Abstract
Age-related macular degeneration, a type of retinal degenerative disease, is the primary cause of severe visual impairment and irreversible blindness in people older than 50, due to age-related deterioration of the photoreceptors and the retinal pigment epithelium (RPE), which could be restored by cell therapy. However, the success of cell therapy depends on biocompatible scaffolds, which serve as carriers for the growth of RPE cells for repairing damaged or diseased retinas. In this study, the plasmid pET-SUMO-R2C for expressing a mini-spidroin, fusing the second repetitive region to the C-terminal domain of the minor ampullate spidroin gene from the spider Araneus ventricosus, was constructed and named R2C. The spidroin R2C was expressed, purified and wet spun into silk-like fibers, possessing a Young’s modulus of 3.51 ± 0.92 GPa and toughness of 24.67 ± 9.51 MPa. The spidroin R2C, mixed with poly(L-lactide-co-ε-caprolactone) (PLCL) at a ratio of 3:1, was electrospun into nanofibrous scaffolds, composed of nanometric fibers with a diameter of 751.70 ± 19.48 nm. After co-culturing with the R2C/PLCL scaffolds, the transcriptomic differences of the ARPE-19 cells were analyzed, showing that fourteen genes that are mainly related to inflammation, tumors and keratinization were upregulated more than three times, while seven genes that are mostly related to substance transport and homeostasis were downregulated more than three times. The R2C/PLCL scaffolds have the potential to be further studied to serve as a suitable carrier for RPE transplantation in the future.
Introduction
Retinal degenerative diseases, including age-related macular degeneration (AMD), diabetic retinopathy and inherited retinal degenerations [Citation1], manifest as apoptosis or functional impairment of photoreceptors and retinal pigment epithelium (RPE), which are the inner and outer layers of the retina, respectively. Degenerative diseases of the retina seriously threaten public health [Citation2]. According to World Health Organization (WHO) data, 253 million individuals worldwide, of whom 81% were aged 50 years or older, suffered from visual impairment in 2017, including 217 million with moderate to severe impairment and 36 million completely blind [Citation3]. AMD, which is thought to be the primary cause of severe visual impairment and irreversible blindness in individuals older than 50 years, is forecast to affect about 288 million people globally by 2040 [Citation4]. AMD can be classified into neovascular (wet) AMD and non-neovascular (dry) AMD [Citation5]. Several therapies, such as treatment with anti-vascular endothelial growth factor (VEGF) agents and photodynamic therapy, have been proven to be effective for wet AMD, while the disease progression is only delayed [Citation6,Citation7]. In addition, there are currently no effective treatments for dry AMD, the most prevalent form of AMD. Due to the cell loss or dysfunction of RPE cells and photoreceptors in dry AMD [Citation8], a more promising opportunity has been confirmed, namely tissue engineering. RPE cells are pre-cultured on a scaffold to support maturation into a functional monolayer, and then the cell/scaffold complexes are implanted beneath the retina [Citation9]. The success of tissue engineering depends on the development of ideal scaffolds, which should be thin, porous, biodegradable and biocompatible, and should possess good mechanical properties [Citation10]. These scaffolds function as Bruch’s membrane, offering a laminar tissue and structural guide route for the transplants as well as a more structured way to distribute these cells to the subretinal areas and increase cell survival and differentiation in multiple retinal degeneration models [Citation11,Citation12].
Numerous natural polymers, such as collagens [Citation13], gelatins [Citation14] and fibrin [Citation15], have been used to construct the biomimetic scaffolds, similar to Bruch’s membrane, to support the attachment and growth of a monolayer of functional RPE cells and continue to support the cells post-implantation in the long term [Citation16]. Due to their similarity to the extracellular matrix (ECM), natural polymers combine several benefits with the capacity to control cell behaviors that closely resemble the ECM of the desired tissue type. However, it is challenging to manage the mechanical characteristics of the resulting scaffolds and the homogeneity of a naturally formed product [Citation9]. Due to their tailorability and consistency, synthetic materials, such as poly(lactic-co-glycolic acid) (PLGA) [Citation17], poly(L-lactide) (PLA) [Citation18], poly(glycerol sebacate) (PGS) [Citation19], poly(ε-caprolactone) (PCL) [Citation20] and expanded polytetrafluoroethylene (ePTFE) [Citation16], have been used to fabricate artificial scaffolds. However, the surfaces of the synthetic scaffolds are too hydrophobic to supply an accurate in vivo microenvironment for cells [Citation21]. Hybrid scaffolds, such as collagen I/PLGA [Citation22] and laminin/PCL [Citation23], have the potential to integrate the characteristics of both natural and synthetic materials, resulting in a scaffold with all the benefits of a natural scaffold in terms of protein composition and native look and the customizability of synthetic scaffolds [Citation24].
