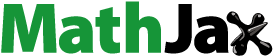
Abstract
The main challenge in bioreactor scale-up is maintaining the bioprocess productivity. The aim of the present work was to scale-up the protein production with enhanced proteolytic activity by phosphate addition in Jacaratia mexicana cell culture, by using the shear rate as scale-up criterion. The volumetric proteolytic activity in the J. mexicana cell culture in shake flask increased 1.9 times with increasing phosphate concentration from 1.25 to 2.5 mmol L−1, while the protein concentration was similar (∼55 mg L−1). This culture was scaled-up from 0.4-L to 4-L in stirred tank bioreactors at average shear rate of 200 s−1 and 234 s−1, respectively, increasing the phosphate concentration in the 4-L bioreactor (2.5 mmol L−1) with respect to the 0.4-L bioreactor (1.25 mmol L−1). There was also an increase in the volumetric proteolytic activity (2 times), without statistically significant difference in the protein concentration. Scale-up strategy and improvement of the culture medium exhibited a similar behaviour maintaining the protein productivity and increasing the proteolytic activity. These results indicate that the average shear rate is a useful hydrodynamic parameter in the scale-up of protein production of J. mexicana cell culture. Additional phosphate and bioreactor hydrodynamics could be related to unusual lengthening and thinning of most cells in the 4-L stirred tank bioreactor. Finally, the power input per unit volume in the scaled-up bioreactor decreased by 53%, improving the volumetric proteolytic activity and maintaining protein productivity. These results encourage proceeding with the scale-up process using the shear rate as a scale-up criterion.
ORCID: 0000-0001-8767-0784
Introduction
Bioprocesses development has as a recurrent step the bioreactor scale-up; i.e. to carry out the culture in a bioreactor of greater operation volume than the bioreactor that reached the target productivity [Citation1]. The main objective of bioreactor scale-up is to achieve at least the same productivity in the large bioreactor as in the small bioreactor. There are several scale-up strategies, which depend on the parameter that has the greatest influence on productivity. This parameter is called the scale-up criterion [Citation2].
Plant cells can be sensitive to the mixing of culture medium, which is done to achieve a homogeneous distribution in bioreactors. Shear rate and shear stress are parameters used for describing the bioreactor hydrodynamics. In Newtonian liquids, the shear stress is a linear function of the shear rate, where the slope is the viscosity. Shear rate can decrease or increase metabolite production, associated or not with changes in morphology and cell size, as described below.
The coumarins and sphaeralcic acid produced by Sphaeralcea angustifolia cells cultured in stirred tank bioreactor (STB) decreased (10%) when the shear rate increased from 33 s−1 to 66 s−1 [Citation3]. Ajmalicine and serpentine accumulation in Catharanthus roseus cells cultured in unbaffled 12-L STB decreased (87 and 50% respectively) when the agitation increased from 100 to 700 rpm [Citation4]. Equal Ginseng saponin production (15 g L−1) and productivity (77 mg L−1 d−1) in Panax notoginseng cells in 3-L STB were observed when the agitation increased from 80 to 210 rpm [Citation5]. The taxol production in Taxus cuspidate cells suspension culture in rotating wall vessel, decreased at shear stress values above 2.3 × 10−3 Nm−2 [Citation6].
Some examples of improve metabolite production at increasing shear rate are as follows: the phenols accumulation in Centaurea calcitrapa cells culture was 74 and 140 µg L−1, at shear rates of 5 and 42 s−1, respectively [Citation7]. Higher protein productivity was achieved in transgenic rice cell culture at 160 rpm [Citation8]. The anthraquinones concentration in the Rubia tinctorum cell culture increased 233% in a 1.5-L STB at 450 rpm, when it scaled-up from shake flask at 100 rpm [Citation9]. In a 7-L stirred tank bioreactor cell culture of Thevetia peruviana, the increase in agitation from 300 to 800 rpm favoured the metabolic activity (Reactive Oxygen Species); from 300 to 550 rpm, biomass concentration (11.92 g DW L−1) and intracellular phenolic compounds production (57.78 mg EGA/100 g FW). The scale-up to 20-L stirred tank bioreactor for cell suspension of Vitis labrusca gave similar growth rates to those observed in shake flask culture and showed high production of resveratrol (4.23 g L−1) and δ-viniferin (0.76 g L−1) [Citation10].
Buddleja cordata cells cultured in a 2-L stirred tank bioreactor enhance the biomass production (13.62 g L−1) and phenolic secondary metabolites (total phenolic (64.63 mg gallic acid equivalents g−1), phenylethanoid glycoside (119.24 mg verbascoside equivalents g−1)) and flavonoid (5.02 mg quercetin equivalents g−1) at 400 rpm [Citation11]. The highest production of biomass (13.62 g L−1) and PSMs [PeC of 64.63 mg gallic acid equivalents g−1 (mg GAE g−1); PeGC of 119.24 mg VB equivalents g−1 (mg VBE g−1); and FC of 5.02 mg quercetin equivalents g−1 (mg QE g−1)] occurred in B2RD at 400 rpm [Citation12]. In addition, the alkaloids concentration in Uncaria tomentosa culture in a 2-L STB increased 10-fold at 400 rpm compared with shake flask at 110 rpm. Furthermore, an enlargement (ƒe 1.4 to 2.55) of U. tomentosa cells was demonstrated [Citation13].
One of our previous works [Citation14] describes the protein production (proteases) of J. mexicana cells suspension culture in three different types of bioreactors. Higher proteolytic activity (170000 TU L−1) and higher protein accumulation (56 mg L−1) arisen in a 0.4-L STB at shear rate of 270 s−1. Under this hydrodynamic condition, the cells were smaller and thinner compared to cell culture in bubble column and airlift bioreactors (40 s−1). The phosphate was 1.25 mmol L−1 (the basal phosphate concentration reported in Murashige & Skoog (MS) [Citation15]) in the experiments mentioned above; however, few reports have mentioned that increasing phosphate concentration could improve the protein concentration [Citation16–18].
