Abstract
Enzymes have been widely studied for their excellent efficiency, selectivity and environmentally benign nature. However, the development of their biocatalytic applications is severely limited by the lack of stability and reusability. Nanostructured materials have been demonstrated as efficient hosts for the immobilization of enzyme due to their large surface areas as well as higher activities and long-term stability. Furthermore, nanomaterials with enzyme-like characteristics are explored as highly stable and low-cost alternatives to mimic the structures and functions of naturally occurring enzymes. This review offers an overview on the research status of enzymatic immobilization adapted from nanostructured materials and nanozymes in numerous fields, from biosensing and biocatalysis to environmental remediation, with emphasis focused on current challenges and future directions in highly selective and efficient biocatalysis.
Introduction
Biological catalysis is the application of enzymes, microbial cells or plant and animal cells as biocatalysts for the catalytic reaction [Citation1]. The spectacular advances of biocatalysis in the faster reaction rates, higher substrate specificity and greener reaction process (mild pH, temperature and pressure) have led to an exponential growth in the number of enzyme catalysts. However, they also have disadvantages such as unstable structure, aggregation and high cost of enzyme separation and purification [Citation2–4]. Therefore, the immobilization of enzymes is a promising technique to allow the catalysts to be retained within the reactor while reagents flow through the system. Enzyme immobilization decreases the required quantity of enzyme, simplifies product work-up and potentially increases enzyme stability [Citation5, Citation6]. At present, the preparation of immobilized enzymes has been generally classified into two major categories including physical and chemical immobilization methods such as adsorption, entrapment [Citation7, Citation8], covalent binding and cross-linking [Citation9, Citation10] ().
Figure 1. Representive methods applied during the enzymatic immobilization. Absorption (a). Entrapment (b). Covalent attachment (c). Cross-linking (d).
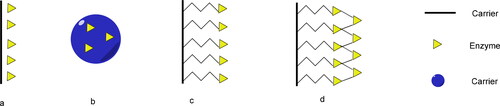
Nanostructured material is a key issue for enzymes immobilization. The immobilized materials are generally organic macromolecular polymers (Chitosan, cellulose) or inorganic materials (SiO2) in the past [Citation11]. However, the stability of enzymes immobilized in these materials is relatively low, and due to the small surface area, their enzyme load has been limited. Nanomaterials have desirable characteristics such as their good biocompatibility, large surface area, high mass transfer efficiency, high enzyme load, hardness and defined geometry [Citation3, Citation5, Citation12–15]. At present, nanomaterials are used for the immobilization of enzymes including magnetic nanomaterials [Citation16] and non-magnetic nanomaterials, in which non-magnetic nanomaterials can be divided into inorganic nanoparticles (Au nanoparticles, mesoporous silica nanoparticle, carbon nanotubes) [Citation17–19], metal-organic frameworks (MOFs) [Citation7, Citation20].
Some nanomaterials with enzyme-like kinetics characteristics can efficiently catalyze specific reactions under mild conditions, and are termed nanozymes [Citation21]. Studies have attempted to apply them to manipulate enzymatic reactions in a wide range of applications [Citation22–24]. In 2007, Yan’s group first reported that the Fe3O4 magnetic nanoparticles (MNPs) had peroxidase-like activity [Citation25]. The MNPs had Michaelis–Menten kinetics characteristics similar to the natural enzyme, and the affinity (KM) with TMB (3,3′,5,5′-tetramethylbenzidine) was even 40 times higher than that of horseradish peroxidase (HRP) [Citation26]. At present, the nanomaterials with enzyme-like activity include metal elements, metal oxides, carbon-based frameworks and MOFs [Citation27–30]. Different types of enzyme activities have been mimicked by nanomaterials including peroxidase, catalase, oxidase and superoxide dismutase (SOD) (). The characteristics of nanomaterials as enzymes are extraordinarily suitable for large-scale applications which have high stability, excellent reusability, low cost and green nature.
Figure 2. Recent applications of nanozymes and proposed mechanisms (A: substrate; POD: peroxidase; CAT: catalase; OXD: oxidase; SOD: superoxide dismutase.). Reprinted with permission from Ref. [Citation21], Huang, Y., Ren, J. and Qu, X., 2019. Nanozymes: Classification, catalytic mechanisms, activity regulation, and applications. Chemical Review, 119(6), pp.4357–4412.
![Figure 2. Recent applications of nanozymes and proposed mechanisms (A: substrate; POD: peroxidase; CAT: catalase; OXD: oxidase; SOD: superoxide dismutase.). Reprinted with permission from Ref. [Citation21], Huang, Y., Ren, J. and Qu, X., 2019. Nanozymes: Classification, catalytic mechanisms, activity regulation, and applications. Chemical Review, 119(6), pp.4357–4412.](/cms/asset/81b9b122-3fdf-42f9-887e-369debfe5fae/tbeq_a_2054727_f0002_c.jpg)
lists both the advantages and disadvantages of nano-carrier immobilized enzymes and nanozymes. This review begins with a survey of the recent developments and application of the two types of catalysts. The challenges and prospects are also discussed.
Table 1. Advantages and disadvantages of enzyme immobilization and nanozyme.
Nano-carrier immobilized enzymes
Immobilization of enzymes is a promising solution to overcome the disadvantages of the natural enzymes. The stability of enzymes can be greatly improved by physical adsorption or covalent crosslinking, and the enzymes can also be recycled through simple methods. Moreover, a relative high enzyme-load can be achieved by benefiting from their high surface area. As shown in , nanomaterials have been used to immobilize enzymes such as horseradish peroxidase (HRP), laccase, versatile peroxidase, polyphenol oxidase and β-galactosidase. These immobilized enzymes have been used in the fields of hydrogen peroxide (H2O2) detection, depolymerization of lignocellulosic biomass into vanillin, oxidation of phenolic compounds and transglycosylation of lactose.
Table 2. The applications and performances of nanomaterial immobilized enzymes.
Magnetic nanomaterials
Magnetic nanomaterials are composed of iron oxide nanoparticles (NPs) with superparamagnetic properties, which have been extensively studied due to their stability and easy separation [Citation56]. The enzymes are immobilized on magnetic nanomaterial by covalent bonds and their stability can be improved in complex environments, which could reduce the leakage of enzymes and their cost of industrial application, and improve their catalytic activity [Citation57]. With an external magnetic field, they can be separated easily and rapidly [Citation58].
Sardaremelli et al. [Citation31] reported the use of Fe3O4 magnetic nanoparticles (MNPs) which were coated with polydopamine (PDA-MNPs) to support HRP for the detection of H2O2. The PDA-MNPs were cast on the surface of the glassy carbon electrode (GCE) that was modified by poly (L-arginine/toluidine blue), so that they could be separated and recycled easily. With the excellent specificity of HRP, the bio-electrochemical sensor has the detection range of 0.5–30 μmol/L with a low limit of 0.23 μmol/L. And there is no significant change in the detection reproducibility after 30 cycles.
Chlorophenol represents an important intermediate of pesticides that is difficult to degrade in the environment and would get enriched in organisms through natural circulation. Therefore, it would irritate the skin and mucous membrane, and even seriously damage the nervous and respiratory system [Citation16]. Zhang et al. [Citation16] completed the immobilization of laccase with chitosan-coated Fe3O4 MNPs (Laccase-Fe3O4@CTS), which then oxidize chlorophenols to produce quinones to achieve the rapid biodegradation of chlorophenol (). Chitosan could introduce a large number of amino groups on the surface of Fe3O4 MNPs, which could generate electrostatic repulsion between nanoparticles to prevent agglomeration. Not only that, chitosan also could be used for immobilization of laccase by forming covalent bonds with laccase molecules. The immobilized laccase could preserve 75.2% of the initial activity after 4 weeks of storage at 4 °C, whereas the free laccase preserved only 40.2%. The oxidation results of chlorophenol showed that 91.4% and 75.5% of 2,4-dichlorophenol (2,4-DCP) and 4-chlorophenol (4-CP) could be converted by Laccase-Fe3O4@CTS at room temperature for 12 h, respectively. Furthermore, Laccase-Fe3O4@CTS could still achieve 75.8% and 57.4% conversion rates after 10 cycles.
