from the plasmid pKD4 as template (). Second, bacteria were transformed using electroporation with the plasmid pKD46, allowing the expression of the λ Red recombinase and the knockout cassette, and kanamycin-resistant strains were selected. Successful recombination was confirmed using PCR with check primers (arcA-DF/DR) (). Then, antibiotic resistance was removed through expression of the FLP recombinase from the pCP20 plasmid, and successful deletions were determined by loss of antibiotic resistance and using PCR with loci check primers (arcA-DF/DR) (). The resultant strain was named FL-01. Using the same method, the strain FL-02 was constructed from FL-B2 by deleting iclR, whereas FL-03 was constructed from FL-01 by deleting iclR.
Construction of recombinant plasmids
The plasmids used in this study are listed in . The pRSF-CBGW plasmid was constructed from the vector pRSF-Duet-1 to express mannose-1-phosphate guanylyltransferase (ManC, E.C. 2.7.7.22), phosphomannomutase (ManB, E.C. 5.4.2.8), GDP-D-mannose-4,6-dehydratase (Gmd, E.C. 4.2.1.47), and GDP-L-fucose synthase (WcaG, E.C. 1.1.1.271) [Citation49], whereas the pET-futC plasmid was constructed from vector pETDuet-1 to express α-1,2-fucosyltransferase (FutC, E.C 2.4.1.69) [Citation50]. The plasmids pRSF-CBGW and pET-futC were transferred by electroporation into the FL-01, FL-02 and FL-03 strains to generate the FL-011, FL-021 and FL-031 strains, respectively ().
Shake-flask cultivation of recombinant E. coli strains
The initial medium for 2′-FL production was prepared as described by Huang et al. [Citation25] with minor modifications. In brief, this medium consisted of 30 g/L glycerol, 17.9 g/L Na2HPO4·12H2O, 3.1 g/L KH2PO4, 2.0 g/L NH4Cl, 1.0 g/L (NH4)2HPO4, 1.7 g/L citric acid, 15 mg/L CaCl2, 2 g/L yeast extract, 2.2 g/L C6H5Na·2H2O, 1 g/L MgSO4·7H2O, 0.3 mL/L Triton-X 100, 10 mg/L vitamin B1 and 10 mL/L trace element solution (25 g/L FeCl3·6H2O, 2 g/L CaCl2·2H2O, 2.0 g/L ZnCl2, 1.9 g/L CuSO4·5H2O, 0.42 g/L MnCl·H2O, 2 g/L Na2B4O7·10H2O and 2 g/L Na2MoO4·2H2O). For flask fermentation, E. coli strains were streaked from frozen stocks onto LB agar plates, which were incubated overnight at 37 °C. One loop of colonies was then inoculated in 5 mL of LB medium and cultivated overnight. Next, the seed culture was transferred into a 250-mL flask containing 50 mL of LB medium at an initial cell density (OD600) of 0.1 and cultivated until it reached an OD600 of ∼0.8. Then, the culture was transferred into a 500-mL flask containing 100 mL of fermentation medium with appropriate antibiotics (100 g/mL ampicillin and 50 g/mL kanamycin) at an initial OD600 of 0.2 and cultivated at 37 °C. Finally, when the OD600 of the culture reached about 0.8, lactose and IPTG were added to a final concentration of 8 g/L and 0.3 mmol/L, respectively, and cultures were further incubated for another 96 h at 26 °C with rotary shaking (250 rpm).
Analytical methods
Growth of E. coli was monitored by measuring OD600 using an UV-1800 spectrophotometer. During fermentation, 2 mL of the culture broth was sampled at different time points. A 1-mL aliquot was boiled for 5 min and filtered for metabolite analyses. Another 1-mL aliquot was centrifuged at 17 800 × g for 10 min at 4 °C. Harvested cells were washed with 10 mL of phosphate-buffered saline (PBS; 10 mmol/L Na2HPO4·12H2O, 10 mmol/L NaH2PO4·2H2O and 500 mmol/L NaCl, pH 7.4) and centrifuged at 17 800 × g for 10 min at 4 °C. The cell pellet was dried to constant weight at 80 °C for 4 h and then the dry cell weight (DCW) was measured. The supernatant was collected and measured by using an Agilent high-performance liquid chromatography (HPLC) system (1,200 Series) equipped with an Aminex HPX-87H column (Bio-Rad) and a refractive index (RI) detector (Agilent). The column heated at 60 °C was applied to analyse 100 μL of diluted culture broth. Sulphuric acid (H2SO4) 5 mmol/L solution was used as a mobile phase at a flow rate of 0.5 mL/min. The standard curves were generated using standard 2′-FL (Carbosynth Ltd, CAS: 41263-94-9), glycerol (Solarbio, CAS: 56-81-5), lactose (Sigma, CAS: 64044-51-5), and acetate (Aladdin, CAS: 64-19-7) and a sensitive and reliable external standard HPLC method for determining the content of metabolites (2′-FL, glycerol, lactose and acetate) was set up.
