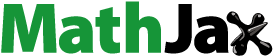
Abstract
Biological treatment is a cost-effective and environmentally friendly technique for treating toluene. However, this process is limited by the type and quantity of toluene degraders that can be applied to different environments and methods. It is a feasible solution to constructing new genetic engineering organisms for toluene degradation through biotechnology. To facilitate genetic manipulation and improve gene expression, the toluene/o-xylene monooxygenase locus from Pseudomonas stutzeri was reconstructed and artificially synthesized. All six genes were optimized and driven with a monocistronic transcriptional pattern by T7 promoter and terminator to be expressed at a high level. The engineered bacteria can hydroxylate toluene to a mixture of o-, m- and p-cresol, which are then further oxidized to 3- and 4-methylcatechol. Benzene can also be oxidized to phenol, catechol and pyrogallol successively. The negative effects of toluene can be removed by the engineered bacteria via proteomics analysis. Overall, the artificial gene clusters established in this study can be used to construct different toluene and benzene degradation organisms for bioremediation.
Supplemental data for this article is available online at https://doi.org/10.1080/13102818.2021.1996267 .
Introduction
As an important chemical raw material and process solvent, toluene is widely used for polycol, antioxidant, corrosion inhibitor, polyurethane, adhesive and polyethylene terephthalate solid-state resin production [Citation1]. The extensive use of toluene in industrial and agricultural activities results its release into the environment. When toluene enters the soil, it will adhere to soil particles through the process of adsorption. Toluene can also accumulate in the surface and ground water [Citation2]. As an indoor volatile organic pollutant, toluene can be emitted from a wide array of building materials and products used indoors. Human health, particularly in susceptible individuals, is threatened by toluene [Citation3, Citation4]. Toluene has multiple negative effects on living cells. It tends to accumulate into the lipophilic layer of the cell membrane, thereby disturbing its integrity. With the concentrations of ≥1 mg L−1, toluene can damage the nervous system, kidney and liver [Citation5]. The effects of toluene exposure on development, immune, respiratory and other physiological functions have been reported and assessed [Citation6]. Thus, the production and release of toluene are strongly regulated by national, supranational and international policies [Citation7].
Biological treatment is a cost-effective and environmentally friendly technique used to treat toluene because it can be easily degraded under anaerobic or aerobic conditions in the presence of microorganisms [Citation1]. Various aerobic or anaerobic degraders, including Burkholderia vietnamiensis, Pseudononas aeruginosa JI104, Pseudononas stutzeri OX1, Pseudomonas putida F1, Rhodococcus jostii RHA1, Thauera sp. DNT-1, Ralstonia pickettii PKO1, Nitrosomonas europaea, Pseudononas putida mt-2, Pseudononas mendocina, Pseudoxanthomonas spadix BD-a59 and different microbial consortiums, have been isolated from contaminated soils, aquifer and industrial effluent [Citation8–12]. Two fungal strains, namely, Paecilomyces variotii and Exophiala oligosperma, were isolated on toluene as the sole carbon and energy source [Citation13]. Four novel soil bacterial species that can utilize toluene as sole carbon and energy source were isolated from soil sites surrounding car painting workshops [Citation2]. However, the degraders that have been found are still struggling to meet the demands in practice, such as high removal efficiency, adaptability to different environmental conditions and remediation methods, and ecological safety of these degraders. And bioremediation of multiple contaminants remains a challenge due to negative effects of other pollutants, conflict of optimal degradation conditions of different pollutants, and complex interactions among degradaters [Citation14–16]. It is an effective solution to endow the appropriate hosts with the degradation ability of toluene through biotechnology.
In general, the degradation pathways of aromatic compounds usually include a peripheral metabolic pathway, which produces dihydroxylated aromatic intermediates, and a core metabolic pathway, which metabolizes these intermediates to enter the tricarboxylic acid cycle. Under aerobic conditions, toluene can be oxidized by different monooxygenases (TMOs) or dioxygenases resulting in the formation of cresol, benzyl alcohol and cis-toluene dihydrodiol as the initial step and funnel into methylcatechol, catechol and protocatechuate via its peripheral pathway [Citation11]. Toluene monooxygenase, including toluene 3-monooxygenase, toluene 4-monooxygenase and toluene/o-xylene monooxygenase (ToMO), are multicomponent nonheme, di-iron enzymes that can hydroxylate aromatic molecules by using molecular oxygen and NADH as a cofactor [Citation17]. ToMO is an enzymatic complex that is encoded by touABCDEF and responsible for the early stages of toluene degradation in P. stutzeri OX1 [Citation18]. ToMO displays a broad spectrum of substrate specificity, including benzene, toluene, cresols, o-xylene, m-xylene, p-xylene, 2,3-dimethylphenol, 3,4-dimethylphenol, naphthalene, ethylbenzene, styrene and chlorinated ethenes [Citation19–22]. The degradation pathways of dihydroxylated intermediates, as the core metabolic pathway of aromatic compounds, widely exist in various bacteria [Citation23, Citation24]. By expressing exogenous ToMO, toluene mineralization can be realized in these microorganisms. This phenomenon makes the enzyme potentially useful in bioremediation strategies.