Liu et al. [Citation34] used multiwalled carbon nanotubes (MWNTs) and Fe3O4 MNPs to form magnetic multiwalled carbon nanotubes (M-MWNTs), and then immobilized the porcine pancreas lipase (PPL) on the surface of M-MWNTs (PPL-M-MWNTs) through hydrophobic interaction, which was used for the synthesis of 2H-chromenes (). Lipase was adsorbed on the hydrophobic surface, and the “lid" of the enzyme could be opened through interfacial activation mechanism, so the active center was fully exposed thus improving the catalytic performance. The synthesis of 2H-chromenes by using enzymes could solve the shortcomings of chemical processes, such as low yield, long reaction time and complex reactions, and it is suitable for a variety of substrates. Nevertheless, the enzymes were immobilized on M-MWNTs through physical absorption, which is why they were prone to leakage. These results indicate that the yield of 2H-chromenes was significantly reduced after 5 cycles. Therefore, M-MWNTs can form multi-point covalent connection with PPL in the future to improve the fixation capacity and reduce the enzyme leakage.
Figure 4. The synthesis of 2H-chromenes. Reprinted with permission from [Citation34].
![Figure 4. The synthesis of 2H-chromenes. Reprinted with permission from [Citation34].](/cms/asset/a20fa228-a999-4374-8ca6-90b82f2ef6a1/tbeq_a_2054727_f0004_c.jpg)
Saikia et al. [Citation36] co-immobilized laccase and versatile peroxidase in magnetic silica microspheres (MSMS) to depolymerize waste lignin into an important flavoring agent vanillin (). Laccase had the ability to depolymerize the phenolic compounds of lignin and versatile peroxidase could oxidize phenolic and non-phenolic compounds, which could be obtained in high yield by the synergistic action of two enzymes. Under the optimal conditions (pH = 6, 6 h, and 30 °C), the yield of vanillin was improved up to 27.1%, and was higher than individual laccase and peroxidase system. Based on the synergistic effect of the two enzymes, lignin can be converted into important chemical raw materials to realize the efficient utilization, which provides a facile access to the recycling of a large amount of waste lignin.
Gold nanoparticles
Gold nanoparticles (Au NPs) are suitable for electrochemical detection due to their unique high conductivity. Their application has been widely studied in the fields of environmental pollutants and veterinary drug residues detection.
Zhang et al. [Citation37] developed a novel laccase based electrochemical biosensor for the detection of catechol. They created a laccase-based biosensor by a new nanocomposite that self-assembled of AuNPs and MoS2 nanosheets, and Nafion, an effective electrochemical binder, was used for immobilization of the laccase. MoS2 is a two-dimensional (2 D) layered nanomaterial with some properties of good biocompatibility and mechanical strength, high specific surface area, easy recyclability but poor conductivity. AuNPs not only could immobilize enzymes, but also have excellent conductivity which could effectively improve the sensitivity of the electrochemical sensor and reduce the detection limit. The combination of the two materials can make up for their respective shortcomings. The detection range of the electrochemical sensor was 2–2000 μmol/L and the limit of detection (LOD) was 2 μmol/L (S/N = 3). After repeating the test 10 times, the relative standard deviation (RSD) of the detection results was 1.2%, indicating a good reproducibility of results. With the help of Nafion, the biosensor could still retain 90% of its initial value after storage at 4 °C for one month, indicating that the stability had been enhanced effectively.
Luo et al. [Citation38] immobilized horseradish peroxidase (HRP) on Au NPs for the detection of chloramphenicol (CAP) in food (). The concentration of CAP in samples was rapidly detected by using flow injection chemiluminescence immunoassay (FI-CLIA) with a syringe pump. The detection range was 0.001–10 ng/mL (R2= 0.9961) and the LOD was 0.33 pg/mL. Compared with other detection methods with large equipment, such as GC-MS with a detection limit of 0.05 ng/mL, this method had a lower LOD and was more convenient. The recovery rates of the standard addition method in shrimp and honey samples were in the range of 83.5%–99.6%, indicating that this method could be used for accurate detection of real samples.
Figure 6. The detection of chloramphenicol (CAP). Reprinted with permission from Ref. [Citation38].
![Figure 6. The detection of chloramphenicol (CAP). Reprinted with permission from Ref. [Citation38].](/cms/asset/9ed73b54-5eae-474f-ba9f-85e7fffe273f/tbeq_a_2054727_f0006_c.jpg)
Mesoporous silica
Mesoporous silica has the advantages of large specific surface area, easy surface modification, excellent chemical inertia and biocompatibility, as well as certain mechanical strength. The micropores on the surface are suitable for the transfer of substrates, and further improve the stability of enzymes [Citation59].
Jo et al. [Citation60] used a template-free interfacial condensation method to synthesize the hollow silica nanocapsules to embed enzymes. The efficiency of coupled enzymatic reactions could be enhanced by constructing multi-enzymes into hollow silica nanocapsules together. The SiO2 membrane could protect the enzymes from the harsh environment and reduce leakage. It did not limit the mass transfer of substrates, which ensured the catalytic efficiency of enzymes.
Mukundan et al. [Citation61] used spray drying method to synthesize silica microcapsules by silica nanoparticles, and at the same time entrapped the S. lactis cells in them. The cells expressed β-galactosidase activity that could catalyse the hydrolysis of lactose into glucose and galactose. The immobilized cells had the higher activity of lactose hydrolysis than free cells (KM=8.33 mmol/L and 4.16 mmol/L, Vmax= 71.43 μmol/L min and 125 μmol/L min for free cells and immobilized cells, respectively). This may be because part of the enzyme was released during the encapsulation process, and the catalytic activity was improved.
Elias et al. [Citation43] prepared nanocellulose (NC) and nano-sized SiO2 by the sustainable biomaterials-oil palm leaves (OPL). Then a nanofiller was synthesized by using NC modified SiO2 NPs to immobilize Candida rugosa lipase (CRL). Finally, the nanofiller has combined with polyethersulfone (PES) to form a membrane material (NC-SiO2-PES/CRL) with stronger mechanical stability which was used for the synthesis of pentyl valerate (PeVa) (). The results showed that the yield of PeVa could reach 91.3% within 3 h, which had great advantages in terms of cost and energy consumption compared to previously developed biocatalysts (reaction need 17 h or even 7–8 days).
The advantages of mesoporous SiO2 nanomaterials have also been reflected by the research of Jia et al [Citation44]. They prepared mesoporous silica nanoflowers by a reverse microemulsion method to immobilize lipase and used them for the synthesis of ethyl levulinate (). The yield of ethyl levulinate achieved by immobilized lipase was 99.5% at 40 °C for 8 h, which was much higher than that by free lipase (67.9%). The yield could be preserved (68%) after 7 cycles, while the free enzymes or inorganic acid cannot be recycled.