Owing to their advantages of biocompatibility, biodegradability and non-toxic breakdown products [Citation25,Citation26], both PLA [Citation27] and PCL [Citation28] have been widely used in biomedical research. Nevertheless, PLA demonstrates strong strength and poor toughness, whereas PCL exhibits the opposite [Citation29]. The poly(L-lactide-co-ε-caprolactone) (PLCL), with improved strength and toughness, was copolymerized by lactide and ε-caprolactone at different ratios to combine both the advantages of PLA and PCL [Citation29,Citation30]. However, PLCL should always be blended with other natural polymers, such as silk fibroin, to fabricate hybrid scaffolds to overcome their drawbacks of being bioinert and lacking cell-binding and recognition sites [Citation31,Citation32]. The surfaces of the electrospun fibroin/PLCL scaffolds were smooth and uniform, with appropriate mechanical properties as skeleton materials [Citation31]. Moreover, these scaffolds can not only markedly promote the proliferation and growth of the retinal progenitor cells (RPC), but also strongly enhance the differentiation of RPCs into specific retinal neurons in vitro [Citation32].
Spider silk, as the natural polymer of spider silk protein (spidroin), possesses extraordinary mechanical properties, such as elasticity and toughness [Citation33], as well as good biocompatibility, biodegradability, and low immunogenicity [Citation34]. Therefore, the spidroins have been widely used as attractive biomaterials, with improved efficacy and minor side effects, in many biomedical applications [Citation35]. Numerous studies on the combination of spidroins with other polymers to form modified membranes have been conducted [Citation36]. Altering the morphology of the synthetic materials by mixing spidroins can promote cell attachment [Citation37]. By using the electrospinning technique, spidroins could be fabricated into nonwoven membranes or meshes with nanometer-scale pore size and structure, exhibiting biocompatibility with biological tissues and even resembling histology structures [Citation34].
In this study, the second repetitive region (Rep2) and the C-terminal domain (CT) of the minor ampullate spidroins (MiSp) gene from the spider Araneus ventricosus were fused together to construct a mini-spidroin (R2C), which was then expressed in Escherichia coli and purified by using a Ni-NTA affinity column. The spidroin R2C was wet spun into silk-like fibers by using the wetspinning technique. The mechanical properties of the wet-spun fibers were tested. The spidroin R2C, which was combined with PLCL at a ratio of 3:1, was electrospun to form thin nanofibrous scaffolds. The transcriptomic effects of the R2C/PLCL scaffolds on the immortalized human adult retinal pigment epithelial cell line (ARPE-19) cells were then investigated.
Materials and methods
Construction of expression plasmid of spidroin R2C
As shown in the schematic illustration in , the fusion protein 6 × His-SUMO-R2C contained three sequential constructs, the 6 × His-SUMO tag, the Rep2 domain of MiSp from the spider A. ventricosus (GeneBank accession number AFV31615.1) and the CT domain of MiSp from the spider A. ventricosus (GeneBank accession number AFV31615.1). According to the protocol of the OEPR cloning [Citation38], the Rep2 and CT sequences (generously donated by Prof. Qing Meng from Donghua University) were sequentially inserted into the 3′ end of the gene sumo of the in-house plasmid pET-SUMO, which contained a 6 × His purification tag and a SUMO tag. The SUMO tag was used to enhance the heterologous expression solubility of spidroin R2C in E. coli BL21 (DE3) (G6030, AngYuBio, Shanghai, China). Firstly, the CT gene was ligated to the 3′ end of the sumo gene of the pET-SUMO plasmid to obtain the plasmid pET-SUMO-CT. The CT gene was amplified by using 1 U of KOD-FX DNA polymerase (KFX-101, TOYOBO, Osaka, Japan) supplemented with 0.2 mmol/L primers CT-F (5′-ATCGAAGCTCACCGTGAACAGATCGGTGGTGTAGCTGCATATGGTGGCGCAG-3′) and CT-R (5′-GTGGTGGTGGTGCTCGAGTCAACCTACATATTGGCCTACTGAATC-3′), 1 × PCR reaction buffer, 0.4 mmol/L dNTPs and 10 ng template DNA in 50 μL of the reaction mixture. The PCR program (BG-TC100 Thermal Cycler, Baygene, Beijing, China) included initial denaturation at 