The phosphorus is one of the six essential macronutrients required for plants, by its participation in photosynthesis, as building blocks of genes and phospholipids required in cellular division [Citation19–21]. The role of phosphorus is evident in the maximum content of ginsenoside Rg1 achieved at 1.1 mmol L−1 phosphate, as well as the higher concentration of ginsenoside Rd at 2.21 mmol L−1 phosphate, in the Panax quinquefolium cells culture [Citation22]. The Astragalus membranaceus roots suspension culture in shake flasks and bioreactor evidenced high yield (2-fold) of Calycosin-7-O-β-D-glucoside when the phosphate concentration was 2.5 mmol L−1 [Citation23]. Also, in the adventitious root culture of Panax ginseng, a lineal correlation between the total saponin and phosphate concentration was significant [Citation24]. Azadirachtin production from Azadirachta indica cell culture and biomass increased when the phosphate increased from 1.25 to 2.0 mmol L−1 [Citation25]. In addition, callus initiation of Agropyron repens improved when the phosphate concentration increased from 1.25 to 8.5 mmol L−1 [Citation26]. Some plant species increase the metabolite synthesis at low phosphate concentration, like camptothecin production from Nothapodytes nimmoniana cells culture optimized at 0.5 mmol L−1 [Citation27] and ginseng saponin production from Panux ginseng cells at 0.25 mmol L−1 [Citation18]. However, conessine production of Holarrbena antidysenterica cells decreased at 0.25 mmol L−1 phosphate [Citation28]. In addition, high (12.5 mmol L−1) and low (0.125 mmol L−1) phosphate concentration inhibited the solasodine production from Solanum khasianum cells culture, the negative effect being greater at high concentration (12.5 mmol L−1) [Citation29].
Studies of Gamborg’s (B5) [Citation30] and Murashige & Skoog (MS) [Citation15] on vitamins indicated that B5 improved the production of asodine of S. khasianum cells culture [Citation29]. Also, favoured the regeneration from the proximal ends of the cotyledons of Vigna radiata [Citation31] and significantly increased the levels of hypericins in shoot cultures of Hypericum rumeliacum and Hypericum tetrapterum [Citation32]. The use of vitamin B5 in the J. mexicana culture could improve the protein production.
Phosphate and the vitamin type affect the metabolite synthesis and cell growth in different ways, according to the plant cell species, as mentioned above. Therefore, the objective of this work was to optimize the culture medium for protein production and scale up the improved process for the protein production of J. mexicana, using the shear rate as the scale-up criterion.
Materials and methods
J. mexicana seeds germination
J. mexicana seeds were washed with extran for 15 min, later were sterilized in 70% (v/v) ethanol for 1 min followed by immersion in a 1.2% (v/v) sodium hypochlorite solution for 15 min, after were rinsed four times in sterile water. The sterile seeds were germinated on 6 g L−1 agar noble, MS¼ [Citation15] basal salts at pH 5.7. The cultures ran at 25 ± 2 °C and 16:8 h photoperiod (50 µmol photons m−2 s−1) for 14 days, when the plantlets reach size 10-15 cm.
Callus and cell cultures of J. mexicana in shaken flask
The J. mexicana callus cultures began with stem cuttings (1 cm) placed on MS medium supplemented with 30 g L−1 sucrose, 0.5 mg L−1 6-Benzylaminopurine (BA), 0.25 mg L−1 2,4-Dichlorophenoxyacetic acid (2, 4-D) and 6 g L−1 agar noble at pH 5.7. The phosphate concentration and vitamin type are mentioned in the experimental design section. Cultures were at 25 ± 2 °C and 16:8 h photoperiod (50 µmol photons m−2 s−1). The calluses were transferred to fresh media every two weeks. Three independent experiments with ten repetitions were carried out.
The J. mexicana cell cultures in 250 mL shaken-flask containing 50 mL MS medium supplemented with 40 g L−1 sucrose, 0.5 mg L−1 BA and 0.25 mg L−1 2, 4-D at pH 5.7, began with 3.0 g of calluses, at 150 rpm, 25 ± 2 °C and 16:8 h photoperiod (50-µmole m−2 s−1). The phosphate concentration and vitamin type Gamborg’s (B5) [Citation30] or Murashige & Skoog (MS) [Citation15] were conducted as mentioned in the experimental design. Three independent experiments were carried out.
Experimental design
As a first step in the improvement of protein productivity in the shake flask cultures, a two-level fractional factorial design 22 (FFD) [Citation33] was used to analyze the medium components influencing the protein production. The FFD had two factors with two coded levels (-1 and +1) and was run to evaluate the linear effects and possible interactions between the two factors tested on the proteolytic activity, protein, and biomass. The factors were phosphate concentration, which were 0.125 to 0.250 mmol L−1 and vitamin type, MS and B5. The results were fitted with a first-order model.
Scale-up strategy
The J. mexicana cells culture grown in a 0.4-L STB was scaled-up to a 4-L STB. The scale-up strategy selected the average shear rate as the scale-up criterion, because in the 0.4-L STB (using 1.25 mol L−1 phosphate in the MS basal salt mixture), the protein concentration and the proteolytic activity correlated linearly with the average shear rate (r2> 0.96). The highest average shear rate was 274 s−1, with which the highest protein concentration (56 mg L−1) and the highest proteolytic activity (170000 TU L−1) were obtained [Citation14]. The 4-L bioreactor operated at 229 rpm achieved an average shear rate of 274 s−1. This average shear rate was in the scale-up zone, from 200 s−1 to 274 s−1, as it is observed in Figures 3A and 4A in Oliver-Salvador et al. [Citation14].