Wu et al. [Citation45] reported the immobilized phospholipase D (PLD) using macroporous SiO2 NPs modified by the epoxy resin-based cationic polymer polyethylenimine (PEI) as a carrier. Phosphatidylserine (PS) could be synthesized from phosphatidylcholine (PC) by using the PLD (). The cationic and amphiphilic PEI coating had a good affinity not only to negatively charged PLD molecules but also to water-insoluble PC, which increased the stability of enzymes and the concentration of substrates around enzymes. A great impact of pH was observed during the immobilization of enzymes. The isoelectric point of PLD was about 5.39 and when pH = 5.39 ∼ 7.00, it could be well adsorbed with PEI coating. When pH > 7.00, the negative charges on the surface of PLD would generate electrostatic repulsion between the two enzyme molecules. The yield of PS catalyzed by immobilized PLD reached 96.20%, which was much higher than the free PLD (68.10%). More importantly, the yield could still achieve 79.30% after 12 cycles.
Metal–organic frameworks (MOFs)
Metal-organic frameworks (MOFs) are a type of porous materials that are composed of metal nodes and organic frameworks [Citation62]. Compared with traditional porous materials, MOFs have much higher porosity and specific surface area [Citation63]. Furthermore, they can be synthesized by a variety of metals and organic substances. There are two types of enzyme immobilization methods with MOFs: (1) to immobilize enzymes on the surface of MOFs by physical adsorption or covalent crosslinking, (2) to encapsulate enzymes in the cavities of MOFs.
Yuan et al. [Citation49] prepared UiO-66 with zirconium chloride and terephthalic acid, then used it to immobilize the Pseudomonas fluorescence lipase (PFL) that was coated with polyethylene glycol (PFL-PEG-UiO-66). The immobilized PFL had been used for the kinetic resolution of 2-(4-hydroxyphenyl) propionic acid ethyl ester enantiomers (2-HPPAEE) and 4-methoxymandelic acid enantiomers (4-MMA) (). PFL was successfully immobilized on the surface of UiO-66 by the covalent cross-linking action and this prevented the enzymes from aggregation. Because the diameter of PFL was larger than the micropores on the surface of UiO-66, the PFL molecules could not enter the interior of UiO-66. The results of the kinetic study showed that PFL-PEG-UiO-66 had a higher affinity for the substrates than free PFL (KM=69.07 mmol/L vs. 117.43 mmol/L). This might be due to the changes in the structure of enzymes during the immobilization process, making the active center more accessible. The conversion rates could achieve 47% and 49% for 2-HPPAEE (ee > 99%) and 4-MMA (ee > 99%), respectively. However, the enzymes immobilized on the surface of MOFs were exposed to the external environment that would cause enzymes leakage or inactivation. The data showed that the loss of 2-HPPAEE conversion rate was only 2.5%–5% in the first four cycles of the enzymes, but rapidly decreased to 18.5% in the next two cycles. Similarly, the conversion rate of 4-MMA quickly dropped to 13% in the fifth cycle.
Figure 9. The kinetic resolution of 2-(4-hydroxyphenyl) propionic acid ethyl ester (2-HPPAEE) enantiomers and 4-methoxymandelic acid(4-MMA) enantiomers.
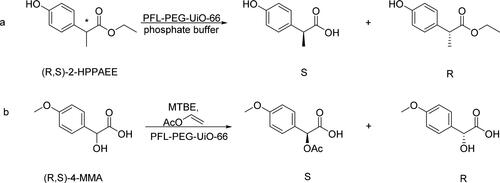
The second method is designed to embed enzymes in the internal cavities of the MOFs to maintain the stability of the enzymes and stop the leakage. However, this method is not suitable for the enzymes whose diameters are larger than the micropores diameter on the surface of MOFs.
Li et al. [Citation52] prepared single-crystal ordered macroporous ZIF-8 (SOM-ZIF-8) by adding 3 D-polystyrene sphere (PS) template during the synthetic preparation of ZIF-8. The diameter of micropores on the surface of ZIF-8 was expanded, while the inner micropores were retained. Therefore, HRP could directly enter the interior of SOM-ZIF-8 through macropores on the surface and be trapped in the interior cavities. The immobilized HRP was used for biodegradation of hazardous dyes (methyl orange, Congo red, rhodamine B and rhodamine 6 G) and could retain 85% of its initial activity after 5 cycles. Compared with enzymes immobilized by covalent cross-linking, the reusability of enzymes, in this case, was improved effectively.
There was a new type of method defined as in-situ growth, in which enzymes were encapsulated in the MOFs in situ during the formation process of MOFs. And the micropores of MOFs could provide ample channels for the mass transfer of substrates [Citation53].
Huang et al. [Citation54] encapsulated glucose oxidase (GOx) within Fe-ZIF-8(GOx@Fe-ZIF-8) by this method. The Fe-ZIF-8 had peroxidase-like activity and could be used to detect glucose in combination with GOx (). The addition of Fe2+ had three main functions: (1) to expand the pore size of ZIF-8, (2) to generate the Fe-GOx complex and increase the enzyme load, (3) to accelerate the formation of ZIF-8 and make it with peroxidase-like activity. The cascade reactions catalyzed by GOx and Fe-ZIF-8 were completed in the same space, and the catalytic efficiency was synergistically enhanced. The glucose concentrations in multiple samples were measured by GOx@Fe-ZIF-8 and a glucometer, and the results from both were similar (RSD = 2.4%–17.4%), indicating that it was suitable for the detection of real samples.
Nanozymes
Immobilization of enzymes on nanomaterials can partly solve the shortcomings of natural enzymes, such as reusability and stability. However, the inherent protein structure of natural enzymes cannot exist stably under extreme conditions and can easily be decomposed by proteases. At the same time, the costs of separation and purification are relatively high. To some extent, their large-scale applications have been limited by the drawbacks above. With the development of nanotechnology, the inherent enzyme-like activities of nanomaterials have attracted a lot of attention. As shown in , nanozymes have been applied in many fields such as tumor therapy, chiral catalysis, cell protection, antibacterial activity, biosensor, environmental protection [Citation64, Citation76, Citation87, Citation101–103]. In the following part, the research progress and applications of nanozymes in the past two years will be described according to the classification of materials.
Table 3. Nanomaterials with enzyme-like activities.
Metal based
Compared with single-metal complexes, multi metal complexes could exhibit synergistic catalytic activity and enhance the catalytic efficiency. For example, Au-Pt NPs showed much higher peroxidase-like activity than PtNPs or AuNPs alone [Citation104]. AuNPs have been widely used as a sensitizer in tumor radiation therapy due to their extensive biocompatibility, stability and strong photoelectric absorption ability. However, their non-specificity in cells may cause damage to normal cells.
In 2020, Chong et al. [Citation64] prepared Au-Ag NPs modified with hyaluronic acid (Au-Ag@HA NPs) for enhancing the therapeutic effect of tumor radiotherapy. Through the surface modification of hyaluronic acid (HA), the CD44 receptor that was overexpressed on the surface of 4T1 breast cancer cells was targeted by Au-Ag NPs, while the concentration of Au-Ag NPs in tumor cells was increased and their damage to normal cells was reduced.
Au-Ag NPs showed peroxidase (POD) activity in the acidic microenvironment of tumor cells. When Au-Ag NPs got into the tumor cells, abundant ROS (·OH) could be generated by the reduction of H2O2 via the Fenton-like reaction and X-ray irradiation. The highly reactive ROS could not only kill tumor cells, but also further facilitate the generation of toxic Ag+ from Au-Ag NPs, resulting in synergistically enhanced antitumor efficacy (). When without Au-Ag NPs, the tumor therapeutic was limited and almost depends on the inefficient decomposition of H2O2 to ·OH by X-ray irradiation. More importantly, Au-Ag NPs displayed superoxide dismutase (SOD)-like activity in the neutral environment of normal cells, which could scavenge ROS and protect normal cells. There are numerous metal-metal and metal-nonmetal complexes that have been reported toward excellent therapeutic effects on tumors, such as Au-Pt NPs and Pt-C NPs [Citation65, Citation66].