Using synthetic biology to transform the degrading strain will be used to degrade typical environmental organic pollutants. Newly discovered genes, which were found in new genomes, uncultivated bacteria, and metagenomic libraries, can be used to construct engineered bacteria [Citation25–27]. Genetic optimization and modular gene synthesis is a necessary step for the heterologous expression after obtaining genes of interest. By a synthetic biological method, we have constructed several engineered bacteria to degrade organic pollutants completely, which contain different core metabolic pathways [Citation28, Citation29]. And various functional modules responsible for different peripheral degradation pathways are needed to degrade particular or multiple organic pollutants. Thus, to facilitate genetic manipulation and bioremediation further, we optimized and synthesized the original ToMO gene cluster in this study. All the six genes were driven with a monocistronic transcription pattern by T7 promoter and terminator to be expressed at a high level. The optimized artificial gene cluster can be successfully expressed in model organism Escherichia coli. The hydroxylation of toluene and benzene by the engineered bacteria was analyzed in this study. And the physiological responses of engineering bacteria exposure to toluene were also identified by proteomics analysis.
Materials and methods
Reagents, vectors, and microorganisms
All chemicals were purchased from Aladdin Industrial Co., Ltd. (Shanghai, China) or Sangon Biotech Co., Ltd. (Shanghai, China). The reagents used as the standards for high-performance liquid chromatography (HPLC) or gas chromatography mass spectrometry (GC-MS) analysis were guaranteed grade and others were analytical grade. Total RNA extractor (TRIzol) was also purchased from Sangon Biotech Co., Ltd. (Shanghai, China). Restriction enzymes, KOD DNA polymerase, and TransScript One-Step gDNA Removal and cDNA Synthesis SuperMix were purchased from TaKaRa Biomedical Technology Co., Ltd. (Beijing, China), TOYOBO Co., Ltd. (Osaka, Japan) and TransGen Biotech Co. Ltd. (Beijing, China), respectively.
The plasmids pGEM-T (Promega, China) and pET-28a-c(+) (Novagen, USA) were used as cloning and expression vectors respectively. The E. coli strain DH 5α (Invitrogen, USA) and E. coli strain BL221-AI (Invitrogen, USA) were applied to clone and express the artificial gene cluster in this study.
Genes optimization, synthesis, and bacterial transformation
All the six genes encoding the different components of ToMO are obtained from P. stutzeri (GenBank no. AJ005663.3; touA, touB, touC, touD, touE and touF). They were chemically synthesized via the PCR-based two-step DNA synthesis method and renamed touAI, touBI, touCI, touDI, touEI and touFI [Citation30]. Codons were modified according to the preferential codon usage in a balance between E. coli and plants. The cleavage sites of the common restriction enzymes in these genes were deleted to facilitate the subsequent vector construction. To improve the stability of mRNA, we removed the inverted repeats, stem-loop structures and transcription termination signal, and the GC content was optimized. The final genes were verified by PCR and DNA sequencing. All sequences of the modified genes can be found in the supplementary material. Then, the six chemically synthesized genes were driven by the T7 promoter and terminator. The six gene expression cassettes were constructed using the modified overlap extension technique [Citation31]. Routine molecular biology techniques about vector construction and bacterial transformation were performed as described previously [Citation28]. The transformant was named BL-tou.
Transcription and expression of the optimized genes
The transformant BL-tou was grown on M9 medium supplemented with 10 g L−1 glycerin (to replace glucose), 1 g L−1 casamino acids and 10 mmol L−1 thiamine hydrochloride at 37 °C for 24 h. Then, the cells were resuspended to the optical density at 600 nm (1 OD600, approximately 3 108 CFU mL−1) of 1.0 and inducted with 1 mmol L−1 IPTG and 2 g L−1 arabinose for heterologous gene expression. DNA-free RNA was isolated from cultures of BL-tou after 3 h of induction. Total RNA was extracted from cells by using Total RNA Extractor (TRIzol) according to the manufacturer’s recommendations. Then, total RNA was reverse transcribed to cDNA for PCR. The PCR programe included 30 cycles of amplification consisting of a denaturing step at 94 °C for 30 s, an annealing step at 55 °C for 30 s and an elongation step at 72 °C for 30 s; with a final extension step for 10 min at 72 °C. The 16S rDNA gene was used as an internal control. All the primers used in this study are listed in Supplemental Table S1. The relative abundances of each expressed component of optimized ToMO were analyzed by proteomic method.
Successive hydroxylation of toluene by BL-tou and proteomic analyses
The transformant BL-tou was resuspended to the optical density at 600 nm (OD600) of 1.0 with the same M9 inducing medium after 24 h of culture. A total of 1 mL of the resuspended cells was sealed with toluene (from 2 to 10 mmol L−1) in 10 mL glass vials sealed tightly with a rubber cap to minimize the loss of toluene by evaporation. The vials were shaken at 30 °C and 120 rpm. The samples were collected at different time intervals and analyzed for residual toluene and the concentrations of other metabolites.