Average shear rate, microscale of turbulence and power input were calculated as indicated elsewhere [Citation14]. Maximum shear rate was calculated from impeller diameter (Di), agitation rate (N), liquid viscosity (µ) and density (ρ) by EquationEquation 1(1)
(1) (Robertson and Ulbrecht, 1987 [Citation34]).
(1)
(1)
The pumping number (NQ) was evaluated employing a stirred tank with two Rushton impellers of standard geometry, in two-phase flow operating at 225, 300 and 400 rpm (Moghaddas et al, 2008 [Citation35]). EquationEquation 2(2)
(2) evaluated the pumping capacity (Q).
(2)
(2)
and the residence time in the high shear rate zone (tr) can be expressed as EquationEquation (3)
(3)
(3)
(3)
(3)
where Vo is the operating liquid volume.
J. mexicana cell culture in stirred tank bioreactor
Experiments were performed in a 4-L STB of standard design (New Brunswick, New Jersey USA) with two Rushton turbines. The culture began with 200 g fresh weight of J. mexicana cells in MS medium supplemented with 40 g L−1 sucrose, 0.5 mg L−1 BA and 0.25 mg 2, 4-D at pH 5.7; 25 ± 2 °C, and 16:8 h photoperiod (50 µmol m−2 s−1). Sodium phosphate and MS salt concentrations, as well as the vitamin type added were determined as optimal, based on results of the FFD. The bioreactor operated at 229 rpm with an aeration rate of 0.5 vvm.
J. mexicana cell size and morphology
Cell images were obtained using a microscope Leica CTR5000. Magnification of the image was 100X. The image analysis from photographs of cell culture samples was realized using ImageJ software. The length, width and cell area were calculated using Excel (Microsoft, Inc. USA) and the statistical analysis was performed using SAS (Statistical Analysis System Version 9.0, Trial Version). The length-to-width ratio (fe) and the areas ratio (fa), defined as the area of a cell relative to the area of an ellipse with the same length and width of the cell, was determined. At least 700 cells were measured for each sampling.
Analytical methods
For biomass analysis reported as dry weight (DW), 1.0 mL aliquots were filtered through 0.22 µm (Millipore) nitrocellulose membrane to separate from broth. The cells were weighed after drying at 60 °C to constant weight for 24 h.
Protein concentration from filtrated broth was determined by the Bradford method [Citation36] (this extracellular protein concentration henceforth is referred to as protein concentration) and cell viability was evaluated by the Trypan Blue dye exclusion test, as described elsewhere [Citation14].
Proteolytic activity of filtrated broth was determined according to [Citation37] as modified by [Citation38] employing as substrate 1% casein solution in 0.05 mol L−1 sodium phosphate at pH 7.6 and 35 °C. The proteolytic activity expressed as Tyrosine Units per litre (TU L−1) means the activity that liberates the equivalent of one microgram of tyrosine per hour.
Viscosity was determined using a Haake viscometer (model RV20 Germany) at 25 ± 0.1 °C and the oxygen uptake and oxygen transfer rates were measured using the dynamic method. The response time of the dissolved oxygen probe (Cole Parmer Instruments Co., USA) was 19 s.
Molecular weight of J. mexicana protease produced in the 4-L STB was determined by sodium dodecyl sulphate polyacrylamide gel electrophoresis (SDS-PAGE) using 10% SDS-containing polyacrylamide. Protein samples were stained with Coomassie blue and visualized in a ChemiDoc system (Bio-Rad).
Immunoblot analysis of J. mexicana protease produced in the 4-L STB was performed by transferring the proteins separated by electrophoresis, to polyvinylidene difluoride (PVDF) membrane (Bio-Rad). The protease was detected with a rabbit anti-mexicain (MX-4) polyclonal antibody (produced in our laboratory, unpublished data). Immunobiochemical detection was performed using the Western ECL Blotting Substrates (Bio-Rad) following the manufacturer’s instructions.
Data analysis
Statistical analysis was conducted by means of analysis of variance (ANOVA) using the Microsoft Excel version 365. All experiments were repeated three times.
Results and discussion
The success of the bioprocesses scale-up occurs when the productivity in the large bioreactor is equal or greater than that obtained in the small bioreactor. In this work, we calculated the productivity, expressed in grams of the product per litre per day, with the concentration of extracellular protein, as well as with the cellular concentration obtained at 21 days of the culture. Once a better medium for protein production was obtained in shake flask experiments, subsequently the optimized medium was employed for the scale-up the protease production from a 0.4-L STB to a 4-L STB. We describe below the procedure to improve the culture medium.
Experimental design for improving protein productivity
Preliminary studies showed that the sodium phosphate and the vitamin type affected callus growth and its friability, as well as the cell growth and metabolite production (data not shown). These components have been reported to have an important effect on metabolite production and cell growth [Citation26, Citation32]. Therefore, a factorial design of experiments 22 was performed for determining the phosphate concentration and the vitamin type, influencing on protein production and proteolytic activity. shows the coded values and actual values of those factors and the responses measured. The ANOVA showed that the factor that significantly increased the proteolytic activity and biomass production was phosphate (p < 0.05) as shown in and for proteolytic activity, and and for biomass. The protein content was neither affected by the phosphate concentration nor by the vitamin type (data not shown). This behaviour was similar in an alfalfa cell culture, where the soluble protein concentration in media supplemented with phosphorus was not significantly different when the phosphorus concentration increased from 0.5 mmol L−1 to 5 mmol L−1 [Citation17].
Figure 1. Pareto chart of the standardized effects of phosphate and vitamin on proteolytic activity of Jacaratia mexicana cell culture (α = 0.05). Dotted line represents t = 2.306.