Figure 11. Schematic illustration shows synergetic multimodal for tumor therapy by Au-Ag@HA nanoparticles. Reprinted with permission from [Citation64].
![Figure 11. Schematic illustration shows synergetic multimodal for tumor therapy by Au-Ag@HA nanoparticles. Reprinted with permission from [Citation64].](/cms/asset/eeb78c81-ebb7-491a-a5dc-935214e09046/tbeq_a_2054727_f0011_c.jpg)
Natural enzymes used in enzyme-linked immunosorbent assay (ELISA) have some drawbacks such as poor stability and difficult storage. Therefore, nanozyme-linked immunosorbent assay (NLISA) was developed. It uses nanozymes to replace natural enzymes [Citation67].
The noble metal Pt has excellent peroxidase-like activity and is used for the detection of a variety of substrates. However, the large-scale application of Pt is limited by its high costs. Thus, the application of Pt can be reduced by mixing Pt with other cheap metals to form multi-metal complexes. Gupta et al. [Citation68] developed novel citric acid-functionalized Pt-Ni hollow nanospheres (CA@Pt-Ni hNS) to replace HRP for the ELISA of human serum albumin (HSA). Citric acid (CA) not only stabilized Pt/Ni hNS, but also provided a negative surface charge of hNS for the attraction of the chromogenic substrate TMB. CA@Pt-Ni hNS showed stronger affinity on both TMB and H2O2 than HRP (KM=0.18, 2.5 mmol/L vs. 0.43, 3.0 mmol/L for CA@Pt-Ni hNS and HRP, respectively), and the NLISA showed better detection performance and stability than ELISA. In other reports, nanozymes applied in detection almost all showed superior performance and stability than natural enzymes. For example, Au-Pt NPs for the detection of aflatoxin B1[72], Au-Mag SiO2 for the detection of glucose in human whole blood [Citation69], Au-Pt NPs for the detection of Ag+ [Citation72].
In addition to noble metals, some nanozymes composed of cheap metals such as copper and iron have also been reported. Du et al. [Citation73] prepared a nanozyme by embedding Fe-Cu dual atomic sites in three-dimensional porous N-doped carbon (Fe Cu-DA/NC), which has cytochrome c oxidase (CcO) activity and can be used to accelerate the oxygen reduction reaction (ORR). ORR is a chemical reaction occurring in some batteries and its slow kinetics limits the performance of batteries. The electrocatalytic ORR activity of Fe Cu-DA/NC is comparable to or even better than that of commercial Pt/C. In addition, the Fe Cu-DA/NC showed better long-term stability and superior resistance to methanol poisoning than Pt/C in both alkaline and acidic environments.
Metallic oxide based
Substrate specificity is one of the most important properties of natural enzymes, which means enzymes can catalyze only one of a variety of substances. This is one of the most common differences between nanozymes and natural enzymes, because pure nanomaterials exhibit little substrate specificity. Chiral separation of racemates and asymmetric catalysis of substrates are usually crucial in the process industrial pharmaceutical-production. Therefore, to narrow the gap between nanozymes and natural enzymes, methods intend to improve the substrate specificity, especially the enantioselectivity of nanozymes [Citation101], such as modifying chemical compounds, amino acids or DNA on the surface of nanozymes.
Zhou et al. [Citation78] prepared a series of chiral nanozymes (Fe3O4@Poly(AA)) by using Fe3O4 NPs as a catalytic center and adopting different kinds of amino acids-appended chiral polymers as a chiral selector. The surface of Fe3O4 was covered with a layer of SiO2, and then the chiral amino acid polymer was linked to the surface of SiO2, finally the SiO2 layer was removed in a high-concentration NaOH solution to form the yolk-shell structure Fe3O4@Poly(AA) NPs. The advantage of this structure was that a lot of chiral ligands could be introduced into the shell to improve the chiral selectivity. Moreover, the chiral ligands and Fe3O4 NPs were not directly linked through covalent bonds, which avoided shielding the catalytic sites of Fe3O4 NPs. Thus, peroxidase-like activity was similar to that of pure Fe3O4 NPs. After introducing D/L-tryptophan (D/L-Trp) to the surface of Fe3O4 NPs yolk (Fe3O4@Poly(D/L-Trp)), D/L-tyrosinol could be selectively transformed into the corresponding D/L-dityrosinol (ee > 99%) (). The kcat/KM values showed that Fe3O4@Poly(D-Trp) was 5.38-fold more active to D-tyrosinol than to L-tyrosinol. And the selectivity of Fe3O4@Poly(D-Trp) was higher than HRP (4.77-fold).
Nanozymes are suitable for the detection of substrates due to their properties such as high catalytic activity and stability. There have been many reports on the application of metallic oxide based nanozymes in the fields of detection. Zhang et al. [Citation79] prepared Fe3O4@C nanowires (Fe3O4@C NWs) with peroxidase-like activity. After combining with a biotin-labeled aptamer, they could be used for the ultra-sensitive detection of platelet-derived growth factor BB (PDGF-BB). The LOD could be as low as 50 amol/L, which was much lower than the detection limit of PDGF-BB in 50% human serum (100 fmol/L) [Citation79].
Yıldırım et al. [Citation82] prepared the monodisperse-porous CeO2 NPs with peroxidase-like activity by the staged sol-gel templating protocol. The resulting product could be used as the adsorbent in metal oxide affinity chromatography (MOAC), which could achieve the separation and enrichment of phosphoprotein in carious samples. Moreover, the CeO2 NPs with peroxidase-like activity could be used to detect the concentration of phosphoprotein.
Based on the intrinsic oxidase-like activity of MnO2 nanosheets, a “lab in a tube” platform was developed for the determination of oxalate (C2O42-) in samples [Citation84]. The chromogen substrate TMB (3,3′,5,5′-tetramethylbenzidine) could be oxidized to oxTMB (blue) by MnO2. In the presence of oxalate, the MnO2 nanosheets would be decomposed to Mn2+ and the color reaction would be suppressed (). The color difference of the solutions could be detected and used for the quantitative determination of oxalate concentration. In order to ensure the stability of MnO2 in complex samples, sodium alginate (SA) hydrogel was used to load MnO2 nanosheets. The porous structure of the SA hydrogel could resist the interference of complex environments to a certain extent, and at the same time would not impede the mass transfer of substrates, which was conducive to the long-term storage of samples.
Figure 13. Schematic illustration for the detection of oxalate. The chromogen reagent TMB will not be oxidized to oxTMB with Mn2+, thus resulting in the color difference.
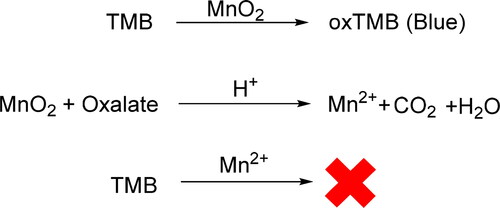
The rapid detection process was completed in a micro-tube and the kit could be stored for seven days with almost no change. There was a good linear relationship between the blue light intensity and the concentration of oxalate in the range of 0.8–800 μmol/L. Furthermore, as detected by the results of standard addition method, the recovery rates of the urine samples were in the range of 98.69–103.60% with the RSDs from 4.16% to 8.54%, indicating this kit was able to analyze the concentration of oxalate in human urine quantitatively.