To detect toluene, o-cresol, m-cresol, p-cresol, 3-methylcatechol and 4-methylcatechol, we infected ethyl acetate (0.5 mmol L−1 hexadecane was added) with the same volume into the sealed vial to extract for 1 h at 4 °C. After centrifugation, the upper organic phase was sucked out for gas chromatography mass spectrometry (GC-MS) analysis.
GC-MS (GC-MS/MS 7890B-7000C, Agilent Inc., USA) and HP-5 MS capillary column (30 m × 0.25 mm × 0.25 μm, Agilent Inc., USA) were used for GC-MS analysis. The chromatographic conditions were as follows: the flow rate was 1 mL min−1 (He). The temperature of the injection port was 280 °C. The GC oven programme started with an initial temperature of 50 °C held for 6 min, followed by a ramp of 20 °C min−1 to 280 °C and then held for 5 min. The sample injection amount was 1 μL, and the shunt ratio was 20:1. The temperature of the transfer line and ion source was set at 295 °C and 230 °C, respectively. The mass spectra from m/z 50 to 200 were recorded under electron ionization at 70 eV.
Proteomic analyses
For proteomic analyses, the cells, including BL-tou and the transformant with blank vector, were used after 3 h induction. Detailed experimental procedures, database search, and bioinformatics methods are provided in the Supplementary Material and based on the previous study we reported [Citation28].
The cells were dissolved in lysate buffer (8 mol L−1 urea, 1% Protease Inhibitor Cocktail, 3 μmol L−1 TSA and 50 mmol L−1 NAM) on ice using a high-intensity ultrasonic processor for 10 min. The supernatants were collected after centrifuging at 12,000g for 10 min at 4 °C. The protein concentration was determined with a BCA kit. The protein preparation was treated with 5 mmol L−1 DTT at 56 °C for 30 min followed by 11 mmol L−1 iodoacetamide (IAA) for 15 min in darkness. A total of 100 mmol L−1 tetraethylammonium bromide (TEAB) was then added to dilute the urea, and trypsin at concentrations 1:50 and 1:100 was then used to digest the protein. After digestion, Strata X C18 SPE column (Phenomenex) and vacuum-dry were used to desalt the peptides and then the peptides were reconstituted with 0.5 mol L−1 TEAB. The peptides were subsequently processed by TMT kit following the manufacturer’s recommendations. The peptides were incubated with labeling reagent at room temperature for 2 h, followed by desalting and vacuum drying.
Liquid phase A (0.1% formic acid) was used to dissolved the tryptic peptides, which were then loaded onto a home-made reversed-phase analytical column (length: 15 cm, i.d.: 75 μm) and separated by EASY-nLC 1000 ultraperformance liquid chromatography system. Liquid phase B contained 0.1% formic acid in 90% acetonitrile. The liquid phase gradient setting was as follows: 0 ∼ 23 min, 9–26% B; 23 ∼ 32 min, 26–38% B; 32 ∼ 36 min, 38–80% B; 36 ∼ 40 min, 80% B. The flow rate was maintained at 500 nL min−1. Peptides were subjected to NSI ion source for ionization followed by tandem mass spectrometry (MS/MS) in Q ExactiveTM (Thermo). The electrospray voltage was set to 2.0 kV and Orbitrap was used for detection and analysis. For primary MS, the scan range was 350–1800 m/z at a resolution of 70,000. Subsequently, normalized collision energy was set at 28% for selected peptides undergoing secondary MS/MS, with scan range starting at 100 m/z and resolution set at 17,500. A data-dependent procedure that alternated between one MS scan followed by 20 MS/MS scans was applied with 15.0 s dynamic exclusion. Automatic gain control (AGC) was set at 5E4. MS2 data were retrieved by Maxquant (v1.5.2.8).
UniProt-GOA database (http://www.ebi.ac.uk/GOA/) was used to implement the Gene Ontology (GO) annotation of proteome. Then proteins were classified by Gene Ontology annotation based on three categories: biological process, cellular component and molecular function. Protein pathways were annotated using the KEGG database. Kyoto Encyclopedia of Genes and Genomes (KEGG) database was used to annotate protein pathway. Firstly, using KEGG online service tools KAAS to annotated protein’s KEGG database description. Then mapping the annotation result on the KEGG pathway database using KEGG online service tools KEGG mapper.
Successive hydroxylation of benzene by BL-tou
Under the same conditions, 3 mL of the resuspended BL-tou was transferred into 50 mL flask to measure its oxidation ability to benzene (1 mmol L−1). Benzene was extracted and detected in the same way as toluene. Phenol, catechol and 1,2,3-benzenetriol were analyzed by HPLC using an Athena C18 HPLC column (ANPEL Inc., Shanghai, China) at 25 °C. The 0.1% formic acid solution/methanol mobile phase (70:30, vol/vol) flowing at a rate of 1.0 mL min−1 was used. Peaks were registered at 213 nm by ultraviolet spectrophotometric detector (Agilent 1100 VWD).