94 °C for 2 min; 25 cycles of denaturation at 98 °C for 10 s, annealing at 60 °C for 30 s and elongation at 68 °C for 30 s; and a final extension step at 68 °C for 1 min. The PCR products were purified using a DNA purification kit (DP214-02, TIANGEN, Beijing, China). The second PCR mixture contained 500 ng of the first PCR products, 0.2 mmol/L reverse primer (5′-ACCACCGATCTGTTCACGGTGAG-3′), 10 ng plasmid pET-SUMO, 1 × PCR reaction buffer, 0.4 mmol/L dNTPs and 1 U KOD-FX DNA polymerase in a 50 μL reaction volume. The program included initial denaturation at 94 °C for 2 min; 25 cycles of denaturation at 98 °C for 10 s, annealing at 60 °C for 30 s and elongation at 68 °C for 5 min; and final extension at 68 °C for 10 min. The second PCR products were purified using a PCR purification kit. Purified products (8 μL) were supplemented with 1 μL 10 × reaction buffer and digested using 1 μL DpnI (FD1704, Thermo Scientific, Waltham, USA) at 37 °C for 1 h. The digested products (5 μL) were transformed directly into 50 μL of chemically competent TOP10F’ cells (G6001, AngYuBio, Shanghai, China). The DNA sequence of the plasmid was verified by DNA sequencing, which was performed by Sangon (Shanghai, China), and then the plasmid was named pET-SUMO-CT. Then, the gene Rep2 was inserted into the 3′ end of gene sumo of the pET-SUMO-CT plasmid to obtain the plasmid pET-SUMO-R2C. The gene Rep2 was amplified by using 1 U of KOD-FX DNA polymerase supplemented with 0.2 mmol/L of primers Rep2-F (5′-ATCGAAGCTCACCGTGAACAGATCGGTGGTGGAGCTGGTGCAGGAGGTGC-3′) and Rep2-R (5′-CTGCGCCACCATATGCAGCTACAGCTCCTCCCGCACCTGCCG-3′), 1× PCR reaction buffer, 0.4 mmol/L dNTPs and 10 ng template DNA in 50 μL reaction volume. The PCR program included initial denaturation at 94 °C for 2 min, 25 cycles of denaturation at 98 °C for 10 s, annealing at 60 °C for 30 s and elongation at 68 °C for 1 min, and a final extension step at 68 °C for 2 min. The PCR products were purified using a PCR purification kit. The second PCR mixture contained 500 ng of the first PCR products, 0.2 mmol/L reverse primer (5′-ACCACCGATCTGTTCACGGTGAG-3′), 10 ng plasmid pET-SUMO-CT, 1 × PCR reaction buffer, 0.4 mmol/L dNTPs and 1 U KOD-FX DNA polymerase in a 50 μL reaction mixture. The PCR program included initial denaturation at 94 °C for 2 min; 25 cycles of denaturation at 98 °C for 10 s, annealing at 60 °C for 30 s and elongation at 68 °C for 5 min, followed by a final extension step at 68 °C for 10 min. The second PCR products were purified using a PCR purification kit. Purified products (8 μL) were supplemented with 1 μL 10 × reaction buffer and digested using 1 μL DpnI at 37 °C for 1 h. The digested products (5 μL) were transformed directly into 50 μL of chemically competent TOP10F’ cells. The DNA sequence of the plasmid was verified by DNA sequencing, which was performed by Sangon (Shanghai, China), and then the plasmid was named pET-SUMO-R2C.
Expression and purification of spidroin R2C
One tube of chemically competent BL21 (DE3) cells (100 μL) (G6030, AngYuBio, Shanghai, China) was taken from storage at −80 °C and placed on ice for 5 min. After thawing, 20 ng of the plasmid pET-SUMO-R2C was added to the competent BL21 (DE3) cells and mixed well. The tube was placed on ice for 30 min, subjected to heat shock at 42 °C for 45 s, and then placed on ice for 2 min. After the addition of 1 mL of SOC medium, the cells were recovered at 220 rpm and 37 °C for 45 min. Finally, 100 μL of cells were spread on an LB agar plate containing 100 μg/mL of ampicillin sodium and incubated at 37 °C overnight. A single colony was picked from the overnight cultured plate and inoculated into 10 mL LB medium containing 100 µg/mL ampicillin sodium and then cultured at 37 °C and 220 rpm for 4-6 h. When the optical density (OD600) value reached about 0.6-1.0, the cultured cells were inoculated at a volume ratio of 1:100 into 500 mL of LB medium supplemented with 100 µg/mL of ampicillin sodium, and were grown at 37 °C and 220 rpm for 4-6 h. When the OD600 value reached about 0.6-1.0, the induction was achieved by adding isopropyl beta-D-thiogalactopyranoside (IPTG, A600168, Sangon, Shanghai, China) to a final concentration of 0.5 mmol/L. The induction was performed at 16 °C and 220 rpm for 16 h.