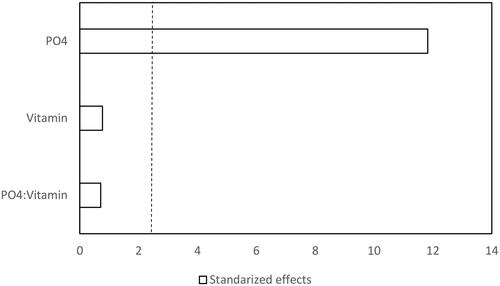
Figure 2. Pareto chart of the standardized effects of phosphate and vitamin on biomass of Jacaratia mexicana cell culture (α = 0.05). Dotted line represents t = 2.306.
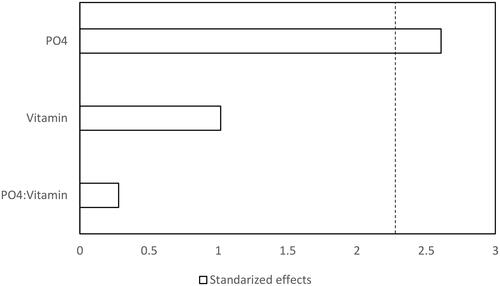
Table 1. Full factorial design 22 and responses observed on Jacaratia mexicana cell culture in shake flask.
Table 2. ANOVA: Proteolytic activity versus phosphate concentration and vitamin type of Jacaratia mexicana cell culture in shake flask.
Table 3. ANOVA: Biomass versus phosphate concentration and vitamin type of Jacaratia mexicana cell culture in shake flask.
Here the data showed similar protein concentration, and enhanced proteolytic specific activity was observed at 2.5 mmol L−1 phosphate. One possible explanation is that the presence of phosphate affects gene expression, as demonstrated before [Citation39, Citation40] thus, increasing de novo protease synthesis in a non-specific manner or, perhaps enhancing proteolytic isoforms. There is also a possibility that phosphate itself stimulates the proteolytic activity of these proteolytic enzymes. Additional experiments are required to confirm the phosphate effect. Meanwhile, as the vitamin type did not influence the proteolytic activity or the protein concentration, Gamborg’s vitamins (B5) were chosen in the subsequent scale-up experiments.
Shear rate as the scale-up criterion
The selection of the scale-up criterion is based on choosing a process parameter that most influences on productivity and finding the range of values of said parameter in which productivity remains high, with the purpose of replicating it in the process scale-up. Productivity is obtained by dividing the product concentration by process time. In this work, the average shear rate was chosen as the scale-up criterion because in J. mexicana cultures grown in various types of bioreactors, the protein concentration and proteolytic activity had a linear correlation with the average shear rate (r2 > 0.96) , not so with the power input, nor with the microturbulence, nor with the volumetric oxygen transfer coefficient, since the oxygen uptake rate of the cells was always lower than the oxygen transfer rate of the bioreactor, as mentioned at the end of this section. Furthermore, the cells had changes in shape and size when they were at an average shear rate from 15 s−1 to 270 s−1. However, at average shear rate between 200 s−1 and 270 s−1 there was a greater proteolytic activity and a greater accumulation of extracellular proteins.
The average shear rate in the scale-up of the J. mexicana cell culture has the advantage that the culture medium, even with the maximum cell concentration reaches (4.15 g DW L−1), exhibits a Newtonian behaviour, with a viscosity of 1.03 mPa s, which is expected to remain constant throughout the growth. Also, Newtonian behaviour in B. vulgaris cell cultures with a biomass of 4 g DW L−1 has been reported [Citation41]. The low viscosity of the culture medium is explained by the fact that the cells of J. mexicana grow in a unicellular manner and do not produce extracellular polymers that can increase the viscosity. In Newtonian liquids, by varying the shear stress, the viscosity remains constant, in such a way that it is possible to know the average and maximum shear rate to which the cells are subjected, using the equations described in this work.
When scaling-up bioprocesses, all hydrodynamic and oxygen transfer parameters change, even the scale-up criterion, always within the scale-up zone values. Then, an analysis of the parameters in the bioreactor being scaled-up must be carefully done to avoid adverse effects on cell growth and metabolite production [Citation42].
In bioreactors containing Newtonian liquids, there is shear stress distribution in the bioreactor determined for the viscosity and the shear rate distribution. There are maximum and minimum shear rates to which the plant cells are subjected; notwithstanding, the average shear rate is used as a parameter to relate growth rate and metabolite production with the bioreactor hydrodynamics.
The culture medium of the J. mexicana cell culture is a Newtonian liquid; its viscosity is 1.03 cP, approximately like the water viscosity. presents the hydrodynamic parameters, power input and volumetric oxygen transfer coefficient of the 0.4-L STB and 4-L STB used in the scale-up strategy, operated at 400 rpm and 229 rpm, respectively. In the 4-L STB operated at 229 rpm, the average shear rate was in the scale-up zone (200 s−1 − 274 s−1). The average shear rate describes the bulk behaviour of the flow, while the zones of highest turbulent flow are in the impellers discharge zones [Citation43], where the cells can be affected by the high shear rate and by the time they stay in these zones; which is related to the hydraulic residence time in the above-mentioned zones [Citation1, Citation2, Citation44].
Table 4. Hydrodynamic parameters, power input and volumetric oxygen transfer coefficient of the stirred tank bioreactors used in the scaling-up strategy of Jacaratia mexicana cell culture.