Results and discussion
Effects of glucose on 2'-FL production
The main carbon source of the initial medium was glycerol (30 g/L). But glucose, as a ‘fast’ carbon source, would be more suitable for cell growth and recombinant protein expression. According to Huang et al. [Citation25], their engineered strain using glucose as a carbon source produced more 2′-FL than that using glycerol as a carbon source. However, the presence of glucose inhibited the transcription of the E. coli lac operon in a lactose-containing medium [Citation24]. Hence, different concentrations of glucose (5–25 g/L) were added to the initial fermentation medium to investigate their effects on the production of 2′-FL in the strain FL-B21 and to identify the optimal carbon source to use in combination with glycerol. As shown in , the glucose concentration obviously affected the maximum DCW and the production of 2′-FL. Indeed, increasing glucose concentrations triggered a trend of early increase and late decrease in the maximum DCW and 2′-FL production. In particular, 15 g/L of glucose showed an inhibitory effect on cell growth and 2′-FL production, whereas 20 or 25 g/L of glucose exerted stronger repression on 2′-FL production than on cell growth. The maximum DCW and 2′-FL production were 6.42 and 3.63 g/L, respectively, and were both obtained at a glucose concentration of 10 g/L. Thus, the best carbon source combination was found to consist of a mixture of 10 g/L of glucose and 30 g/L of glycerol.
Figure 2. Effect of glucose concentration on the cell mass (DCW) and 2'-FL production of recombinant E. coli FL-B21.
Note: All experiments were conducted at least thrice and the error bars represent the standard deviations.

However, as shown in , strain FL-B21 produced 1.8 g/L acetate in the initial fermentation medium supplemented with 10 g/L of glucose. As previously reported [Citation28], the strains for producing 2′-FL also produced acetate. The concentration of acetate in cells, even as low as 0.5 g/L, will affect cell density, biomass accumulation and macromolecular biosynthesis [Citation26,Citation27]. So, an attempt was made to delete arcA and iclR gene in strain FL-B21, to reduce the accumulation of acetate and boost 2′-FL production.
Table 2. Comparison of results of shake-flask fermentations of the engineered E. coli strain FL-B21 in different carbon sources.
Effects of arcA and iclR gene knockout on cell growth
The arcA, iclR and arcA/iclR double mutant E. coli strains were constructed using the λ Red recombinase protocol, transformed with recombinant plasmids, and then tested for 2′-FL production. The control strain (FL-B21) and arcA, iclR and arcA/iclR mutants (namely FL-011, FL-021 and FL-031, respectively) were cultivated in Erlenmeyer flasks at 26 °C for 96 h, after induction via addition of IPTG at a final concentration of 0.3 mmol/L. As shown in , compared with that of the control FL-B21, the maximum DCW of the mutant strains FL-021 and FL-031 was slightly increased (by 6.2% and 3.1%, respectively), whereas that of the arcA mutant FL-011 was decreased by 3.9%. Although FL-011 grew better than FL-B21 for the first 32 h of culture, it grew slower than FL-B21 later. Consistent with its growth status, FL-011 consumed glycerol faster than FL-B21 during the first 32 h of culture (). However, after 32 h, the glycerol consumption of FL-011 slowed down, resulting in a residual glycerol content slightly higher than that of FL-B21. In addition, the cell yield of each strain was also calculated. FL-011 achieved 2.06 g DCW/g glycerol, a 3.7% lower yield than that of the control strain FL-B21 (). And, the cell yields of FL-021 and FL-031 were slightly higher than that of FL-B21. These results indicate that deletion of arcA, iclR, or both did not significantly affect the growth of the E. coli cells.
Figure 3. Cell mass and substrate consumptions of FL-011, FL-021 and FL-031 strains cultivated in flasks. (A) Cell mass (DCW), (B) glycerol for FL-011, FL-021 and FL-031.
Note: All experiments were conducted at least thrice and the error bars represent the standard deviations.
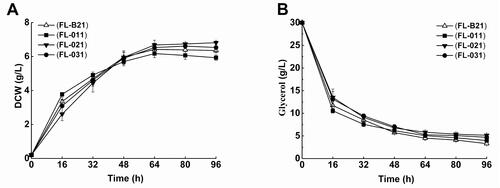
Figure 4. The effects of arcA and/or iclR knockout on the cell phycology and metabolism of recombinant E. coli strains in shake-flask cultures.
Note: The lactose conversion efficiencies, glycerol conversion efficiencies, cell yields of strains and 2'-FL production per gram of DCW. The lactose and glycerol conversion efficiencies are the mass ratio of 2'-FL production and lactose and glycerol consumption, respectively. All experiments were conducted at least thrice and the error bars represent the standard deviations.