After induction, the cells were collected by centrifuging at 2907 g for 20 min at room temperature, resuspended in 40 mL of 20 mmol/L Tris-HCl buffer (pH 8.0) supplemented with 0.5 mol/L NaCl, and then disrupted by an ultrasonic crusher (Lichen, Shanghai, China) for about 2-3 h under the condition of an ice bath. After the cell disruption process, the supernatant was obtained by centrifugation at 11627 g, 4 °C for 10 min. After that, 5 mol/L imidazole was added to the supernatant, bringing the final concentration to 10 mmol/L. After being passed through a 0.45-μm membrane filter, the supernatant was applied to a Ni-NTA column (Sangon, Shanghai, China). The target protein was eluted with 40 mL of elution buffer (20 mmol/L Tris-HCl, pH 8.0, 0.5 mol/L NaCl, and 200 mmol/L imidazole). Then, the eluates from the Ni-NTA column were desalted and concentrated by ultrafiltration at 5697 g and 4 °C, and then digested by using the SUMO protease (SAE0067-2500UN, Sigma-Aldrich, USA) overnight at 4 °C to remove the SUMO tag and release the target spidroin R2C. The digested solution was reloaded onto the Ni-NTA column to remove the SUMO tag. The flow-through solution was collected, desalted by using dialysis with a 12-kDa-cutoff dialysis membrane, and then lyophilized.
SDS-PAGE electrophoresis
After purification, the protein samples were analyzed via sodium dodecyl sulfate-polyacrylamide gel electrophoresis (SDS-PAGE) electrophoresis. In summary, 15 μL of each sample was added to 5 μL of 4 × SDS-PAGE loading solution and thoroughly mixed. After 10-min boiling, the mixtures were loaded into corresponding wells of a 12% acrylamide gel. After 120-min electrophoresis at 35 mA, the gel was fixed with a fixative solution containing 0.77% ammonium acetate, 10% acetate acid and 50% methanol, and subsequently stained with staining solution containing 10% acetate acid, 45% methanol and 0.025% Coomassie brilliant blue R250. Finally, the decolorizing step was carried out with decoloring solution containing 8% acetate acid and 25% methanol.
Preparation of wet-spun fibers of R2C
The purified spidroin R2C was dissolved in 1,1,1,3,3,3-Hexafluoro-2-propanol (HFIP, H811026, Macklin, Shanghai, China) at a final concentration of 10% (w/v). The solutions were thoroughly stirred overnight at room temperature and then centrifuged at 13680 g for 5 min to discard the insoluble impurities. The supernatant was placed in a 5 mL plastic syringe connected to a 30-gauge needle with an inner diameter of 0.16 mm. The wet-spun fibers were formed by withdrawing the wet-spinning solution into the coagulation bath (90% methanol) at a constant rate of 2 mL/h from the syringe by using a syringe pump (LongerPump, Hebei, China), and wound onto a drum.
Morphology of the wet-spun fibers of R2C
Dry fibers were positioned on the stub of the scanning electron microscope (SEM) (Phenom-World BV, The Netherlands), coated with gold for 45 s, followed by observation and photography at an acceleration voltage of 10 kV and room temperature.
Mechanical properties of the wet-spun fibers of R2C
The wet-spun fibers that were used for tensile tests were observed and photographed by an inverted phase-contrast microscope (DMi8, Leica, USA) at 400× magnification. Three different locations along the fiber in each photograph were used for calculating the average diameter of the wet-spun fibers by using ImageJ software.
The mechanical properties of the wet-spun fibers were tested using a Fiber Tensile Tester XQ-1AN (New Fiber Instrument, Shanghai, China) at room temperature and relative humidity of about 40%-60%. At a constant strain rate of 20 mm/min, the fibers were stretched until the fibers broke. The average mechanical properties of the wet-spun R2C fibers were calculated from 10 samples. The mechanical properties of the breaking strain and stress, Young’s modulus and toughness were calculated via the software of the Fiber Tensile Tester XQ-1AN.