In this work, the maximum shear rate in the 4-L STB was 15% lower than that in the 0.4-L STB and in both bioreactors was 6.2-fold higher than the average shear rate. The residence time in the high shear rate zone of the 4-L STB was 83% higher than that in the 0.4-L STB (). Therefore, cells in the 4-L STB would be subjected for a longer time to a lower maximum shear rate than in the 0.4-L STB. In addition, the 0.4-L STB and the 4-L STB operated at 6000 Re number and 13200 Re number, respectively. In such hydrodynamic conditions the movement of liquid in all directions is random and unsteady [Citation45].
The power input is related to the energy transferred from the movement of the impellers to the liquid and therefore, to the energy dissipated per unit mass of liquid, which is implicit in the Kolmogorov microturbulence scale [Citation46], which is responsible for causing damage to fragile cells smaller than the mentioned microturbulence. The microscale of turbulence was 15% higher in the 4-L STB (54 µm vs 47 µm in the 0.4-L STB); and therefore, the microturbulence was potentially less harmful to cells than that in the small reactor, because greater microturbulence causes lesser energy dissipation in the culture medium [Citation47]. Maximum shear rate and microturbulence could have several implications in cell growth and metabolite production, as it is described in sections below.
The power input in the 4-L STB was 53% lower than in the 0.4-L STB; even so, the cell distribution in the 4-L STB was homogenous, avoiding its sedimentation. In addition, the volumetric oxygen transfer coefficient in the 4-L STB was 18% lower than that in the 0.4-L STB; however, there was no oxygen limitation for cell growth in the 4-L STB because, the oxygen uptake rate (5.4 × 10−4 g oxygen/L h) of the maximum cell concentration (4.15 g DW/L), was lower than the oxygen transfer rate (6 × 10−2 g oxygen/L h).
Productivities
Volumetric proteolytic activity and cell productivity in the 4-L STB, using culture medium with 2.5 mmol L−1 phosphate, were higher (3.2 times and 1.5 times, respectively) than those obtained in the 0.4-L STB (, respectively), using 1.25 mmol L−1 phosphate, while the protein productivity reached in the 4-L STB was like the one obtained in the 0.4-L STB (). These results were most probably due to the use of the culture medium with 2.5 mmol L−1 phosphate in the 4- L STB (as it was demonstrated in the protein production improvement experiments in shake flask with 2.5 mmol L−1 phosphate) and to the scale-up strategy employed, because the hydrodynamic conditions which the cells were subjected to in the scaled-up bioreactor, allowed them to have a similar metabolism to the one they had in the culture medium improvement experiments with 2.5 mmol L−1 phosphate, specifically in increasing the volumetric proteolytic activity while maintaining protein productivity, which also continues suggesting that a greater number of proteases are part of the extracellular proteins formed by cells in the 4-L STB.
Proteolytic activity and protein kinetics
The proteolytic activity in the 4-L STB, with 2.5 mmol L−1 phosphate, after 7 days was approximately 2 times higher than that obtained in the 0.4-L STB, with 1.25 mmol L−1 phosphate (). In addition, the kinetics of the protein concentration was similar in both bioreactors (). It is noticeable that both results, mentioned above for bioreactors, were like those found in the improved experiments of the culture medium in shake flask cultures, where the proteolytic activity obtained with 2.5 mmol L−1 phosphate (MSMB + P medium) was 1.96 times greater than that obtained using 1.25 mmol L−1 phosphate (MSMS medium), and the protein concentration was similar for both media, considering the standard deviation ().
Figure 4. Kinetics of Jacaratia mexicana cell culture in bioreactors. Proteolytic activity (A), extracellular protein concentration (B) and cell concentration (C) of J. mexicana cell culture in 0.4-L bioreactor at 400 rpm, with 1.25 mmol L-1 phosphate concentration ( □ ) and 4-L stirred tank biorreactor at 229 rpm, with 2.5 mmol L-1 phosphate concentration ( ○ ); 0.5 vvm aeration rate. Bars represent standard deviation.
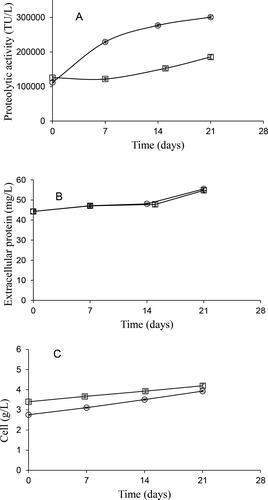
Similar effects of the increase of phosphate concentrations on proteolytic activity and protein concentration in shake flasks and bioreactors were evidence that the main objective of the scale-up strategy was achieved. Therefore, the hydrodynamic conditions of the flasks and bioreactors, although they were different, allowed to obtain the same results.
Proteinase identification
J. mexicana produce an enzymatic complex of proteases. It was characterized by cation-exchange chromatography, finding at least five isoform proteases (P-I to P-V) and P-IV (MX-4), and was denominated mexicain owing to being present in the highest quantity in fruit latex [Citation48]. To biochemically identify the mexicain produced in the 4-L STB, SDS-PAGE and Western Blot were carried out. shows a band with molecular weight of ∼24 kDa in the latex (Lane 1); the same band can be seen in the samples of cell culture of J. mexicana in the 4-L STB (Lane 3 and 4) and the molecular weight marker proteins (Lane 2). For molecular identification of mexicain shows the immunodetection of mexicain. The enzyme of J. mexicana cell culture has equal molecular weight (∼24 kDa) and cross reacted with anti-mexicain antibodies, thus are immunochemically homologous with mexicain (MX-4). Our findings demonstrate that protease produced in the 4-L STB, was equal with the mexican obtained from fruit latex of J. mexicana.
Figure 5. Electrophoresis and immunodetection of extracellular protease from Jacaratia mexicana cell suspension culture: (A) Electrophoresis SDS-PAGE of proteins from latex and broth 4 L stirred tank biorreactor at 229 rpm; (B) Immunodetection of mexicain (P-IV or MX-4). Line 1: Latex of J. mexicana fruits; Line 2: molecular weight (24 kDa); Line 3 and 4 samples of suspension culture of J. mexicana 25 µg/line of total soluble protein.