Metallic oxide nanozymes possessing superoxide dismutase (SOD)-like activity are used for promoting the cleavage of ROS. Moderate ROS concentration is necessary for normal cells to maintain intracellular functions such as the defense against bacteria and viruses. However, it may lead to cell damage if the ROS concentration is too high. One of the main pathological mechanisms of Parkinson’s disease (PD) is mitochondrial dysfunction mediated by ROS [Citation76]. Hao et al. [Citation76] prepared novel chiral molecule-mediated porous CuxO nanoparticle clusters (CuxO NCs) with four kinds of enzyme-like activities (SOD, peroxidase, catalase and glutathione peroxidase). L-phenylalanine (L-Phe) was selected as the structure guiding agent, and other chiral amino acids, L-tyrosine (L-Tyr) and L-aspartic acid (L-asp) could not form the uniform structure of NCs. The cell experiments have shown that porous CuxO NCs could alleviate the cell death caused by neurotoxin 1-methyl-4-phenylpyridinium (MPP+), suggesting that they had the ability to scavenge ROS in cells. At the same time, they showed an excellent ability of anti-oxidative stress in the PD mouse models and successfully realized cognitive recovery in mice. CuxO NCs had good stability and low toxicity in vivo, which was beneficial to the treatment of organisms.
Carbon based
Carbon based nanomaterials have always been the focus of attention because of their extensive chemical inertness, good biocompatibility and unique high hardness.
Fan et al. [Citation93] developed the N-doped porous carbon nanospheres(N-PCNSs) with four enzyme-like activities (peroxidase, catalase, oxidase and SOD). With the help of ferritin, N-PCNSs were specific for tumor cells and could produce ROS in tumor cells, leading to the death of tumor cells. Ibarbia et al. [Citation94] developed a 3-(triethoxysilyl)propyl methacrylate (MPS) modified graphene oxide quantum dot (GOQD-MPS) with significantly enhanced peroxidase-like activity. Irradiation with visible light, GOQD-MPS could degrade Rhodamine B (RHB) effectively, indicating the GOQD-MPS was suitable for the treatment of organic dye wastewater.
Fang et al. [Citation87] developed oxygenated nanodiamonds (ONDs) with peroxidase-like activity against periodontal bacterial infection. Previous studies have confirmed that the carbonyl groups and carboxyl groups on the surface of a nanozyme with peroxidase-like activity were the catalytically active sites and the substrate binding sites, while the hydroxyl groups would inhibit the catalytic activity [Citation105, Citation106]. Therefore, the researchers treated the ordinary nano-diamonds (NDs) in the mixed acid composed of nitric acid and sulfuric acid for different time (12 h and 24 h). Consequently, the hydroxyl groups on the surface of NDs would be oxidized to carbonyl and carboxyl groups; the ONDs with enhanced peroxidase activity were finally synthesized.
Antibacterial agents, such as β-lactams, have lots of restrictions, since they are allergenic in the process of use and lead to multiple drug resistance. Another antimicrobial agent, H2O2, would not cause effective damage to bacteria at low concentrations (0.33 mol/L − 1 mol/L), while may have adverse effects on healthy tissues at high concentrations [Citation87]. The ONDs could generate abundant ROS (·OH) at low concentration of H2O2 to kill bacteria and avoid the potential damage of high H2O2 concentrations to the human body (genetic damage, oxidative damage). The experimental results showed that ONDs could effectively kill gram-negative and gram-positive bacteria under physiological conditions. The longer the treatment time of mixed acids, the better antibacterial performance produced. Compared with traditional antimicrobial agents, the nanozyme antibiotic hardly generated multi-drug resistance, which provided a new strategy to against bacterial infections.
Ren et al. [Citation29] synthesized a kind of porous carbon with Enteromorpha prolifera (EPC) that has oxidase and peroxidase-like activities. E. prolifera is a renewable marine waste biomass. EPC exhibited oxidase-like activity in the presence of oxygen, while showing peroxidase-like properties in the presence of H2O2 and without oxygen.
Based on the oxidase-like activity of EPC, an acid phosphatase colorimetric sensor was developed with a linear range of 0.5–15 U/L and a LOD of 0.1 U/L. Based on the peroxidase-like activity of EPC, a H2O2 fluorescent sensor was developed with a linear range of 0.05–80 μmol/L and a LOD of 0.017 μmol/L. Compared with H2O2 bio-electrochemical sensor with HRP [Citation31], EPC has more excellent performance in both detection range and LOD. Furthermore, EPC could be used to detect the glucose by combining with GOx, having a linear range of 0.05–10 mmol/L and a LOD of 30 μmol/L. The use of marine waste biomass as the raw material could reduce waste and costs, and the generated EPC has excellent sensing performance.
Others
In addition to the above nanozymes, there are other nanomaterials possessing enzyme-like activities, such as MOFs, Prussian blue (PB) NPs and so on.
Li et al. [Citation98] synthesized MOF-818 nanozyme with trinuclear copper centers by mimicking the binuclear copper centers structure of natural catechol oxidase. MOF-818 has catechol oxidase-like activity instead of peroxidase-like activity, so it could be used to oxidize O-diphenol to the corresponding O-quinone in the presence of oxygen ().
Son et al. [Citation100] synthesized a novel 3-aminophenylboronic acid (APBA) modified Prussian blue NPs (PBBA), which were applied to detect glycated albumin (GA) through colorimetric and electrochemical methods without affecting the peroxidase-like activity. PBBA exhibited a superior peroxidase-like activity with low detection limits (7.32 μg/mL and 3.47 μg/mL for colorimetric and electrochemical immunoassay, respectively), which could be used as a substitute for natural enzymes in the quantitative analysis of GA.
Conclusions and outlook
The advantage of enzyme catalysis lies in its high catalytic activity and greener reaction process, while the disadvantage lies in the lack of stability and difficult recycling. In fact, the immobilization of enzymes could overcome the above shortcomings. The immobilization carrier and immobilization methods are two most important factors by interfering the immobilization process. Nanomaterials are used to immobilize enzymes requiring the following criteria: mild synthesis process, high enzyme load, stability under extreme conditions (pH or temperature), large surface area and high mass transfer efficiency, which could improve the catalytic efficiency and increase the recycle times of immobilized enzymes.
As natural enzyme mimics, nanozyme undoubtedly occupies an advantage in terms of stability and reusability. However, current research on nanozymes is mainly concentrated on exploring the enzyme-like activity of some existing materials and less on the mechanism of the catalytic process. In addition, scientists recognize that nanozymes could have potential biotoxicity. Although nanostructured biocatalysts have been studied extensively, few products have been applied to large-scale industrial production actually. Recently, the application fields of nanozymes are still relatively few they mainly include sensors and biomedicine. Continuous efforts about nanozyme could be made in the following aspects: (1) profound study on the nanozyme catalytic mechanism to enable the design and synthesis of nanomaterials with specific enzyme activities; (2) development toward a nanozyme switch which could selectively turn on/off specific catalytic activity; (3) control over the substrate specificity of nanozymes through surface modification or the design of their materials; (4) expanded scope on the enzymatic activities and the application fields.
In this review, the development of nanomaterial-enzyme complexes and nanozyme were reviewed, and their advantages and disadvantages were compared. However, the two forms of nanostructured biocatalysts are not antagonistic. As we mentioned in the section about metal–organic frameworks (MOFs), a cascade reaction could be performed simultaneously by combining nanomaterial-enzyme complexes and nanozyme. It is a promising strategy to carry out joint research in the two fields. In addition, it is necessary to develop unified standards on the catalytic capacity and biological toxicity of nanostructured biocatalysts. With the development of nanotechnology and novel enzymes, we believe that great opportunities will be provided by nanostructured biocatalysis in the fields of biopharmaceutical, chemical engineering and environmental protection.
Acknowledgments
We are grateful for the National Natural Science Foundation of China (Project 21808205), Zhejiang Provincial Science and Technology Plan Project-Academician Special Project (No. 2019-ZJ-JS-03) and the Zhejiang Provincial Key R&D Project (No. 2020C03006)) for financial aids to this work.
Data availability statement
Data sharing is not applicable to this article as no new data were created or analyzed in this study.