Preparation of the electrospun R2C/PLCL scaffolds
The electrospinning method was schematically illustrated in . The spidroin R2C and PLCL (lactide: caprolactone, 70: 30) (P918694, Macklin, Shanghai, China), at a ratio of 3:1, were dissolved in HFIP at a final concentration of 7.5% and 2.5% (w/v), respectively (). After complete dissolution, the R2C and PLCL electrospinning solutions were mixed and stirred overnight at room temperature to make sure they were completely dissolved, followed by centrifugation at 13680 g for 5 min to discard any insoluble parts. For the fabrication of electrospun scaffolds, the electrospinning solution was placed in a 2.5-mL plastic syringe equipped with an extended tube and a 23-gauge needle with an inner diameter of 0.34 mm. As illustrated in , a positive high voltage of about 15 kV was applied by using a high voltage generator (Dongwen High Voltage, Tianjin, China) at about a 15-cm distance between the needle tip and an aluminum foil as a fiber collector. The electrospinning solution was withdrawn into the high-voltage electric field at a constant rate of 0.6 mL/h from the syringe by utilizing a syringe pump (LongerPump, Hebei, China). The electrospun R2C/PLCL scaffolds were dried in a fume hood for 24 h and then stripped from the aluminum foil.
Morphology of the R2C/PLCL scaffolds
After being coated with gold particles for 45 s, the microstructure of the electrospun R2C/PLCL scaffolds was observed and photographed by the SEM microscope at magnifications of ×2500 with an accelerating voltage of 10 kV. Fifty fibers on the SEM image were randomly selected to calculate the diameter distribution of the electrospun fibers by using ImageJ software.
Culture of ARPE-19 cells
The electrospun R2C/PLCL scaffolds were cut into 3 cm × 3 cm squares and then sterilized by UV light at 254 nm for 60 min. The ARPE-19 cells (CRL-2302, ATCC, USA) were cultured in DMEM/F-12 (11320033, Gibco, USA) containing 10% fetal bovine serum (10091148, Gibco, USA) and 1% penicillin-streptomycin (15140163, Gibco, USA) in a 5% CO2 humidified incubator at 37 °C. The medium was changed every two days. After 80% confluence, cells were digested with 0.25% trypsin (15090046, Gibco, USA) at 37 °C for 30 s, scraped off and centrifuged at 74 g for 3 min. Cells were seeded in culture at a density of 1 × 106 cells/mL and co-cultured with the electrospun R2C/PLCL scaffolds in a 5% CO2 humidified incubator at 37 °C, while ARPE-19 cells were cultured without the electrospun R2C/PLCL scaffolds as a control.
Transcriptomic analysis
After being cultured for 24 h, the ARPE-19 cells (CRL-2302, ATCC, USA) that were co-cultured with or without the electrospun R2C/PLCL scaffolds were detached with 0.25% trypsin at 37 °C for 30 s and collected after centrifugation at 74 g for 3 min. Total RNA of the ARPE-19 cells was extracted by using TRIzol reagent (15596026, Invitrogen, USA). The detection of RNA concentration and purity was performed via NanoDrop 2000 (ThermoFisher, USA). The RNA samples were sent to Shanghai Majorbio Bio-Pharm Technology Co., Ltd (Shanghai, China) for commercial RNA-seq. Differentially expressed genes (DEGs), with an adjusted p value (padj) < 0.05, were identified by using DESeq2.
Statistical analysis
All the experiments were performed as three independent replicates and the data are reported as mean values with standard deviation (±SD). The OriginPro 2021 software (OriginLab Inc., USA) was used to statistically analyze the data.
Results
Expression and purification of spidroin R2C
The mini-spidroin R2C, with a theoretical molecular weight of 40.8 kDa, consists of a MiSp Rep2 domain of 367 amino acids and a MiSp CT domain of 144 amino acids ().
Figure 3. Amino acid sequence of spidroin R2C. The spidroin R2C consists of a Rep2 domain and a CT domain. The DNA sequences of the Rep2 and CT domains were from A. ventricosus MiSp (GeneBank accession number AFV31615.1) and were translated into amino acid sequences via DNAMAN software. The amino acid sequences of Rep2 and CT domains were marked with green and blue shading, respectively.
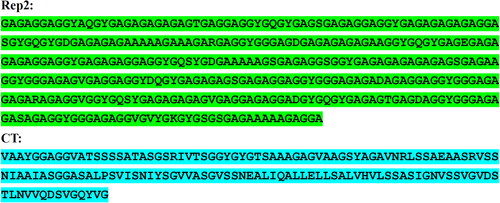
The expressed and purified spidroin R2C was detected by using SDS-PAGE electrophoresis (). Compared with the uninduced cells (Lane 1), a new weak band between 45.0 kDa and 66.2 kDa appeared in the IPTG-induced cells (Lane 2). After ultrasonic crushing, the target band appeared in the supernatant (Lane 3), while it was almost absent in the pellets (Lane 4). The fusion protein was then purified from the cell lysate supernatants via a Ni-NTA column. After the supernatants passed through the Ni-NTA column, the fusion protein was absolutely adsorbed on the Ni-NTA column, as evidenced by its absence in the flow-through of the Ni-NTA column (Lane 5). After washing with the wash buffer, the fusion protein 6 × His-SUMO-R2C was eluted by the elution buffer containing 200 mmol/L imidazole (Lane 6). The eluted fusion protein 6 × His-SUMO-R2C was then digested by SUMO protease to cut off the 6 × His-SUMO tag and release the spidroin R2C (Lane 7). The higher new band (Lane 7) between 35.0 kDa and 45.0 kDa was indicated as spidroin R2C. The molecular size of the spidroin R2C was consistent with the theoretical molecular weight of the purified R2C. By using SDS-PAGE analysis, the purified spidroin R2C was shown to have high purity (Lane 8).