J. mexicana cell size and morphology
In a previous work, the J. mexicana cells changed in size (average length 4.5% smaller and average width 13.1% smaller) and morphology (8.6% thinner) when were submitted to average shear rate from 20 s−1 − 40 s−1 to 200 s−1 − 270 s−1 [Citation14]. In this work, although the average shear rate was 234 s−1 in the 4-L STB, the cells were 2 times longer and 0.7 times thinner on average than the cells cultivated in the 0.4-L STB (). Also, the cell morphology significantly changed in the 4-L bioreactor with respect to the 0.4-L bioreactor, since the average fe factor changed from 1.6 to 5.3, indicating that the cells were 3.3 times larger (); while the average fa factor changed from 1.01 to 0.52 (), indicating that the cells were 49% thinner.
Figure 6. Length (A), width (B), fe ratio (C) and fa (D) distributions of cells from J. mexicana cell suspension cultures in 0.4-L STB at 400 rpm (Δ); and 4-L STB at 229 rpm (○); 0.5 vvm aeration rate.
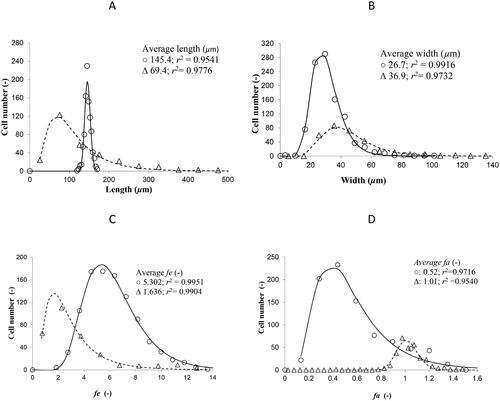
As the microturbulence in the 4-L STB was 54 µm () and the length of all cells was greater than 54 μm () and the width of all cells was smaller than 54 µm (), the cells could undergo hydrodynamic stress at the large (longitudinal direction) and not at the width, as it is reported by Nienow et al. [Citation49], where the hydrodynamics could elicit the proteases synthesis and/or its excretion toward the culture medium [Citation14]. However, the results indicate that the protein production of the cells was not negatively affected by the hydrodynamic condition in the 4-L STB and was influenced mainly for 2.5 mmol L−1 phosphate in the culture medium. The lengthening and thinning of most cells could be due to the combined effect of the 2.5 mmol L−1 phosphate and the hydrodynamic conditions in the 4-L STB, as it is described below.
Cell growth kinetics
In the 4-L STB the initial cell concentration was lower than the initial cell concentration in the 0.4-L STB; however, the cell concentration at 21 days was practically similar in both bioreactors, approximately 4.15 g DW/L; consequently, the cell productivity obtained in the 4-L STB was 1.5 times greater than that obtained in the 0.4-L STB (). These results were due to the maximum specific growth rate in the 4-L STB (0.0169 d−1), which was 1.7 times higher than that in the 0.4-L STB (0.0099 d−1), and the fact that the maximum specific growth rate was maintained along the 21 days of the culture. Therefore, the effect of 2.5 mmol L−1 phosphate was again demonstrated by causing a faster specific growth rate in the 4-L STB. The lengthening and thinning of most cells in the 4-L STB could be due to the higher maximum specific growth rate, compared with that obtained in the 0.4-L STB, because in the exponential growth phase it appears that the cells are more sensitive to the shear, because the cell wall strength may decrease during the increase in cell size [Citation50]. Therefore, the hydrodynamic conditions in the 4-L bioreactor and the culture medium with 2.5 mmol L−1 phosphate seem to be associated with more changes in cell size and morphology than those expected.
Conclusions
The volumetric proteolytic activity in the J. mexicana shake flask cell culture increased 1.9 times with increasing phosphate concentration from 1.25 to 2.5 mmol L−1, while the protein concentration was similar. The J. mexicana cell culture was scaled-up from 0.4-L to 4-L in stirred tank bioreactors using the shear rate as scale-up criterion, with an average shear rate of 234 s−1. In the 4-L bioreactor the phosphate concentration was 2.5 mmol L−1, while in the 0.4-L STB was 1.25 mmol L−1. In the scaled-up bioreactor, the volumetric proteolytic activity increased 2 times, while the protein concentration in both bioreactors was similar, approximately 55 mg/L. The results obtained in shake flask by phosphate addition were similar to those obtained in the scale-up strategy with phosphate addition, indicating that the average shear rate is a useful hydrodynamic parameter in the scale-up of J. mexicana cell culture. The lengthening and thinning of most cells in the 4-L stirred tank bioreactor were not expected. However, they may be related with a higher maximum specific growth rate due to 2.5 mmol L−1 phosphate concentration and to the bioreactor hydrodynamics. The power input per unit volume decreased 53% in the scaled-up bioreactor, maintaining the volumetric proteolytic activity and the protein productivity.
Author contributions
García-Salas: Conceptualization, Data analysis and interpretation, Writing – review & editing. Gómez-Montes: Experiment conducting, data collect, statistical analysis, drafting the manuscript. Ramírez-Sotelo: Data analysis, Drafting the manuscript and review the manuscript. Oliver-Salvador: Concept and design, Data analysis and interpretation, drafting the manuscript & review
Acknowledgements
The authors wish to acknowledge the financial support by Instituto Politécnico Nacional (IPN), México SIP 20195364 and 20200740. C. Oliver-Salvador and S. García-Salas are recipients of a fellowship from Comisión de Operación y Fomento de Actividades Académicas-IPN. Gómez-Montes E. O. was a recipient of a posdoctoral fellowship from the Estancias Posdoctorales Nacionales del Consejo Nacional de Ciencia y Tecnología CONACYT, México.