Disclosure statement
The authors declare no conflict of interest.
References
- Albery WJ, Knowles JR. Efficiency and evolution of enzyme catalysis. Angew Chem Int Ed Engl. 1977;16(5):285–293.
- Grigoras AG. Catalase immobilization – a review. Biochem Eng J. 2017;117:1–20.
- Liu D-M, Dong C. Recent advances in nano-carrier immobilized enzymes and their applications. Process Bioch. 2020;92:464–475.
- Rasheed T, Nabeel F, Bilal M. Self-assembly of artificial peroxidase mimics from alternating copolymers with chromogenic and biocatalyst potentialities. J Ind Eng Chem. 2019;78:315–323.
- Bilal M, Rasheed T, Zhao Y, et al. “Smart" chemistry and its application in peroxidase immobilization using different support materials. Int J Biol Macromol. 2018;119:278–290.
- Romero-Fernandez M, Paradisi F. Protein immobilization technology for flow biocatalysis. Curr Opin Chem Biol. 2020;55:1–8.
- Hu Y, Dai L, Liu D, et al. Rationally designing hydrophobic UiO-66 support for the enhanced enzymatic performance of immobilized lipase. Green Chem. 2018;20(19):4500–4506.
- Ladole MR, Pokale PB, Patil SS, et al. Laccase immobilized peroxidase mimicking magnetic metal organic frameworks for industrial dye degradation. Bioresour Technol. 2020;317:124035.
- Zhu YT, Ren XY, Liu YM, et al. Covalent immobilization of porcine pancreatic lipase on carboxyl-activated magnetic nanoparticles: characterization and application for enzymatic inhibition assays. Mat Sci Eng C-Mater. 2014;38:278–285.
- Liu DM, Chen J, Shi YP. Tyrosinase immobilization on aminated magnetic nanoparticles by physical adsorption combined with covalent crosslinking with improved catalytic activity, reusability and storage stability. Anal Chim Acta. 2018;1006:90–98.
- Liu DM, Chen J, Shi Y-P. Advances on methods and easy separated support materials for enzymes immobilization. TrAC-Trend Anal Chem. 2018;102:332–342.
- Cipolatti EP, Valério A, Henriques RO, et al. Nanomaterials for biocatalyst immobilization – state of the art and future trends. RSC Adv. 2016;6(106):104675–104692.
- Ramakrishna TRB, Nalder TD, Yang W, et al. Controlling enzyme function through immobilisation on graphene, graphene derivatives and other two dimensional nanomaterials. J Mater Chem B. 2018;6(20):3200–3218.
- Hong T, Liu W, Li M, et al. Recent advances in the fabrication and application of nanomaterial-based enzymatic microsystems in chemical and biological sciences. Anal Chim Acta. 2019;1067:31–47.
- An J, Li G, Zhang Y, et al. Recent advances in enzyme-nanostructure biocatalysts with enhanced activity. Catalysts. 2020;10(3):338.
- Zhang K, Yang W, Liu Y, et al. Laccase immobilized on chitosan-coated Fe3O4 nanoparticles as reusable biocatalyst for degradation of chlorophenol. J Mol Struct. 2020;1220:128769.
- Hong T, Chi C, Ji Y. Pepsin-modified chiral monolithic column for affinity capillary electrochromatography. J Sep Sci. 2014;37(22):3377–3383.
- Xu S, Mo R, Jin C, et al. Mesoporous silica nanoparticles incorporated hybrid monolithic stationary phase immobilized with pepsin for enantioseparation by capillary electrochromatography. J Pharmaceut Biomed. 2017;140:190–198.
- Ortega-Liebana MC, Bonet-Aleta J, Hueso JL, et al. Gold-based nanoparticles on amino-functionalized meso-porous silica supports as nanozymes for glucose oxidation. Catalysts. 2020;10(3):333.
- Rafiei S, Tangestaninejad S, Horcajada P, et al. Efficient biodiesel production using a lipase@ZIF-67 nanobioreactor. Chem Eng J. 2018;334:1233–1241.
- Huang Y, Ren J, Qu X. Nanozymes: classification, catalytic mechanisms, activity regulation, and applications. Chem Rev. 2019;119(6):4357–4412.
- Wei H, Wang E. Nanomaterials with enzyme-like characteristics (nanozymes): next-generation artificial enzymes. Chem Soc Rev. 2013;42(14):6060–6093.
- Wu J, Wang X, Wang Q, et al. Nanomaterials with enzyme-like characteristics (nanozymes): next-generation artificial enzymes (II). Chem Soc Rev. 2019;48(4):1004–1076.
- Manea F, Houillon FB, Pasquato L, et al. Nanozymes: gold-nanoparticle-based transphosphorylation catalysts. Angew Chem Int Ed Engl. 2004;43(45):6165–6169.
- Gao L, Zhuang J, Nie L, et al. Intrinsic peroxidase-like activity of ferromagnetic nanoparticles. Nat Nanotechnol. 2007;2(9):577–583.
- Liang M, Yan X. Nanozymes: from new concepts, mechanisms, and standards to applications. Acc Chem Res. 2019;52(8):2190–2200.
- Wang Y, He C, Li W, et al. Catalytic performance of oligonucleotide-templated Pt nanozyme evaluated by laccase substrates. Catal Lett. 2017;147(8):2144–2152.
- Chen M, Wang Z, Shu J, et al. Mimicking a natural enzyme system: cytochrome c oxidase-like activity of Cu2O nanoparticles by receiving electrons from cytochrome c. Inorg Chem. 2017;56(16):9400–9403.
- Ren H, Liu X, Yan L, et al. Ocean green tide derived hierarchical porous carbon with bi-enzyme mimic activities and their application for sensitive colorimetric and fluorescent biosensing. Sensors Actuat B-Chem. 2020;312:127979.
- Ma L, Jiang F, Fan X, et al. Metal-organic-framework-engineered enzyme-mimetic catalysts. Adv Mater. 2020;32(49):2003065.
- Sardaremelli S, Hasanzadeh M, Seidi F. Enzymatic recognition of hydrogen peroxide (H2O2) in human plasma samples using HRP immobilized on the surface of poly(arginine-toluidine blue)-modified Fe3O4 nanoparticles modified polydopamine; a novel biosensor. J Mol Recognit. 2021;34(11):e2928.
- Cui J, Ren S, Lin T, et al. Shielding effects of Fe3+-tannic acid nanocoatings for immobilized enzyme on magnetic Fe3O4@silica core shell nanosphere. Chem Eng J. 2018;343:629–637.
- Suo H, Xu L, Xue Y, et al. Ionic liquids-modified cellulose coated magnetic nanoparticles for enzyme immobilization: improvement of catalytic performance. Carbohyd Polym. 2020;234:115914.
- Liu J, Zhao W, Zhang L, et al. Synthesis of substituted 2H-chromenes catalyzed by lipase immobilized on magnetic multiwalled carbon nanotubes. Biotechnol Appl Bioc. 2021;68(2):411–416.
- Shi J, Zhang S, Deng Q, et al. A versatile biocatalytic nano-platform based on Fe3O4-filled and zirconia shrunk holey carbon nanotubes. Chem Eng J. 2020;402:125737.
- Saikia K, Vishnu D, Rathankumar AK, et al. Development of a magnetically separable co-immobilized laccase and versatile peroxidase system for the conversion of lignocellulosic biomass to vanillin. J. Air Waste Manage. 2020;70(12):1252–1259.
- Zhang Y, Li X, Li D, et al. A laccase based biosensor on Au NPs-MoS2 modified glassy carbon electrode for catechol detection. Colloid Surface B. 2020;186:110683.