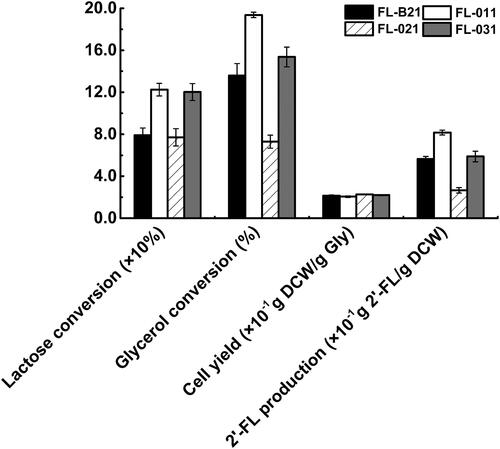
Deletion of arcA or iclR gene overcomes acetate overflow
Next, we examined the effect of arcA and iclR knockout on the production of acetate. As shown in , the control strain FL-B21 produced acetate continuously throughout the period of culture. After 96 h of cultivation, the production of acetate reached the highest yield of 1.80 g/L. In contrast, the arcA mutant FL-011, the iclR mutant FL-021, and the arcA/iclR mutant FL-031 hardly produced acetate. These results indicated that arcA and iclR mutants could dramatically decrease acetate accumulation during 2′-FL production. This decrease of acetate accumulation may be due to the increase in TCA cycle flux. Nizam et al. [Citation51] reported the effect of arcA knockout on acetate formation in E. coli K12 strain, and their results were not as significant as shown in this study. This could be a result of different host strains. In addition, as studied by Liu et al. [Citation47], the ability to overcome acetate formation of arcA-iclR double mutants was less than that of the arcA single mutants. In our study, however, arcA, iclR and arcA/iclR double mutant strains all showed reduced acetate production remarkably as compared to the control strains. The regulatory mechanisms remained unclear and needed to be resolved.
Knockout of arcA and/or iclR increases 2'-FL production
The effect of arcA, iclR and arcA/iclR knockout on 2′-FL production by E. coli FL-B21 was also investigated (). The 2′-FL yield of the arcA mutant FL-011 was 5.04 g/L, approximately 1.4 times higher than that of the control strain FL-B21. Notably, the conversion efficiency of glycerol to 2′-FL was increased by 1.4-fold by arcA knockout, from 13.6% to 19.4% (). Lactose is another substrate for the production of 2′-FL. Similarly, upon arcA knockout, the efficiency of lactose conversion to 2′-FL also increased by 1.6-fold, from 79.2% to 122.5%. These data suggest that knockout of arcA significantly improved the production of 2′-FL as well as the glycerol and lactose conversion efficiency. Moreover, it is speculated that the genetic modification of arcA knockout could result in a highly efficient production of 2′-FL and reduce the manufacturing cost in industrial applications. The engineering design of global regulatory agencies may show phenotypes and changes in carbon fluxes and metabolic pathways. It is hard to determine which particular genes or pathways were affected by the knockout of the global regulator ArcA. So, at present, the regulatory mechanisms of global regulator ArcA are unclear and need to be further explored.
On the contrary, compared with that in the control FL-B21, 2′-FL production sharply decreased in the iclR mutant FL-021. Indeed, only 1.81 g/L of 2′-FL was accumulated by FL-021 (), and the conversion efficiency of glycerol and lactose to 2′-FL were also decreased by 63.7% and 37.1%, respectively (). In addition, the arcA/iclR mutant FL-031 produced 3.89 g/L of 2′-FL, a 114.9% higher yield than that observed in FL-021, but 22.8% lower than that observed in FL-011 (). The conversion efficiency of glycerol and lactose to 2′-FL were increased by 110.4% and 56.0%, respectively, compared with those of FL-021 (). These results further indicate that deletion of arcA could improve the production of 2′-FL and the conversion efficiency of glycerol and lactose to 2′-FL, while the deletion of iclR resulted in no benefit for the biosynthesis of 2′-FL. On the one hand, the maximum DCW of the iclR mutant increased by 6.2%, which may affect the cell physiology and metabolism. On the other hand, the deletion of iclR may promote the growth of the cells instead of the biosynthesis of 2′-FL, but the molecular mechanisms are not clear completely.
Besides, compared with FL-B21, FL-011 displayed an enhanced 2′-FL production per gram of DCW, which increased by 0.5-fold, from 0.56 to 0.82 g 2′-FL/g DCW (). Furthermore, compared with FL-021, FL-031 showed an enhanced 2′-FL production per gram of DCW, which increased by 1.2-fold, from 0.27 to 0.59 g 2′-FL/g DCW (). These data show that deletion of arcA led to improved 2′-FL production per gram of DCW. Therefore, the arcA mutant FL-011 clearly contributed to the production of 2′-FL and was selected for further study.
Optimization of culture medium compositions
The optimization of culture medium composition can effectively enhance cell density and the expression of recombinant proteins in E. coli and consequently increase the yield of target products [Citation52]. Therefore, the effects of different culture medium compositions on the production of 2′-FL by FL-011 were investigated with respect to the original fermentation medium.