Disclosure statement
The authors report no conflict of interest
Data availability statement
All data that support the findings reported in this study are available from the corresponding author upon reasonable request.
References
- Mahdinia E, Cekmecelioglu D, Demirci A. Bioreactor scale-up. In: Berenjian A, editor. Essentials in fermentation technology. Cham: Springer International Publishing; 2019. pp. 213–236.
- Su R, Sujarani M, Shalini P, et al. A review on bioreactor technology assisted plant suspension culture. AJB2T. 2019;5(13):1–13.
- Pérez-Hernández J, Nicasio-Torres MdP, Sarmiento-López LG, et al. Production of anti-inflammatory compounds in Sphaeralcea angustifolia cell suspension cultivated in stirred tank bioreactor. Eng Life Sci. 2019;19(3):196–205.
- Leckie F, Scragg AH, Cliffe KC. Effect of bioreactor design and agitator speed on the growth and alkaloid accumulation by cultures of Catharanthus roseus. Enzyme Microb Technol. 1991;13(4):296–305.
- Zhang Z-Y, Zhong J-J. Scale-up of centrifugal impeller bioreactor for hyperproduction of ginseng saponin and polysaccharide by high-density cultivation of Panax notoginseng cells. Biotechnol Prog. 2004;20(4):1076–1081.
- Sun X, Linden JC. Shear stress effects on plant cell suspension cultures in a rotating wall vessel bioreactor. J Ind Microbiol Biotechnol. 1999;22(1):44–47.
- Raposo S, Lima-Costa ME. Rheology and shear stress of Centaurea calcitrapa cell suspension cultures grown in bioreactor. Biotechnol Lett. 2006;28(6):431–438.
- Kwon J-Y, Cheon S-H, Nam H-J, et al. Process characterization of hCTLA4Ig production in transgenic rice cell cultures using a 3-L bioreactor. Appl Biochem Biotechnol. 2013;171(5):1276–1288.
- Busto VD, Rodríguez-Talou J, Giulietti AM, et al. Effect of shear stress on anthraquinones production by Rubia tinctorum suspension cultures. Biotechnol Prog. 2008;24(1):175–181.
- Lambert C, Lemaire J, Auger H, et al. Optimize, modulate, and scale-up resveratrol and resveratrol dimers bioproduction in Vitis labrusca L. cell suspension from flasks to 20 L bioreactor. Plants. 2019;8(12):567.
- Vazquez-Marquez AM, Zepeda-Gómez C, Burrola-Aguilar C, et al. Effect of stirring speed on the production of phenolic secondary metabolites and growth of Buddleja cordata cells cultured in mechanically agitated bioreactor. Plant Cell Tiss Organ Cult. 2019;139(1):155–166.
- Arias JP, Mendoza D, Arias M. Agitation effect on growth and metabolic behavior of plant cell suspension cultures of Thevetia peruviana at bench scale reactor. Plant Cell Tiss Organ Cult. 2021;145(2):307–319.
- Trejo-Tapia G, Cerda-García-Rojas CM, Rodríguez-Monroy M, et al. Monoterpenoid oxindole alkaloid production by Uncaria tomentosa (Willd) D.C. cell suspension cultures in a stirred tank bioreactor. Biotechnol Prog. 2005;21(3):786–792.
- del Carmen Oliver-Salvador M, Morales-López E, Durán-Páramo E, et al. Shear rate and microturbulence effects on the synthesis of proteases by Jacaratia mexicana cells cultured in a bubble column, airlift, and stirred tank bioreactors. Biotechnol Bioproc E. 2013;18(4):808–818.
- Murashige T, Skoog F. A revised medium for rapid growth and bio assays with tobacco tissue cultures. Physiol Plant. 1962;15(3):473–497.
- Medora RS, Mell GP, Bilderback DE. Effect of various media on growth and protease production in Carica papaya L. callus cultures. Z Pflanzenphysiol. 1984;114(2):179–185.
- Qamar SFA, Sors TG, Cunningham SM, et al. Phosphate nutrition effects on growth, phosphate transporter transcript levels and physiology of alfalfa cells. Plant Cell Tiss Organ Cult. 2005;82(2):131–140.
- Liu S, Zhong JJ. Phosphate effect on production of ginseng saponin and polysaccharide by cell suspension cultures of Panax ginseng and Panax quinquefolium. Process Biochem. 1998;33(1):69–74.
- Raghothama KG. Phosphate transport and signaling. Curr Opin Plant Biol. 2000;3(3):182–187.
- Crawford NM, Forde BG. Molecular and developmental biology of inorganic nitrogen nutrition. In The Arabidopsis book. Vol. 1. Washington (DC): The American Society of Plant Biologists; 2002. p. e0011.
- Günter EA, Ovodov YS. Effect of calcium, phosphate and nitrogen on cell growth and biosynthesis of cell wall polysaccharides by Silene vulgaris cell culture. J Biotechnol. 2005;117(4):385–393.
- Kochan E, Szymczyk P, Kuźma Ł, et al. Nitrogen and phosphorus as the factors affecting ginsenoside production in hairy root cultures of Panax quinquefolium cultivated in shake flasks and nutrient sprinkle bioreactor. Acta Physiol Plant. 2016;38(6):149.
- Jin H, Yu Y, Hu S, et al. Effect of nitrogen concentration, source, and phosphate concentration on accumulation of biomass and calycosin-7-O-β-D-glucoside in Astragalus membranaceus adventitious roots. In Vitro Cell Dev Biol Plant. 2020;56(4):407–414.