Figure 4. SDS-PAGE analysis of the recombinant spidroin R2C. Lane M, Protein marker (C600525, Sangon, Shanghai, China); lane 1, lysates of uninduced cells; lane 2, lysates of induced cells; lane 3, supernatant of cell lysates; lane 4, pellet of cell lysates; lane 5, flow-through of the Ni-NTA column by passing the supernatants through the Ni-NTA column; lane 6, eluate of Ni-NTA column by using elution buffer; lane 7, fragments after SUMO protease digestion; lane 8, purified spidroin R2C. The target band of spidroin R2C is indicated by the black arrow.
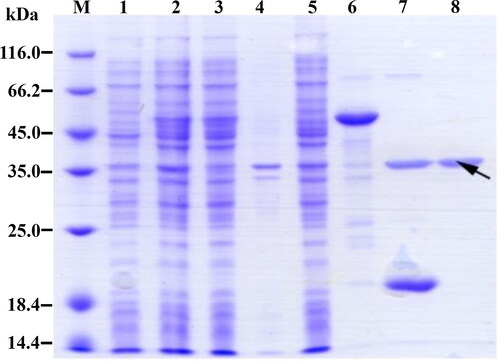
Preparation and characterization of wet-spun fibers of R2C
When injected into the coagulation solution (90% methanol), continuous silk-like fibers longer than 10 cm could be formed by the wet-spinning solution, in which the purified spidroin R2C was dissolved in HFIP at a final concentration of 10% (w/v) (). SEM microscopy showed that the wet-spun fibers of R2C possessed smooth surfaces and a uniform diameter of 5.67 ± 0.72 μm ( and ).
Figure 5. Preparation and mechanical properties of the wet-spun fibers of R2C. (A) Continuous silk-like fibers formed in coagulation solution of 90% methanol. (B) Silk-like fibers more than 10 cm in length. (C) SEM micrography of the wet-spun fiber of R2C using the full backscattered electron detector. The scale bar is 4 μm. (D) Stress-strain curve of the wet-spun fibers of R2C.
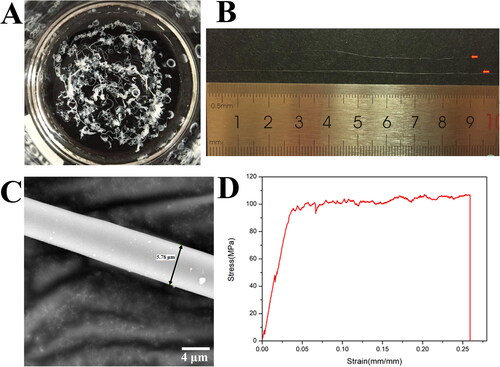
Table 1. Mechanical properties of the wet-spun fibers of R2C.
By using inverted phase-contrast microscopy, the fibers with a smooth surface and uniform diameter (Supplemental Figure S1) were selected to test the mechanical properties of the fibers, which were shown in the typical stress-strain curve () and summarized in . The wet-spun fibers of R2C have sufficient mechanical performance with breaking strain of 0.26 ± 0.07 mm/mm, breaking stress of 106.68 ± 20.53 MPa, a Young’s modulus of 3.51 ± 0.92 GPa and toughness of 24.67 ± 9.51 J/m3.
Preparation and characterization of nanofibrous R2C/PLCL scaffolds
In this experiment, the electrospinning solution of spidroin R2C/PLCL, in which the spidroin R2C and PLCL were dissolved in HFIP at a total concentration of 7.5% and 2.5% (w/v), respectively, was successfully electrospun into nanofibrous scaffolds at a high voltage of 15 ± 1 kV. The morphology of the nanofibrous R2C/PLCL scaffold was observed by SEM microscopy, which demonstrated that the scaffolds were fabricated of randomly distributed nanometer-size fibers and closely interconnected to form pore structures (). The average diameter of the R2C/PLCL scaffold was calculated to be 751.70 ± 19.48 nm ().