Effect of phosphate on 2'-FL production
Phosphate, one of the crucial elements for E. coli growth and metabolism, is essential for the growth of cells [Citation53]. In the initial fermentation medium, phosphate was supplied in the form of Na2HPO4·12H2O, KH2PO4, and (NH4)2HPO4, among which Na2HPO4·12H2O was the most concentrated compound at 50 mmol/L. To study the effect of phosphate on 2′-FL production, the concentration of Na2HPO4·12H2O was changed through a series of small increments within the range of 20–200 mmol/L. As indicated in , when the Na2HPO4·12H2O concentration increased from 0 to 50 mmol/L, the production of 2′-FL was enhanced from 2.01 to 5.04 g/L and the final OD600 increased from 14.04 to 17.00. However, with concentrations higher than 50 mmol/L, both the production of 2′-FL and OD600 gradually decreased. Therefore, Na2HPO4·12H2O significantly affected the cell growth and 2′-FL production of E. coli. For shake-flask culture, the optimum Na2HPO4·12H2O concentration was found to be 50 mmol/L, corresponding to the concentration of the original medium.
Figure 6. Effect of different concentrations of (A) phosphate, (B) Oxoid yeast extract, (C) fish peptone and (D) Oxoid tryptone on the cell density and 2'-FL production of recombinant E. coli FL-011.
Note: Culture was carried out in 500 mL shake flask containing 100 mL medium with different concentration of phosphate, Oxoid yeast extract, fish peptone and Oxoid tryptone at 230 rpm and 37 °C and was induced with 0.3 mmol/L IPTG at 26 °C for 96 h. The OD600 value was obtained by diluting the sample to OD < 1 and multiplying by the corresponding dilution factor. All experiments were conducted at least thrice and the error bars represent the standard deviations.
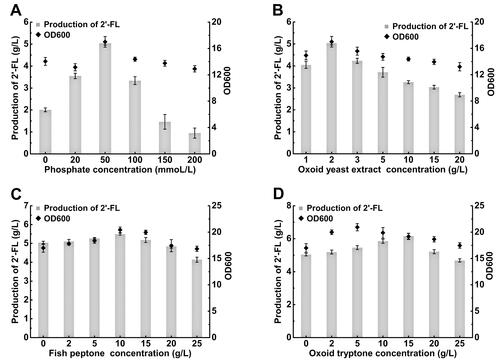
Effect of nitrogen sources on 2'-FL production
During the growth of recombinant E. coli, the nitrogen and carbon sources in the fermentation medium act as pivotal elements in the synthesis of the recombinant product. The nitrogen source is one of the most important components of microbial culture media, since nitrogen is mainly used for the synthesis of cell macromolecules, such as proteins and nucleic acids, as well as of some other nitrogen-containing metabolites. Nitrogen sources include organic and inorganic sources. It is acknowledged that proper combinations of organic and inorganic nitrogen sources can promote cell growth and enhance the expression of recombinant proteins [Citation54].
The original fermentation medium contained Oxoid yeast extract as an organic nitrogen source and NH4Cl and (NH4)2HPO4 as inorganic nitrogen sources. Thus, at first, different concentrations of Oxoid yeast extract were used to investigate their effects on 2′-FL production. The results are shown in . The highest OD600 of FL-011 (16.99) was reached at an Oxoid yeast extract concentration of 2 g/L. Simultaneously, the highest 2′-FL production (5.04 g/L) also occurred at this Oxoid yeast extract concentration. Therefore, the optimum Oxoid yeast extract concentration was determined as 2 g/L, corresponding to the concentration of the original shake-flask medium.
Next, various concentrations of organic nitrogen sources such as Oxoid tryptone and fish peptone were added to the fermentation medium. The results are shown in . The highest 2′-FL production (5.49 g/L) and OD600 (20.43) were obtained at a fish peptone concentration of 10 g/L. The highest OD600 (20.92) of FL-011 was achieved at an Oxoid tryptone concentration of 5 g/L. However, such high OD600 did not lead to high 2′-FL production. The highest 2′-FL production (6.17 g/L) appeared at an Oxoid tryptone concentration of 15 g/L. Therefore, Oxoid tryptone may be the most suitable nitrogen source for 2′-FL production because it led to higher 2′-FL yields (5.85 and 6.17 g/L, respectively) than those triggered by fish peptone (5.49 and 5.18 g/L, respectively) at the same concentrations (10 and 15 g/L).
Optimization of induction conditions
In addition to the culture medium composition, the conditions that induce recombinant protein production by E. coli also influence cell growth and synthesis of target products. Therefore, the induction conditions, such as initial induction OD600, induction temperature and inducer concentration, were optimized systematically.
Temperature is not only one of the most important factors associated with the growth and reproduction of microorganisms, but also the key factor affecting the expression of recombinant proteins. Thus, its influence on the production of 2′-FL by E. coli FL-011 was evaluated at five different induction temperatures (24, 26, 28, 30 and 32 °C). The results () showed that neither the highest nor the lowest induction temperatures tested promoted 2′-FL production or cell growth in the recombinant strain FL-011. However, among the intermediate temperatures tested, it was observed that, with the increase in the induction temperature, OD600 and 2′-FL production was first increased and then decreased. The highest OD600 (19.41) and 2′-FL production (6.58 g/L) were obtained at an induction temperature of 28 °C.