Results
Synthesis of six genes encoding ToMO and expression vector construction
The six optimized genes, namely, touAI, touBI, touCI, touDI, touEI and touFI encoding the different components of ToMO from P. stutzeri OX1 were obtained by chemical synthesis (). All codons were designed to be conducive to improve the expression. Each gene was added with T7 promoter and terminator and assembled to construct an artificial gene cluster. The identities of the DNA sequence of the six optimized genes were 81.2%, 76.6%, 81.2%, 78.8%, 81.5% and 79.9% similarity with original sequences. These genes were cloned into vector Pet-28a-c(+) and transformed to the host E. coli strain BL221-AI. The transformant was named BL-tou. PCR reaction was carried out to demonstrate the successful transformation of the multigene vector (). The plasmid extracted from the transformant BL-tou was used as the template.
Figure 1. Reconstruction of the ToMO gene cluster. The black arrows and striped arrows indicate the touABCDEF genes and other open reading fames in the wild ToMO gene cluster (AJ005663.3). The gray arrows indicate the optimized genes used for genetic recombination. T7p, T7 promoter; T7t, T7 terminator.
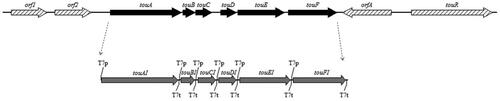
Figure 2. Cloning and expression of all synthetic genes in the transformant BL-tou. (A) PCR reaction was carried out using the plasmid extracted from BL-tou as template. (B) PCR reaction was carried out using the cDNA from BL-tou as template. (C) Expression rates of different genes at expression level in BL-tou by proteomics analysis.
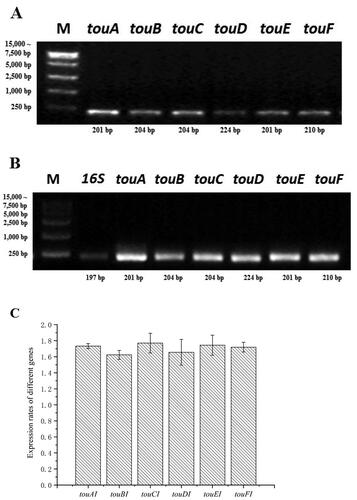
Expression of six genes in transformant BL-tou
The expression of all six genes at the transcriptional level was analyzed by reverse transcription PCR. The electrophoretic results showed that all the six genes can be transcribed normally (). All the foreign proteins were identified by proteomics analysis, and their relative expression levels were similar ().
Toluene hydroxylation by transformant BL-tou and intermediate monitoring
In the closed condition, toluene with different concentration was added to the bacterial culture broth in the M9 medium mentioned above to detect the reaction ability of the transformant BL-tou.
As shown in , the toluene consumption rate of BL-tou was significantly higher than that of control bacteria. The initial hydroxylation products, namely, o-, m- and p-cresol, were detected by GC-MS (). As time passed by, the hydroxylation continued to produce 3- and 4-methylcatechol ().
Figure 3. Degradation of toluene by the transformant BL-tou. BL-con was the transformant with empty vector. Blank indicates toluene was added to the medium without any transformant.
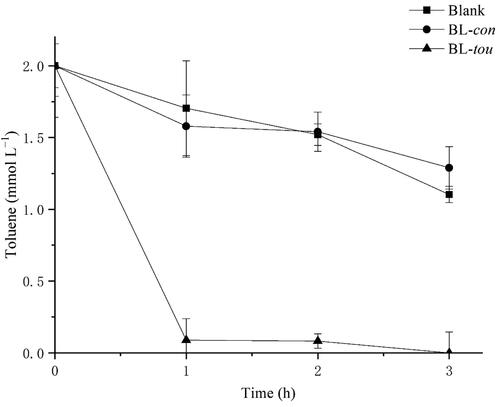
Figure 4. The products of successive hydroxylation of toluene by the transformant BL-tou. (A) Determination of the generated o-cresol, m-cresol, p-cresol, 3-methylcatechol and 4-methylcatechol by GC-MS. (B) Quantitative analysis of the toluene hydroxylation products.
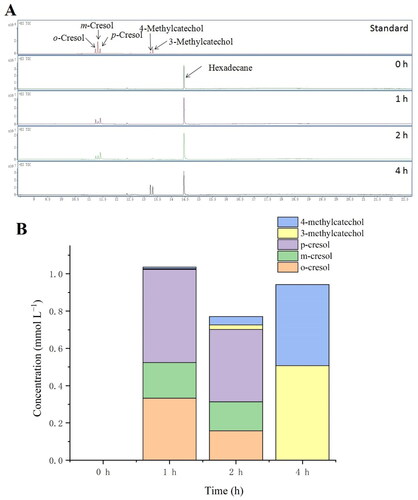
The results showed that the engineered bacteria showed toluene monooxygenase activity by expressing all these optimized genes. Similar results can be obtained with high toluene concentrations (Supplemental Figure S1).