Figure 6. Preparation of the electrospinning R2C/PLCL scaffolds and their effects on ARPE-19 cells. (A) SEM micrography of the electrospinning R2C/PLCL scaffolds using the full backscattered electron detector. The scale bar is 10 μm. (B) Fiber diameter distribution of the electrospinning R2C/PLCL scaffolds. (C) Volcano plot of differential gene expression after co-culture of ARPE19 cells with membranes. (D) Heatmap of differential gene expression with a threshold of a log2 FoldChange more than 3 and a p adjust value less than 0.05. The volcano plot and heatmap were generated by the online tool of Majorbio Cloud Platform (https://cloud.majorbio.com/page/tools/).
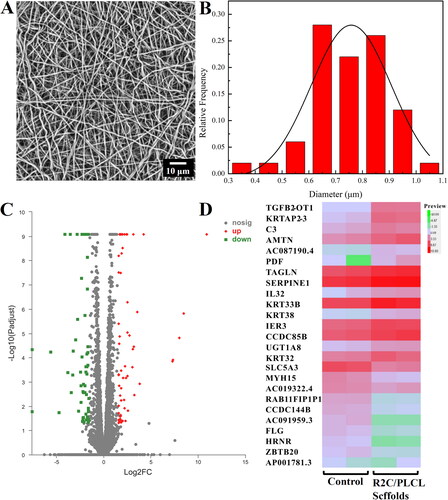
Transcriptomic analysis of differentially expressed genes
To gain insight into the effects of the nanofibrous R2C/PLCL scaffolds on RPE cells, ARPE-19 cells were co-cultured with the R2C/PLCL scaffolds, while those without the R2C/PLCL scaffolds were used as the control. After a 24-h incubation, the RNA of the ARPE-19 cells was extracted and was used for analysis of the transcriptomic differences, which were demonstrated in volcano plots (). By using the threshold of a log2 FoldChange greater than 3 and a padj < 0.05, 21 DEGs, including 14 up-regulated genes and 7 down-regulated genes, were detected. The up- and down-regulated genes were presented in a heatmap (), and the details of those were summarized in and , respectively.
Table 2. Up-regulated genes with a log2 FoldChange more than 3.
Table 3. Down-regulated genes with a log2 FoldChange more than 3.
Discussion
Spider silk, a natural polymer made of spidroins, has long been regarded as one of the most attractive materials due to its exceptional combination of high strength and extensibility [Citation39]. However, because the majority of spiders exhibit cannibalistic and territorial behavior, it is challenging to collect natural spider silk in sufficient amounts [Citation40]. It seems to be more suitable to obtain spider silk protein through heterologous expression. However, it is extremely difficult to heterologously express spidroins of natural sizes, whereas truncated miniature spidroins, which only have 1-4 repeated Rep domains fused to CT domains, can self-assemble to create fibers successfully in the absence of NT domains [Citation41–43]. For orb-weaving spiders, at least seven types of spidroins have been synthesized to spider silks for different applications [Citation44]. Due to its strength and lack of supercontraction in water, minor ampullate silk may be especially useful for biomedical applications. Therefore, a mini-MiSp spidroin was constructed in this study. Fusion to the SUMO tag improved the solubility of the spidroin in E. coli BL21 (DE3). By using the wet-spun method, continuous silk-like fibers could be formed by the spidroin R2C. The wet-spun fibers had smooth surfaces and uniform diameters. The mean diameter of the wet-spun fibers (5.67 ± 0.72 μm) was larger than that of the natural MiSp silk (0.69 ± 0.10 μm or 1.8 ± 0.1 μm) [Citation45,Citation46]. The wet-spun fibers of R2C possessed sufficient mechanical properties for biomedical applications, although their mechanical parameters were inferior to those of natural MiSp silk [Citation45,Citation46].
Due to the age-related deterioration of the photoreceptors and RPE, AMD, manifested as the accumulation of drusen, severely impairs the central vision of people older than 50. Cell-based therapy could be used to cure dry AMD. The cells directly injected into the body, however, cannot survive without the resorbable, biocompatible and thin scaffolds that are used to restore the impaired photoreceptors, or RPE [Citation47]. Although an extensive range of synthetic materials have been used to fabricate the scaffolds, the addition of natural biocompatible polymers should be used to improve the mechanical performance and biocompatibility of the hybrid scaffolds. In addition, the electrospinning technique is among the most preferred methods for creating ultrathin membranes composed of nanometric fibers [Citation48]. Therefore, in this study, the spidroin R2C/PLCL blend nanofibrous scaffolds were electrospun to simulate Bruch’s membrane. Bruch’s membrane, which ranges in diameter from 50 to 500 nm and is less than 5 μm thick, is where native RPE cells are located [Citation24]. Due to their large size, ARPE-19 cells require a larger surface area for cell attachment. ARPE-19 cells adhered and proliferated more readily to bioactive glass nanoparticle loaded PCL with a fiber diameter of 344 ± 45 nm than they did to PCL-only nanofibers with a diameter of 109 ± 10 nm [Citation49]. In addition, compared with PCL scaffolds with a diameter of 527 ± 184 nm, ARPE-19 cells grown on PCL scaffolds with a diameter of 1309 ± 116 nm possessed significantly higher confluency and adhesion [Citation50]. Here, the mean fiber diameter of the R2C/PLCL scaffolds was determined to be 751.70 ± 19.48 nm, which was sufficient for ARPE-19 cell attachment.