Figure 7. Effect of different induction temperatures and IPTG on the cell density and 2'-FL production of recombinant E. coli FL-011.
Note: Culture was carried out in 500 mL shake flask containing 100 mL medium at 230 rpm and 37 °C and was induced with 0.3 mmol/L IPTG at five different induction temperatures (24, 26, 28, 30, 32 °C) for 96 h and was induced with different IPTG concentrations at 28 °C for 96 h. The OD600 value was obtained by diluting the sample to OD < 1 and multiplying by the corresponding dilution factor. All experiments were conducted at least thrice and the error bars represent the standard deviations.
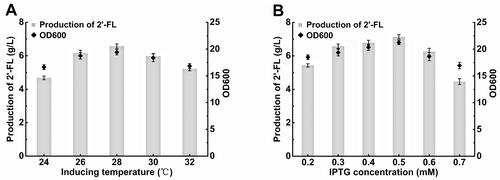
The lac promoter and its derivatives are widely used in the expression of exogenous genes. IPTG can inhibit the repression of the lac promoter; therefore, the level of recombinant proteins is regulated by the concentration of IPTG in the medium. Considering the high cost of IPTG, lactose is often a valid substitute for this inducer to induce protein expression [Citation55]. However, lactose can be used not only as an inducer but also as a carbon source and a substrate in the process of 2′-FL production. Thus, using lactose as an inducer is more complex than using IPTG. Therefore, the induction of protein expression for 2′-FL biosynthesis by FL-011 was conducted at various concentrations of lactose (8–130 g/L). The results showed that the yields of 2′-FL were between 0.2 and 0.7 g/L when lactose alone was supplied as an inducer (data not shown). Therefore, lactose cannot be used as an effective inducer to achieve high yields in strain FL-011. This could be because lactose is the carbon source for cell metabolism and the substrate for 2′-FL production. Its concentration changes dynamically. So, there is not enough lactose in the cell as an inducer. On the contrary, IPTG, as an inducer, is not degraded and metabolized in E. coli and has a long-lasting induction effect.
Consequently, different concentrations of IPTG (0.2–0.7 mmol/L) were used to investigate their effects on the production of 2′-FL. The results are shown in . The highest 2′-FL production (7.14 g/L) and OD600 (21.24) were obtained at an IPTG concentration of 0.5 mmol/L. Since IPTG is toxic to bacteria and at high concentrations it may even kill cells, IPTG concentrations of 0.6 and 0.7 mmol/L inhibited the growth of the strain and reduced the 2′-FL yield. Furthermore, the 2′-FL production by strain FL-011 was induced at different initial induction OD600 values (0.5, 0.7, 0.9, 1.1 and 1.3). The production of 2′-FL was almost the same at all induction OD600 tested (data not shown).
Based on the above optimization, compared with the initial culture conditions, the optimum fermentation medium was supplemented with 10 g/L glucose and 15 g/L Oxoid tryptone. The optimum concentration of the inducer IPTG was increased from 0.3 to 0.5 mmol/L and the optimum induction temperature was enhanced from 26 to 28 °C. Finally, the optimum culture conditions for 2′-FL production were as follows: medium supplemented with 30 g/L glycerol, 10 g/L glucose, 2 g/L yeast extract, 15 g/L Oxoid tryptone, 17.9 g/L Na2HPO4·12H2O, 3.1 g/L KH2PO4, 2.0 g/L NH4Cl, 1.0 g/L (NH4)2HPO4, 1.7 g/L citric acid, 15 mg/L CaCl2, 2.2 g/L C6H5Na·2H2O, 1 g/L MgSO4·7H2O, 0.3 mL/L Triton-X 100, 10 mg/L vitamin B1 and 10 mL/L trace element solution (25 g/L FeCl3·6H2O, 2 g/L CaCl2·2H2O, 2.0 g/L ZnCl2, 1.9 g/L CuSO4·5H2O, 0.42 g/L MnCl·H2O, 2 g/L Na2B4O7·10H2O and 2 g/L Na2MoO4·2H2O); induction with 0.5 mmol/L IPTG at an initial induction OD600 of 0.7–0.9; and growth at 28 °C for 96 h. Cultivation in shake flasks was performed in the optimized medium under the optimized induction conditions and resulted in a high 2′-FL production of 7.14 g/L. Such yield was 1.42 times higher than that (5.04 g/L) before optimization.
The production levels of 2′-FL in shake-flask fermentation in previous studies were compared in [Citation23,Citation28,Citation56–59]. Currently, the highest yield of 2′-FL in shake-flask fermentation is 15.4 g/L [Citation28]. The difference from this study is that the strain uses WcfB instead of FutC as an α-1,2-fucosyltransferase. In the reports using FutC as the α-1,2-fucosyltransferase for production of 2′-FL, the highest reported yields were about 6-7 g/L [Citation20,Citation23,Citation28,Citation57–59]. Our engineered strains with a deletion of the arcA gene used a mixed carbon source of glucose and glycerol and under optimized fermentation conditions reached high 2′-FL production of 7.14 g/L. 2′-FL, being one of most abundant oligosaccharides in human milk, is involved in many biological functions beneficial for infant health. 2′-FL has a great potential in applications for functional food materials and pharmaceuticals.