Proteomic analyses to physiological responses of BL-tou exposure to toluene
Protein abundance measurements were compared for the transformant BL-tou and BL-control (expressing empty vector) treated with toluene. A total of 2208 proteins were identified in this study, of which 1998 proteins contained quantitative information. Among these proteins, 550 proteins exhibited statistically significant (P < 0.05) fold changes (≥1.2 fold) in expression levels, with 260 proteins upregulated and 290 proteins downregulated in BL-tou/BL-control comparison. Biological process analysis showed that the majority of the differentially expressed proteins were involved in metabolic, cellular and single-organism processes. Further cellular component analysis revealed that most of the differentially expressed proteins were located in the cell or membrane. Most of the differentially expressed protein carried the molecular function of catalytic activity and binding by a molecular function annotation (). These results demonstrated that engineered E. coli showed significant changes in response to toluene by the expression of exogenous genes encoding ToMO.
Hydroxylation of benzene by transformant BL-tou and intermediate monitoring
To investigate the oxidation of another important substrate further, we treated the transformant BL-tou with 1 mmol L−1 benzene. The successive hydroxylation of benzene occurred in the presence of the transformant BL-tou. To identify the products, we subjected the treated supernatant to HPLC. The retention times of the products were identical with those of the standard phenol, catechol and pyrogallol (). Benzene can be converted to pyrogallol through the three successive steps of hydroxylation (). The results also verified the hydroxylation ability of the transformant BL-tou by using phenol and catechol as substrates, respectively ( and Supplemental Figure S1). However, the hydroxylation rate of catechol by the transformant was the slowest, and the conversion was still incomplete even after 24 h (data not shown). In the presence of the product pyrogallol, it can significantly inhibit catechol hydroxylation by transformant BL-tou ().
Discussion
P. stutzeri OX1 is of interest to researchers because it can grow on toluene, o-xylene, cresols, 2,3-dimethylphenol and 3,4-dimethylphenol as the only carbon and energy source. The enzyme involved in the first step of toluene and o-xylene degradation belongs to the multicomponent ToMO, and the coding genes touABCDEF have been mapped, cloned and sequenced [Citation18, Citation19]. All the six genes are essential for the full activity of the ToMO expressed in E. coli, and each expressed subunit plays a different role in electron transfer. Subunits B, E and A form a subcomplex with hydroxylase activity. The other components, including subunits C (a Rieske-type ferredoxin), D (a small regulatory protein) and F (a NADH oxidoreductase), have different functions [Citation22]. Through steady-state and pre-steady-state kinetics studies, subunit D increases the turnover rate at substoichiometric concentrations, but excess subunit D inhibits steady state catalysis depending on subunit C. A competitive model in which subunits C and D compete for the same binding site on the hydroxylase was presented [Citation32]. Toluene 4-monooxygenase from Pseudomonas mendocina KR1 also has four protein components, which are a di-ion hydroxylase, a cofactor-less effector protein, a soluble Rieske-type ferredoxin, and a NADH oxidoreductase similar to ToMO from P. stutzeri OX1 [Citation33]. To obtain maximum catalysis activity, the relative concentration of each subunit needs to be optimized. A linear relationship was obtained at low ratios of subunits C and D by kinetic measurements, but high ratios cannot promote the reaction rate [Citation22]. For this reason, all genes were driven with a monocistronic transcription pattern for the fine regulation of the expression of each gene in subsequent studies, although the optimization was not involved in this study.
The P. stutzeri OX1 DNA fragments were expressed in different host E. coli. The toluene degradation activity of recombinant bacteria were 0.14–10.00 nmol min−1 mg of protein−1 [Citation18, Citation19]. The degradation rate of transformant BL-tou is higher than that of a coculture of P. putida and P. fluorescens, although the degradation conditions were obviously different [Citation34]. Immobilization of the co-cultured cells can significantly improve their degradation efficiency by tolerating higher concentration of substrates. The different TMOs catalyze the regiospecific hydroxylation of toluene to form o-cresol, m-cresol or p-cresol. The toluene 3-monooxygenase of R. pickettii PKO1 hydroxylates toluene at the meta position. However, the E. coli expressing this toluene 3-monooxygenase oxidizes toluene to 90% p-cresol and 10% m-cresol [Citation35]. The reconstituted toluene 2-monooxygenase from Burkholderia cepacia G4 can oxidize toluene to o-cresol and m-cresol to 3-methylcatechol [Citation36]. In P. mendocina KR1, toluene is initially hydroxylated by its toluene 4-monooxygenase to form p-cresol [Citation37]. The regioselectivity of TMOs can be genetically modified by protein engineering. TMO from B. cepacia G4 can hydroxylate indole at C-3 or C-2 positions to form isatin, indigo and indirubin by DNA shuffling [Citation38]. The mutants of toluene 4-monooxygenase from P. mendocina KR1 produce 20% m-cresol and 80% p-cresol, while the wild-type produces 96% p-cresol [Citation39]. Compared with other four-component aromatic/alkene monooxygenases, ToMO exhibits relaxed regioselectivity. The hydroxylation of toluene by ToMO yields a mixture of o- (36%), m- (19%), and p-cresol (45%) [Citation40]. This finding was similar to the results of the present study.