- Luo L, Zhou X, Pan Y, et al. A simple and sensitive flow injection chemiluminescence immunoassay for chloramphenicol based on gold nanoparticle-loaded enzyme. Luminescence. 2020;35(6):877–884.
- Chronopoulou L, Scaramuzzo FA, Fioravanti R, et al. Noble metal nanoparticle-based networks as a new platform for lipase immobilization. Int J Biol Macromol. 2020;146:790–797.
- Wang H, Li S, Li J, et al. Immobilized polyphenol oxidase: preparation, optimization and oxidation of phenolic compounds. Int J Biol Macromol. 2020;160:233–244.
- Bounegru AV, Apetrei C. Development of a novel electrochemical biosensor based on carbon nanofibers-gold nanoparticles-tyrosinase for the detection of ferulic acid in cosmetics. Sensors-Basel. 2020;20(23):6724.
- Karimi Alavijeh M, Meyer AS, Gras SL, et al. Improving β-galactosidase-catalyzed transglycosylation yields by cross-linked layer-by-layer enzyme immobilization. ACS Sustain Chem Eng. 2020;8(43):16205–16216.
- Elias N, Wahab RA, Chandren S, et al. Structure and properties of lipase activated by cellulose-silica polyethersulfone membrane for production of pentyl valerate. Carbohyd Polym. 2020;245:116549.
- Jia B, Liu C, Qi X. Selective production of ethyl levulinate from levulinic acid by lipase-immobilized mesoporous silica nanoflowers composite. Fuel Process Technol. 2020;210:106578.
- Wu JQ, Xu XM, Wang DL, et al. Immobilization of phospholipase D on macroporous SiO2/cationic polymer nano-composited support for the highly efficient synthesis of phosphatidylserine. Enzyme Microb Tech. 2020;142:109696.
- Ariaeenejad S, Jokar F, Hadian P, et al. An efficient nano-biocatalyst for lignocellulosic biomass hydrolysis: xylanase immobilization on organically modified biogenic mesoporous silica nanoparticles. Int J Biol Macromol. 2020;164:3462–3473.
- Chen J, Sun B, Sun C, et al. Immobilization of lipase AYS on UiO-66-NH2 met-al-organic framework nanoparticles as a recyclable biocatalyst for ester hydrolysis and kinetic resolution. Sep Purif Technol. 2020;251:117398.
- Zhu F, Xu W, Li X, et al. Lipase immobilization on UiO-66/poly(vinylidene fluoride) hybrid membranes and active catalysis in the vegetable oil hydrolysis. New J Chem. 2020;44(34):14379–14388.
- Yuan X, Liu Y, Cao F, et al. Immobilization of lipase onto metal–organic frameworks for enantioselective hydrolysis and transesterification. AIChE J. 2020;66(9):e16292.
- Guo M, Chi J, Zhang C, et al. A simple and sensitive sensor for lactose based on Cascade reactions in au nanoclusters and enzymes co-encapsulated metal-organic frameworks. Food Chem. 2021;339:127863.
- Li X, Feng Q, Lu K, et al. Encapsulating enzyme into metal-organic framework during in-situ growth on cellulose acetate nanofibers as self-powered glucose biosensor. Biosens Bioelectron. 2021;171:112690.
- Li S-F, Zhai X-J, Zhang C, et al. Enzyme immobilization in highly ordered macro–microporous metal–organic frameworks for rapid biodegradation of hazardous dyes. Inorg Chem Front. 2020;7(17):3146–3153.
- Farmakes J, Schuster I, Overby A, et al. Enzyme immobilization on graphite oxide (GO) surface via one-pot synthesis of GO/metal-organic framework composites for large-substrate biocatalysis. ACS Appl Mater Interfaces. 2020;12(20):23119–23126.
- Huang S, Chen G, Ye N, et al. Iron-mineralization-induced mesoporous metal-organic frameworks enable high-efficiency synergistic catalysis of natural/nanomimic enzymes. ACS Appl Mater Interfaces. 2020;12(51):57343–57351.
- Zhu L, Shen B, Song Z, et al. Permeabilized TreS-expressing Bacillus subtilis cells decorated with glucose isomerase and a shell of ZIF-8 as a reusable biocatalyst for the coproduction of trehalose and fructose. J Agric Food Chem. 2020;68(15):4464–4472.
- Xu J, Sun J, Wang Y, et al. Application of iron magnetic nanoparticles in protein immobilization. Molecules. 2014;19(8):11465–11486.
- Kiran; Rathour RK, Bhatia RK, Rana DS, et al. Fabrication of thermostable and reusable nanobiocatalyst for dye decolourization by immobilization of lignin peroxidase on graphene oxide functionalized MnFe2O4 superpara-magnetic nanoparticles. Bioresour Technol. 2020;317:124020.
- Darwesh OM, Ali SS, Matter IA, et al. Enzymes immobilization onto magnetic nanoparticles to improve industrial and environmental applications. Methods Enzymol. 2020;630:481–502.
- Zhou Z, Hartmann M. Progress in enzyme immobilization in ordered mesoporous materials and related applications. Chem Soc Rev. 2013;42(9):3894–3912.
- Jo SM, Jiang S, Graf R, et al. Aqueous core and hollow silica nanocapsules for confined enzyme modules. Nanoscale. 2020;12(47):24266–24272.
- Mukundan S, Melo JS, Sen D, et al. Enhancement in β-galactosidase activity of Streptococcus lactis cells by entrapping in microcapsules comprising of correlated silica nanoparticles. Colloids Surf B Biointerfaces. 2020;195:111245.
- Huang S, Kou X, Shen J, et al. “Armor-plating" enzymes with metal-organic frameworks (MOFs). Angew Chem Int Ed. 2020;59(23):8786–8798.
- Liang J, Gao S, Liu J, et al. Hierarchically porous biocatalytic MOF microreactor as a versatile platform towards enhanced multienzyme and cofactor-dependent biocatalysis. Angew Chem Int Ed Engl. 2021;60(10):5421–5428.
- Chong Y, Huang J, Xu X, et al. Hyaluronic acid-modified Au-Ag alloy nanoparticles for radiation/nanozyme/Ag+ multimodal synergistically enhanced cancer therapy. Bioconjugate Chem. 2020;31(7):1756–1765.
- Yang R, Fu S, Li R, et al. Facile engineering of silk fibroin capped AuPt bimetallic nanozyme responsive to tumor microenvironmental factors for enhanced nanocatalytic therapy. Theranostics. 2021;11(1):107–116.
- Xu Z, Sun P, Zhang J, et al. High-efficiency platinum–carbon nanozyme for photodynamic and catalytic synergistic tumor therapy. Chem Eng J. 2020;399:125797.
- Wu L, Zhou M, Wang Y, et al. Nanozyme and aptamer-based immunosorbent assay for aflatoxin B1. J Hazard Mater. 2020;399:123154.
- Gupta PK, Son SE, Seong GH. One-pot synthesized citric acid-modified bimetallic PtNi hollow nanospheres as peroxidase mimics for colorimetric detection of human serum albumin. Mat Sci Eng C-Mater. 2020;116:111231.
- Gökçal B, Kip Ç, Tuncel A. One-pot, direct glucose detection in human whole blood without using a dilution factor by a magnetic nanozyme with dual enzymatic activity. J Alloy Compd. 2020;843:156012.
- Long L, Liu J, Lu K, et al. Highly sensitive and robust peroxidase-like activity of Au-Pt core/shell nanorod-antigen conjugates for measles virus diagnosis. J Nanobiotechnol. 2018;16:46.
- Wu J, Qin K, Yuan D, et al. Rational design of Au@Pt multibranched nanostructures as bi-functional nanozymes. ACS Appl Mater Interfaces. 2018;10(15):12954–12959.