Table 3. The reported bio-production of 2'-FL in shake-flask fermentation.
Conclusions
In this work, we first used glucose and glycerol as co-carbon sources to improve 2′-FL production from 2.67 to 3.62 g/L. Then, the effect of the deletion of the genes arcA or iclR on 2′-FL production by E. coli FL-B21 was investigated. Knockout of arcA and iclR was surprisingly useful for avoiding acetate formation. Moreover, the arcA mutant FL-011 displayed higher 2′-FL production and higher lactose and glycerol utilization efficiency than the control strain FL-B21. The 2′-FL production of the arcA mutant FL-011 was 5.04 g/L. To maximize 2′-FL production by FL-011, systematic optimization of the culture medium composition and induction conditions was performed. As a result, the production of 2′-FL reached 7.1 g/L in shake-flask culture.
Author contributions
Yingxue Liao conceived and designed the experiments. Yingxue Liao and Yuanfei Ge performed the experiments. Zhijian Ni, Jinyong Wu and Zhongkui Li contributed to the experiment plan and discussion of the manuscript. Yingxue Liao wrote the manuscript under the guidance of Xiangsong Chen and Jianming Yao. All authors reviewed and approved the manuscript.
Acknowledgements
This work was supported by the Science and Technology Service Network Initiative of Chinese Academy of Science and Wuhan Science and Technology Bureau under Grant No. KFJ-STS-QYZD-122 and Grant No. 2020020602012110, respectively. In addition, this work was supported by Hubei Industrial Technology Innovation and Incubation Center.
Disclosure statement
The authors have no financial conflicts of interest to declare.
Additional information
Funding
References
- Bode L. The functional biology of human milk oligosaccharides. Early Hum Dev. 2015;91(11):619–622.
- Bode L. Human milk oligosaccharides: every baby needs a sugar mama. Glycobiology. 2012;22(9):1147–1162.
- Kunz C, Rudloff S, Baier W, et al. Oligosaccharides in human milk: structural, functional, and metabolic aspects. Annu Rev Nutr. 2000;20:699–722.
- Castanys-Munoz E, Martin MJ, Prieto PA. 2'-fucosyllactose: an abundant, genetically determined soluble glycan present in human milk. Nutr Rev. 201371(12):773–789.
- Newburg DS, Ruiz-Palacios GM, Morrow AL. Human milk glycans protect infants against enteric pathogens. Annu Rev Nutr. 2005;25:37–58.
- Han NS, Kim TJ, Park YC, et al. Biotechnological production of human milk oligosaccharides. Biotechnol Adv. 2012;30(6):1268–1278.
- Chin YW, Park JB, Park YC, et al. Metabolic engineering of Corynebacterium glutamicum to produce GDP-L-fucose from glucose and mannose. Bioprocess Biosyst Eng. 201336(6):749–756.
- Hollands K, Baron CM, Gibson KJ, et al. Engineering two species of yeast as cell factories for 2'-fucosyllactose. Metab Eng. 2019;52:232–242.
- Sprenger GA, Baumgärtner F, Albermann C. Production of human milk oligosaccharides by enzymatic and whole-cell microbial biotransformations. J Biotechnol. 2017;258:79–91.
- Bode L, Contractor N, Barile D, et al. Overcoming the limited availability of human milk oligosaccharides: challenges and opportunities for research and application. Nutr Rev. 2016;74(10):635–644.
- Fernandezmayoralas A, Martinlomas M. Synthesis of 3-fucosyl-lactose and 2'-fucosyl-lactose and 3,2'-difucosyl-lactose from partially benzylated lactose derivatives. Carbohydr Res. 1986;154:93–101.
- Jain RK, Locke RD, Matta KL. A convenient synthesis of O-α-L-fucopyranosyl-(1→2)-O-β-D-galactopyranosyl-(1→4)-D-glucopyranose (2'-O-α-l-fucopyranosyllactose). Carbohydr Res. 1991;212:C1–C3.
- Izumi M, Tsuruta O, Harayama S, et al. Synthesis of 5-thio-L-fucose-containing disaccharides, as sequence-specific inhibitors, and 2'-fucosyllactose, as a substrate of alpha-L-fucosidases. J Org Chem. 1997;62(4):992–998.
- Pereira CL, McDonald FE. Synthesis of human milk oligosaccharides: 2'- and 3'-fucosyllactose. Heterocycles. 2012;84(1):637–655.
- Wang GN, Andre S, Gabius HJ, et al. Bi- to tetravalent glycoclusters: synthesis, structure-activity profiles as lectin inhibitors and impact of combining both valency and headgroup tailoring on selectivity. Org Biomol Chem. 2012;10(34):6893–6907.