TMO performs the successive hydroxylation of toluene to form different cresol isomers, and 3- or 4-methylcatechol. This is similar to a phenol hydroxylase from Arthrobacter sp. W1 [Citation41]. The successive hydroxylation and relatively relaxed regiospecificity are of physiological relevance to R. pickettii PKO1. The minor product (m-cresol) formed from toluene oxidation is needed for the induction of the redundant phenol hydroxylase and the lower degradation pathway comprising the meta-cleavage enzymes to enable the full utilization of toluene as a carbon source because o- and p-cresol are not inducers of the two operons [Citation17]. However, relaxed regiospecificity may not be conducive to the subsequent substrate degradation in bioremediation. The regioselectivity of ToMO has been thoroughly investigated for its molecular determinants, and a computational model was developed and used to the synthesis of p-tyrosol from 2-phenylethanol [Citation42, Citation43]. All these studies will contribute to ToMO’s application in the field of bioremediation.
As another usual substrate and an important pollutant, benzene can also be converted to phenol, chatechol and pyrogallol by different TMOs from P. mendocina KR1, R. pickettii PKO1 and B. cepacia G4, respectively [Citation44]. In the present study, E. coli expressing optimized ToMO gene cluster also showed similar reaction, and the predominant product was pyrogallol. However, this finding was different from the results of Cafaro et al. [Citation45]. This is possibly because of the ratio of biocatalyst concentration to substrate concentration similar to the transformation of fluorobenzene by the T4MO from P. mendocina KR1 [Citation46]. The enzymatic reaction of catechol to pyrogallol is theoretically unfavorable to the subsequent ring-opening reaction of microorganisms because catechol is the usual and dominating substrate for this reaction. The inhibition of pyrogallol on this reaction prevents excessive amount of catechol from being converted to pyrogallol.
Organic contaminants exhibit different high toxicities toward bacteria. The inhibitory effects of organic contaminants on microbial metabolism must be considered for biological treatment methods. The specific growth rate of P. putida F1 is inhibited by high toluene concentration [Citation47]. Proteomics analysis showed that toluene has a negative effect on E. coli, which is similar to that of phenol [Citation28]. In the transformant BL-tou, several upregulated enzymes were enriched in the citrate cycle. This result indicated that the transformation of toluene by ToMO can eliminate the inhibition of toluene on the TCA cycle of E. coli. However, several proteins involved in the response to stimuli and defense mechanisms were also upregulated possibly due to the effect of the products cresols or methylcatechols. Thus, the treatment of excreted metabolites or byproducts for biological methods is important. It is also an ideal solution to construct engineered bacteria that completely degrade toluene by adding the core metabolic pathway, and this is the goal of our follow-up research.
Supplemental Material
Download PDF (224.4 KB)Disclosure statement
No potential conflict of the interest was reported by the authors.
Funding
This study was funded by the Key Project Fund of the Shanghai Municipal Committee of Agriculture (2021-02-08-00-12-F00795), Shanghai Academic Technology Research Leader (19XD1432300), National Natural Science Foundation (31901069), and the “Zhu-Pao” Plan of Shanghai Academy of Agricultural Sciences (ZP20211).
Data availability
All data that support the findings reported in this study are available from the corresponding author upon reasonable request.
References
- Rajamanickam R, Kaliyamoorthi K, Ramachandran N, et al. Batch biodegradation of toluene by mixed microbial consortia and its kinetics. Int Biodeter Biodegr. 2017;119:282–288.
- Jacob JH, Irshaid FI. Toluene biodegradation by novel bacteria isolated from polluted soil surrounding car body repair and spray painting workshops. JEP. 2015;6(12):1417–1429.
- Morales P, Cáceres M, Scott F, et al. Biodegradation of benzo[α]pyrene, toluene, and formaldehyde from the gas phase by a consortium of Rhodococcus erythropolis and Fusarium solani. Appl Microbiol Biotechnol. 2017;101(17):6765–6777.
- Barro R, Regueiro J, Llompart M, et al. Analysis of industrial contaminants in indoor air: Part 1. Volatile organic compounds, carbonyl compounds, polycyclic aromatic hydrocarbons and polychlorinated biphenyls. J Chromatogr A. 2009;1216(3):540–566.
- Cao B, Nagarajan K, Loh KC . Biodegradation of aromatic compounds: current status and opportunities for biomolecular approaches. Appl Microbiol Biotechnol. 2009;85(2):207–228.