- Tian Y, Chen Y, Chen M, et al. Peroxidase-like Au@Pt nanozyme as an integrated nanosensor for Ag+ detection by LSPR spectroscopy. Talanta. 2021;221:121627.
- Du C, Gao Y, Chen H, et al. A Cu and Fe dual-atom nanozyme mimicking cytochrome c oxidase to boost the oxygen reduction reaction. J Mater Chem A. 2020;8(33):16994–17001.
- Mohammad M, Ahmadpoor F, Shojaosadati SA. Mussel-inspired magnetic nanoflowers as an effective nanozyme and antimicrobial agent for biosensing and catalytic reduction of organic dyes. ACS Omega. 2020;5(30):18766–18777.
- Gallay P, Eguilaz M, Rivas G. Designing electrochemical interfaces based on nanohybrids of avidin functionalized-carbon nanotubes and ruthenium nanoparticles as peroxidase-like nanozyme with supramolecular recognition properties for site-specific anchoring of biotinylated residues. Biosens Bioelectron. 2020;148:111764.
- Hao C, Qu A, Xu L, et al. Chiral molecule-mediated porous CuxO nanoparticle clusters with antioxidation activity for ameliorating parkinson’s disease. J Am Chem Soc. 2019;141(2):1091–1099.
- Wang F, Zhang Y, Liu Z, et al. A mesoporous encapsulated nanozyme for decontaminating two kinds of wastewater and avoiding secondary pollution. Nanoscale. 2020;12(27):14465–14471.
- Zhou Y, Wei W, Cui F, et al. Construction of a chiral artificial enzyme used for enantioselective catalysis in live cells. Chem Sci. 2020;11(41):11, 11344–11350.
- Zhang R, Lu N, Zhang J, et al. Ultrasensitive aptamer-based protein assays based on one-dimensional core-shell nanozymes. Biosens Bioelectron. 2020;150:111881.
- Gong F, Yang N, Wang Y, et al. Oxygen-deficient bimetallic oxide FeWOx nanosheets as peroxidase-like nanozyme for sensing cancer via photoacoustic imaging. Small. 2020;16(46):2003496.
- Tian Z, Liu H, Guo Z, et al. A pH-responsive polymer-CeO2 hybrid to catalytically generate oxidative stress for tumor therapy. Small. 2020;16(47):2004654.
- Yıldırım D, Gökçal B, Büber E, et al. A new nanozyme with peroxidase-like activity for simultaneous phosphoprotein isolation and detection based on metal oxide affinity chromatography: monodisperse-porous cerium oxide microspheres. Chem Eng J. 2021;403:126357.
- Tian L, Zhang Y, Wang L, et al. Ratiometric dual signal-enhancing-based electrochemical biosensor for ultrasensitive kanamycin detection. ACS Appl Mater Interfaces. 2020;12(47):52713–52720.
- Jin R, Zhao L, Yan X, et al. Lab in hydrogel portable kit: on-site monitoring of oxalate. Biosens Bioelectron. 2020;167:112457.
- Liu J, Gao J, Zhang A, et al . Carbon nanocage-based nanozyme as an endogenous H2O2-activated oxygenerator for real-time bimodal imaging and enhanced phototherapy of esophageal cancer. Nanoscale. 2020;12(42):21674–21686.
- Zhu X, Liu Y, Yuan G, et al. In situ fabrication of MS@MnO2 hybrid as nanozymes for enhancing ROS-mediated breast cancer therapy. Nanoscale. 2020;12(43):22317–22329.
- Fang J, Wang H, Bao X, et al. Nanodiamond as efficient peroxidase mimic against periodontal bacterial infection. Carbon. 2020;169:370–381.
- Heo NS, Song HP, Lee SM, et al. Rosette-shaped graphitic carbon nitride acts as a peroxidase mimic in a wide pH range for fluorescence-based determination of glucose with glucose oxidase. Microchim Acta. 2020;187:286.
- Zhang P, Sun D, Cho A, et al. Modified carbon nitride nanozyme as bifunctional glucose oxidase-peroxidase for metal-free bioinspired Cascade photocatalysis. Nat Commun. 2019;10(1):940.
- Zeng G, Duan M, Xu Y, et al. Platinum (II)-doped graphitic carbon nitride with enhanced peroxidase-like activity for detection of glucose and H2O2. Spectrochim Acta A Mol Biomol Spectrosc. 2020;241:118649.
- Zhao X, Li S, Yu X, et al . In situ growth of CeO2 on g-C3N4 nanosheets toward a spherical g-C3N4/CeO2 nanozyme with enhanced peroxidase-like catalysis: a selective colorimetric analysis strategy for mercury(II). Nanoscale. 2020;12(41):21440–21446.
- Wang L, Gao F, Wang A, et al. Defect-rich adhesive molybdenum disulfide/rGo vertical heterostructures with enhanced nanozyme activity for smart bacterial killing application. Adv Mater. 2020;32(48):2005423.
- Fan K, Xi J, Fan L, et al. In vivo guiding nitrogen-doped carbon nanozyme for tumor catalytic therapy. Nat Commun. 2018;9(1):1440.
- Ibarbia A, Sánchez-Abella L, Lezama L, et al. Graphene quantum dot-based hydrogels for photocatalytic degradation of organic dyes. Appl Surf Sci. 2020;527:146937.
- Liu X, Liu Z, Dong K, et al. Tumor-activatable ultrasmall nanozyme generator for enhanced penetration and deep catalytic therapy. Biomaterials. 2020;258:120263.
- Hormozi Jangi SR, Akhond M. Synthesis and characterization of a novel metal-organic framework called nanosized electroactive quasi-coral-340 (NEQC-340) and its application for constructing a reusable nanozyme-based sensor for selective and sensitive glutathione quantification. Microchem J. 2020;158:105328.
- Xu Z, Long LL, Chen YQ, et al. A nanozyme-linked immunosorbent assay based on metal-organic frameworks (MOFs) for sensitive detection of aflatoxin B1. Food Chem. 2021;338:128039.
- Li M, Chen J, Wu W, et al. Oxidase-like MOF-818 nanozyme with high specificity for catalysis of catechol oxidation. J Am Chem Soc. 2020;142(36):15569–15574.
- Li X, Li X, Li D, et al. Electrochemical biosensor for ultrasensitive exosomal miRNA analysis by Cascade primer exchange reaction and MOF@Pt@MOF nanozyme. Biosens Bioelectron. 2020;168:112554.
- Son SE, Gupta PK, Hur W, et al. Determination of glycated albumin using a Prussian blue nanozyme-based boronate affinity sandwich assay. Anal Chim Acta. 2020;1134:41–49.
- Zhang R, Zhou Y, Yan X, et al. Advances in chiral nanozymes: a review. Microchim Acta. 2019;186:782.
- Tao X, Wang X, Liu B, et al. Conjugation of antibodies and aptamers on nanozymes for developing biosensors. Biosens Bioelectron. 2020;168:112537.
- Meng Y, Li W, Pan X, et al. Applications of nanozymes in the environment. Environ Sci Nano. 2020;7(5):1305–1318.
- Bian H, Nguyen NT, Yoo J, et al. Forming a highly active, homogeneously alloyed AuPt co-catalyst decoration on TiO2 nanotubes directly during anodic growth. ACS Appl Mater Interfaces. 2018;10(21):18220–18226.
- Sun H, Zhao A, Gao N, et al. Deciphering a nanocarbon-based artificial peroxidase: chemical identification of the catalytically active and substrate-binding sites on graphene quantum dots. Angew Chem Int Ed. 2015;54(24):7176–7180.
- Wang H, Li P, Yu D, et al. Unraveling the enzymatic activity of oxygenated carbon nanotubes and their application in the treatment of bacterial infections. Nano Lett. 2018;18(6):3344–3351.