- Rencurosi A, Poletti L, Panza L, et al. Improvement on lipase catalysed regioselective O-acylation of lactose: a convenient route to 2'-O-fucosyllactose. J Carbohydr Chem. 2001;20(7&8):761–765.
- Scheppokat AM, Morita M, Thiem J, et al. Enzymatic alpha(1→2)-L-fucosylation: investigation of the specificity of the alpha(1→2)-L-galactosyltransferase from Helix pomatia. Tetrahedron-Asymmetry. 2003;14(16):2381–2386.
- Albermann C, Piepersberg W, Wehmeier UF. Synthesis of the milk oligosaccharide 2'-fucosyllactose using recombinant bacterial enzymes. Carbohydr Res. 2001;34(2):97–103.
- Agoston K, Hederos MJ, Bajza I, et al. Kilogram scale chemical synthesis of 2'-fucosyllactose. Carbohydr Res. 2019;476:71–77.
- Lee W-H, Pathanibul P, Quarterman J, et al. Whole cell biosynthesis of a functional oligosaccharide, 2'-fucosyllactose, using engineered Escherichia coli. Microb Cell Fact. 2012;11:48.
- Chin Y-W, Seo N, Kim J-H, et al. Metabolic engineering of Escherichia coli to produce 2'-fucosyllactose via salvage pathway of guanosine 5'-diphosphate (GDP)-l-fucose. Biotechnol Bioeng. 2016;113(11):2443–2452.
- Ni Z, Li Z, Wu J, et al. Multi-path optimization for efficient production of 2'-fucosyllactose in an engineered Escherichia coli C41 (DE3) derivative. Front Bioeng Biotechnol. 2020;8(1):13.
- Stein DB, Lin YN, Lin CH. Characterization of Helicobacter pylori alpha 1,2-fucosyltransferase for enzymatic synthesis of tumor-associated antigens. Adv Synth Catal. 2008;350(14–15):2313–2321.
- Lee WH, Han NS, Park YC, et al. Modulation of guanosine 5'-diphosphate-D-mannose metabolism in recombinant Escherichia coli for production of guanosine 5'-diphosphate-L-fucose. Bioresour Technol. 2009;100(24):6143–6148.
- Huang D, Yang K, Liu J, et al. Metabolic engineering of Escherichia coli for the production of 2'-fucosyllactose and 3-fucosyllactose through modular pathway enhancement. Metab Eng. 2017;41:23–38.
- Farmer WR, Liao JC. Reduction of aerobic acetate production by Escherichia coli. Appl Environ Microbiol. 1997;63(8):3205–3210.
- Nakano K, Rischke M, Sato S, et al. Influence of acetic acid on the growth of Escherichia coli K12 during high-cell-density cultivation in a dialysis reactor. Appl Microbiol Biotechnol. 1997;48(5):597–601.
- Chin Y-W, Kim J-Y, Kim J-H, et al. Improved production of 2'-fucosyllactose in engineered Escherichia coli by expressing putative α-1,2-fucosyltransferase, WcfB from Bacteroides fragilis. J Biotechnol. 2017;257:192–198.
- Lynch AS, Lin ECC. Transcriptional control mediated by the ArcA two-component response regulator protein of Escherichia coli: characterization of DNA binding at target promoters. J Bacteriol. 1996;178(21):6238–6249.
- Sauer U, Hatzimanikatis V, Bailey JE, et al. Metabolic fluxes in riboflavin-producing Bacillus subtilis. Nat Biotechnol. 1997;15(5):448–452.
- Fischer E, Zamboni N, Sauer U. High-throughput metabolic flux analysis based on gas chromatography-mass spectrometry derived 13C constraints. Anal Biochem. 2004;325(2):308–316.
- Perrenoud A, Sauer U. Impact of global transcriptional regulation by ArcA, ArcB, Cra, Crp, Cya, Fnr, and Mlc on glucose catabolism in Escherichia coli. J Bacteriol. 2005;187(9):3171–3179.
- Vemuri GN, Eiteman MA, Altman E. Increased recombinant protein production in Escherichia coli strains with overexpressed water-forming NADH oxidase and a deleted ArcA regulatory protein. Biotechnol Bioeng. 2006;94(3):538–542.
- Nikel PI, Pettinari MJ, Ramirez MC, et al. Escherichia coli arcA mutants: metabolic profile characterization of microaerobic cultures using glycerol as a carbon source. J Mol Microbiol Biotechnol. 2008;15(1):48–54.
- Kim HJ, Hou BK, Lee SG, et al. Genome-wide analysis of redox reactions reveals metabolic engineering targets for D-lactate overproduction in Escherichia coli. Metab Eng. 2013;18:44–52.
- Yamamoto K, Ishihama A. Two different modes of transcription repression of the Escherichia coli acetate operon by IclR. Mol Microbiol. 2003;47(1):183–194.
- Gui LZ, Sunnarborg A, Pan B, et al. Autoregulation of iclR, the gene encoding the repressor of the glyoxylate bypass operon. J Bacteriol. 1996;178(1):321–324.