- Kim JH, Chang EJ, Oh H-I. Saponin production in submerged adventitious root culture of Panax ginseng as affected by culture conditions and elicitors. Asia Pacific J Mol Biol Biotechnol. 2005;13:87–91.
- Prakash G, Srivastava AK. Statistical media optimization for cell growth and azadirachtin production in Azadirachta indica (A. Juss) suspension cultures. Process Biochem. 2005;40(12):3795–3800.
- Gyulai G, Janovszky J, Kiss E, et al. Callus initiation and plant regeneration from inflorescence primordia of the intergeneric hybrid Agropyron repens (L.) Beauv.xBromus inermis Leyss. cv. nanus on a modified nutritive medium. Plant Cell Rep. 1992;11(5–6):266–269.
- Karwasara VS, Dixit VK. Culture medium optimization for camptothecin production in cell suspension cultures of Nothapodytes nimmoniana (J. Grah.) Mabberley. Plant Biotechnol Rep. 2013;7(3):357–369.
- Panda AK, Mishra S, Bisaria VS. Alkaloid production by plant cell suspension cultures of Holarrhena antidysenterica: I. Effect of major nutrients. Biotechnol Bioeng. 1992;39(10):1043–1051.
- Jacob A, Malpathak N. Manipulation of MS and B5 components for enhancement of growth and solasodine production in hairy root cultures of Solanum khasianum Clarke. Plant Cell Tiss Organ Cult. 2005;80(3):247–257.
- Gamborg OL, Miller RA, Ojima K. Nutrient requirements of suspension cultures of soybean root cells. Exp Cell Res. 1968;50(1):151–158.
- Gulati A, Jaiwal PK. Culture conditions effecting plant regeneration from cotyledons of Vigna radiata (L.) Wilczek. Plant Cell Tiss Organ Cult. 1990;23(1):1–7.
- Danova K, Nikolova-Damianova B, Denev R, et al. Influence of vitamins on polyphenolic content, morphological development, and stress response in shoot cultures of Hypericum spp. Plant Cell Tiss Organ Cult. 2012;110(3):383–393.
- Kuehl RO, Kuehl R. 2000. Design of experiments: statistical principles of research design and analysis. 2nd ed. Pacific Grove (CA): Duxbury/Thomson Learning.
- Robertson B, Ulbrecht J. 1987. Measurement of shear rate on an agitator in a fermentation broth. New York (NY): American Institute of Chemical Engineers; 1987.
- Moghaddas J, Revstedt J, Trägårdh C, et al. Comparison of mixing between single-and bubbly two-phase systems in a Rushton stirred tank. Adv Fluid Mech. 2008;7(267):267–274.
- Bradford MM. A rapid and sensitive method for the quantitation of microgram quantities of protein utilizing the principle of protein-dye binding. Anal Biochem. 1976;72(1–2):248–254.
- Kunitz M. Crystallization of a trypsin inhibitor from soybean. Science. 1945;101(2635):668–669.
- Ortega ML, del Castillo LM. Actividad de la mexicaína en presencia de altas concentraciones de urea. Ciencia Mexicana. 1966;24:247–251.
- Bozzo GG, Singh VK, Plaxton WC. Phosphate or phosphite addition promotes the proteolytic turnover of phosphate-starvation inducible tomato purple acid phosphatase isozymes. FEBS Lett. 2004;573(1–3):51–54.
- Cho H, Bouain N, Zheng L, et al. Plant resilience to phosphate limitation: current knowledge and future challenges. Crit Rev Biotechnol. 2021;41(1):63–71.
- Rodrı́guez-Monroy M, Galindo E. Broth rheology, growth and metabolite production of Beta vulgaris suspension culture: a comparative study between cultures grown in shake flasks and in a stirred tank. Enzyme Microb Technol. 1999;24(10):687–693.
- Nienow AW. Scale-up considerations based on studies at the bench scale in stirred bioreactors. J Chem Eng Japan/JCEJ. 2009;42(11):789–796.
- de Lamotte A, Delafosse A, Calvo S, et al. Investigating the effects of hydrodynamics and mixing on mass transfer through the free-surface in stirred tank bioreactors. Chem Eng Sci Nancy. 2017;172:125–142.
- Jameel F, Czyzewski AM, Zhu T, et al. 2020. Chapter 22: development and scale-up of the mixing process for biopharmaceuticals. In: Feroz Jameel, John W. Skoug, Robert R. Nesbitt, editors. Development of biopharmaceutical drug-device products. PublishingCham, Switzerland: Springer Nature Switzerland AG. p. 539–565.
- Bakker A, Myers K, Ward R, et al. The laminar and turbulent flow pattern of a pitched blade turbine. Trans IChemE. 1996;74:485–491.
- Buffo MM, Corrêa LJ, Esperança MN, et al. Influence of dual-impeller type and configuration on oxygen transfer, power consumption, and shear rate in a stirred tank bioreactor. Biochem Eng J. 2016;114:130–139.
- Thomas C. 1990. Problems of shear in biotechnology. In: M. A. Winkler, editors Chemical engineering problems in biotechnology. London & New York: Chemical Industry by Elsevier Applied Sciences. p. 23–94. ISSN: 1-85166-454-8
- Oliver-Salvador MC, Gonzalez-Ramirez LA, Gavira JA, et al. Purification, crystallization and preliminary X-ray analysis of mexicain. Acta Crystallogr D Biol Crystallogr. 2004;60(Pt 11):2058–2060.
- Nienow AW, Scott WH, Hewitt CJ, et al. Scale-down studies for assessing the impact of different stress parameters on growth and product quality during animal cell culture. Chem Eng Res Des. 2013;91(11):2265–2274.
- Hooker BS, Lee JM, An G. Response of plant tissue culture to a high shear environment. Enzyme Microb Technol. 1989;11(8):484–490.