- Bolden AL, Kwiatkowski CF, Colborn T. New look at BTEX: are ambient levels a problem? Environ Sci Technol. 2015;49(9):5261–5276.
- Blasi B, Tafer H, Kustor C, et al. Genomic and transcriptomic analysis of the toluene degrading black yeast Cladophialophora immunda. Sci Rep. 2017;7(1):11436.
- Ortega-Gonzalez DK, Zaragoza D, Aguirre-Garrido J, et al. Degradation of benzene, toluene, and xylene isomers by a bacterial consortium obtained from rhizosphere soil of Cyperus sp. grown in a petroleum-contaminated area. Folia Microbiol. 2013;58(6):569–577.
- Kuppardt A, Kleinsteuber S, Vogt C, et al. Phylogenetic and functional diversity within toluene-degrading, sulphate-reducing consortia enriched from a contaminated aquifer. Microb Ecol. 2014;68(2):222–234.
- Olapade OA, Ronk AJ. Isolation, characterization and community diversity of indigenous putative toluene-degrading bacterial populations with catechol-2,3-dioxygenase genes in contaminated soils. Microb Ecol. 2015;69(1):59–65.
- Yoshikawa M, Zhang M, Toyota K. Biodegradation of volatile organic compounds and their effects on biodegradability under co-existing conditions. Microbes Environ. 2017;32(3):188–200.
- Fulekar MH, Koul Sheetal TNG, Darshana N. Bioremediation of toluene using microbial consortium obtained from industrial effluent. Int J Curr Microbiol App Sci. 2017;6(2):552–558.
- Estevez E, Veiga MC, Kennes C. Biodegradation of toluene by the new fungal isolates Paecilomyces variotii and Exophiala oligosperma. J Ind Microbiol Biotechnol. 2005;32(1):33–37.
- Jayamani I, Cupples AM. Effect of isobutanol on toluene biodegradation in nitrate amended, sulfate amended and methanogenic enrichment microcosms. Biodegradation. 2013;24(5):657–663.
- Yoshikawa M, Zhang M, Kurisu F, et al. Bacterial degraders of coexisting dichloromethane, benzene, and toluene, identified by stable-isotope probing. Water Air Soil Pollut. 2017;228(11):418.
- Yoshikawa M, Zhang M, Toyota K. Integrated anaerobic-aerobic biodegradation of multiple contaminants including chlorinated ethylenes, benzene, toluene, and dichloromethane. Water Air Soil Pollut. 2017;228(1):25.
- Fishman A, Tao Y, Wood TK. Physiological relevance of successive hydroxylations of toluene by toluenepara-monooxygenase of Ralstonia pickettii PKO1. Biocatal Biotransform. 2004;22(4):283–289.
- Bertoni G, Martino M, Galli E, et al. Analysis of the gene cluster encoding toluene/o-xylene monooxygenase from Pseudomonas stutzeri OX1. Appl Environ Microbiol. 1998;64(10):3626–3632.
- Bertoni G, Bolognese F, Galli E, et al. Cloning of the genes for and characterization of the early stages of toluene and o-xylene catabolism in Pseudomonas stutzeri OX1. Appl Environ Microbiol. 1996;62(10):3704–3711.
- Chauhan S, Barbieri P, Wood T. Oxidation of trichloroethylene, 1,1-dichloroethylene, and chloroform by toluene/o-xylene monooxygenase from Pseudomonas stutzeri OX1. Appl Environ Microbiol. 1998;64(8):3023–3024.
- Ryoo D, Shim H, Canada K, et al. Aerobic degradation of tetrachloroethylene by toluene-o-xylene monooxygenase of Pseudomonas stutzeri OX1. Nat Biotechnol. 2000;18(7):775–778.
- Cafaro V, Scognamiglio R, Viggiani A, et al. Expression and purification of the recombinant subunits of toluene/o-xylene monooxygenase and reconstitution of the active complex. Eur J Biochem. 2002;269(22):5689–5699.
- Kahlon RS. 2016. Biodegradation and bioremediation of organic chemical pollutants by Pseudomonas. In Kahlon RS, editor. Pseudomonas: Molecular and Applied Biology. Berlin: Springer. pp. 343–417.
- Wells T, Jr., Ragauskas AJ . Biotechnological opportunities with the β-ketoadipate pathway. Trends Biotechnol. 2012;30(12):627–637.
- Abu Laban N, Tan B, Dao A, et al. Draft genome sequence of uncultivated toluene-degrading Desulfobulbaceae bacterium Tol-SR, obtained by stable isotope probing using [13C6]toluene. Genome Announc. 2015;3:e01423-14.
- Bouhajja E, McGuire M, Liles MR, et al. Identification of novel toluene monooxygenase genes in a hydrocarbon-polluted sediment using sequence- and function-based screening of metagenomic libraries. Appl Microbiol Biotechnol. 2017;101(2):797–808.
- Tancsics A, Farkas M, Horvath B, et al . Genome analysis provides insights into microaerobic toluene-degradation pathway of Zoogloea oleivorans Buc(T). Arch Microbiol. 2020;202(2):421–426.