- Cortay JC, Negre D, Galinier A, et al. Regulation of the acetate operon in Escherichia coli: purification and functional characterization of the IclR repressor. EMBO J. 1991;10(3):675–679.
- Cozzone AJ. Regulation of acetate metabolism by protein phosphorylation in enteric bacteria. Annu Rev Microbiol. 1998;52:127–164.
- El-Mansi M, Cozzone AJ, Shiloach J, et al. Control of carbon flux through enzymes of central and intermediary metabolism during growth of Escherichia coli on acetate. Curr Opin Microbiol. 2006;9(2):173–179.
- Scheel RA, Ji L, Lundgren BR, et al. Enhancing poly(3-hydroxyalkanoate) production in Escherichia coli by the removal of the regulatory gene arcA. Amb Express. 2016;6(1):120.
- Hun J, Jung H-M, Jung M-Y, et al. Effects of gltA and arcA mutations on biomass and 1,3-propanediol production in Klebsiella pneumoniae. Biotechnol Bioproc E. 2019;24(1):95–102.
- Ding Z, Fang Y, Zhu L, et al. Deletion of arcA, iclR, and tdcC in Escherichia coli to improve l-threonine production. Biotechnol Appl Biochem. 2019;66(5):794–807.
- Nanchen A, Schicker A, Revelles O, et al. Cyclic AMP-dependent catabolite repression is the dominant control mechanism of metabolic fluxes under glucose limitation in Escherichia coli. J Bacteriol. 2008;190(7):2323–2330.
- Waegeman H, Beauprez J, Moens H, et al. Effect of iclR and arcA knockouts on biomass formation and metabolic fluxes in Escherichia coli K12 and its implications on understanding the metabolism of Escherichia coli BL21 (DE3). BMC Microbiol. 2011;11:70.
- Waegeman H, Maertens J, Beauprez J, et al. Effect of iclR and arcA deletions on physiology and metabolic fluxes in Escherichia coli BL21 (DE3). Biotechnol Lett. 2012;34(2):329–337.
- Liu M, Ding Y, Chen H, et al. Improving the production of acetyl-CoA-derived chemicals in Escherichia coli BL21(DE3) through iclR and arcA deletion. BMC Microbiol. 2017;17(1):10.
- Datsenko KA, Wanner BL. One-step inactivation of chromosomal genes in Escherichia coli K-12 using PCR products. Proc Natl Acad Sci U S A. 2000;97(12):6640–6645.
- Lee WH, Shin SY, Kim MD, et al. Modulation of guanosine nucleotides biosynthetic pathways enhanced GDP-L-fucose production in recombinant Escherichia coli. Appl Microbiol Biotechnol. 2012;93(6):2327–2334.
- Wang G, Rasko DA, Sherburne R, et al. Molecular genetic basis for the variable expression of Lewis Y antigen in Helicobacter pylori: analysis of the alpha (1,2) fucosyltransferase gene. Mol Microbiol. 1999;31(4):1265–1274.
- Nizam SA, Zhu J, Ho PY, et al. Effects of arcA and arcB genes knockout on the metabolism in Escherichia coli under aerobic condition. Biochem Eng J. 2009;44(2–3):240–250.
- Sahdev S, Khattar SK, Saini KS. Production of active eukaryotic proteins through bacterial expression systems: a review of the existing biotechnology strategies. Mol Cell Biochem. 2008;307(1–2):249–264.
- Huber R, Roth S, Rahmen N, et al. Utilizing high-throughput experimentation to enhance specific productivity of an E.coli T7 expression system by phosphate limitation. BMC Biotechnol. 2011;11(1):22.
- Shiloach J, Fass R. Growing E. coli to high cell density-a historical perspective on method development. Biotechnol Adv. 2005;23(5):345–357.
- Donovan RS, Robinson CW, Glick BR. Review: optimizing inducer and culture conditions for expression of foreign proteins under the control of the lac promoter. J Ind Microbiol. 1996;16(3):145–154.
- Seydametova E, Yu J, Shin J, et al. Search for bacterial alpha 1,2-fucosyltransferases for whole-cell biosynthesis of 2'-fucosyllactose in recombinant Escherichia coli. Microbiol Res. 2019;222:35–42.
- Yu S, Liu J-J, Yun EJ, et al. Production of a human milk oligosaccharide 2'-fucosyllactose by metabolically engineered Saccharomyces cerevisiae. Microb Cell Fact. 2018;17(1):101.
- Liu J-J, Kwak S, Pathanibul P, et al. Biosynthesis of a functional human milk oligosaccharide, 2'-fucosyllactose, and L-fucose using engineered Saccharomyces cerevisiae. ACS Synth Biol. 2018;7(11):2529–2536.
- Deng J, Gu L, Chen T, et al. Engineering the substrate transport and cofactor regeneration systems for enhancing 2'-fucosyllactose synthesis in Bacillus subtilis. ACS Synth Biol. 2019;8(10):2418–2427.