- Wang B, Xu J, Gao J, et al. Construction of an Escherichia coli strain to degrade phenol completely with two modified metabolic modules. J Hazard Mater. 2019;373:29–38.
- Wang B, Gao J, Xu J, et al. Optimization and reconstruction of two new complete degradation pathways for 3-chlorocatechol and 4-chlorocatechol in Escherichia coli. J Hazard Mater. 2021;419:126428.
- Xiong A, Yao Q, Peng R, et al. A simple, rapid, high-fidelity and cost-effective PCR-based two-step DNA synthesis method for long gene sequences. Nucleic Acids Res. 2004;32(12):e98–e98.
- Peng R, Xiong A, Yao Q. A direct and efficient PAGE-mediated overlap extension PCR method for gene multiple-site mutagenesis. Appl Microbiol Biotechnol. 2006;73(1):234–240.
- Liang AD, Lippard SJ. Component interactions and electron transfer in toluene/o-xylene monooxygenase. Biochemistry. 2014;53(47):7368–7375.
- Bailey LJ, Elsen NL, Pierce BS, et al. Soluble expression and purification of the oxidoreductase component of toluene 4-monooxygenase. Protein Expr Purif. 2008;57(1):9–16.
- Shim H, St Y. Biodegradation of benzene, toluene, ethylbenzene, and o-xylene by a coculture of Pseudomonas putida and Pseudomonas fluorescens immobilized in a fibrous-bed bioreactor. J Bacteriol. 1999;67:99–112.
- Fishman A, Tao Y, Wood TK. Toluene 3-monooxygenase of Ralstonia pickettii PKO1 is a para-hydroxylating enzyme. J Bacteriol. 2004;186(10):3117–3123.
- Newman LM, Wackett LP. Purification and characterization of toluene 2-monooxygenase from Burkholderia cepacia G4. Biochemistry. 1995;34(43):14066–14076.
- Whited GM, Gibson DT. Toluene-4-monooxygenase, a three-component enzyme system that catalyzes the oxidation of toluene to p-cresol in Pseudomonas mendocina KR1. J Bacteriol. 1991;173(9):3010–3016.
- Rui L, Reardon KF, Wood TK. Protein engineering of toluene ortho-monooxygenase of Burkholderia cepacia G4 for regiospecific hydroxylation of indole to form various indigoid compounds. Appl Microbiol Biotechnol. 2005;66(4):422–429.
- Fishman A, Tao Y, Bentley WE, et al. Protein engineering of toluene 4-monooxygenase of Pseudomonas mendocina KR1 for synthesizing 4-nitrocatechol from nitrobenzene. Biotechnol Bioeng. 2004;87(6):779–790.
- Cafaro V, Notomista E, Capasso P, et al. Regiospecificity of two multicomponent monooxygenases from Pseudomonas stutzeri OX1: molecular basis for catabolic adaptation of this microorganism to methylated aromatic compounds. Appl Environ Microbiol. 2005;71(8):4736–4743.
- Ma F, Shi SN, Sun TH, et al. Biotransformation of benzene and toluene to catechols by phenol hydroxylase from Arthrobacter sp. W1. Appl Microbiol Biotechnol. 2013;97(11):5097–5103.
- Notomista E, Cafaro V, Bozza G, et al. Molecular determinants of the regioselectivity of toluene/o-xylene monooxygenase from Pseudomonas sp. strain OX1. Appl Environ Microbiol. 2009;75(3):823–836.
- Notomista E, Scognamiglio R, Troncone L, et al. Tuning the specificity of the recombinant multicomponent toluene o-xylene monooxygenase from Pseudomonas sp. strain OX1 for the biosynthesis of tyrosol from 2-phenylethanol. Appl Environ Microbiol. 2011;77(15):5428–5437.
- Tao Y, Fishman A, Bentley WE, et al. Oxidation of benzene to phenol, catechol, and 1,2,3-trihydroxybenzene by toluene 4-monooxygenase of Pseudomonas mendocina KR1 and toluene 3-monooxygenase of Ralstonia pickettii PKO1. Appl Environ Microbiol. 2004;70(7):3814–3820.
- Cafaro V, Izzo V, Scognamiglio R, et al. Phenol hydroxylase and toluene/o-xylene monooxygenase from Pseudomonas stutzeri OX1: interplay between two enzymes. Appl Environ Microbiol. 2004;70(4):2211–2219.
- Nolan LC, O’Connor KE. Use of Pseudomonas mendocina, or recombinant Escherichia coli cells expressing toluene-4-monooxygenase, and a cell-free tyrosinase for the synthesis of 4-fluorocatechol from fluorobenzene. Biotechnol Lett. 2007;29(7):1045–1050.
- Bordel S, Munoz R, Diaz LF, et al. New insights on toluene biodegradation by Pseudomonas putida F1: influence of pollutant concentration and excreted metabolites. Appl Microbiol Biotechnol. 2007;74(4):857–866.