When the effects of the R2C/PLCL scaffolds on ARPE-19 cells were investigated, several DEGs were identified. Compared with the control cells, 14 genes, including TGFB2-OT1, KRTAP2-3, C3, AMTN, PDF, TAGLN, SERPINE1, IL32, KRT33B, KRT38, IER3, CCDC85B, UGT1A8 and KRT32, were upregulated more than three times. One category of the up-regulated genes is related to inflammation or tumors. Inflammatory inducers can promote the high expression of TGFB2-OT1, which causes autophagy and inflammation in vascular endothelial cells [Citation51]. The overexpression of SERPINE1 can promote the malignant progression and contribute to the poor prognosis of gastric cancer [Citation52], while CCDC85B is highly expressed in the cytoplasm of non-small cell lung cancer tumor cells [Citation53]. More upregulated genes possess the ability to suppress inflammation and tumors. TAGLN, as a tumor suppressor [Citation54], triggers inflammation when TAGLN3 is downregulated [Citation55]. Meanwhile, IER3 exhibits powerful anti-inflammatory activity by counter-regulating NF-κB [Citation56]. As a multifaceted cytokine associated with inflammatory conditions, IL32 not only acts as a pro-inflammatory cytokine in various cells but also induces other anti-inflammatory cytokines [Citation57,Citation58]. Compared with normal tissues, the expression of UGTs has different regulatory patterns in tumors, either promoting the progression of some tumor types or inhibiting the progression of others [Citation59]. Although complement protein C3 can be inappropriately activated in response to biomaterials or transplantation, thereby triggering inflammation, the complement system is mainly considered the host defense system [Citation60]. As a class of metalloenzyme, PDF can be used as a new target for antibacterial, antimalarial and anticancer drugs [Citation61–63].
The other category of upregulated genes is related to keratinization. AMTN is expressed during tooth enamel maturation, which promotes bone tissue mineralization [Citation64]. All of KRTAP2-3, KRT33B, KRT38 and KRT32 belong to the keratins, whose major function is providing a scaffold for epithelial cells and tissues to keratinize epithelial cells [Citation65].
Genes with more than triple downregulation were HRNR, ZBTB20, SLC5A3, FLG, MYH15, CCDC144B and RAB11FIP1P1. HRNR and ZBTB20 act as oncogenes [Citation66,Citation67], and their downregulation is conducive to the normal growth and reproduction of cells. Additionally, SLC5A3, FLG, MYH15, and RAB11FIP1P1 are mostly associated with substance transport and homeostasis [Citation68–71].
Conclusions
In this study, the expression plasmid of a mini-MiSp spidroin, containing the MiSp Rep2 and the MiSp CT domain from the spider A. ventricosus, was successfully constructed. The spidroin R2C was successfully expressed in E. coli and purified to a high purity. By using the wetspinning technique, silk-like fibers with excellent mechanical properties were formed. By using the electrospinning technique, the nanofibrous R2C/PLCL scaffolds of a combination of spidroin R2C and PLCL at a ratio of 3:1 were formed. The transcriptomic effects of the R2C/PLCL scaffolds on ARPE-19 cells showed that the scaffolds did not have a considerable impact on the ARPE-19 cells and have the potential to be explored as a Bruch’s membrane for RPE transplantation in the future.
Author contributions
Conceived and designed the experiments: C.-J. Liu and E. Meng. Performed the experiments: C.-J. Liu, K. Yi, Z.-Y. Zhang, Q.-Y. Li, H.-M. Li, C. Qu, M.-K. Chen, K.-L. Jin, and E. Meng. Analyzed the data: C.-J. Liu and E. Meng. Contributed reagents/materials/analysis tools: C.-J. Liu and E. Meng. Wrote and revised the paper: C.-J. Liu. Critically read the paper: E. Meng. All authors have read and approved the final manuscript and have agreed on the journal to which it is submitted.
Supplemental Material
Download PDF (86 KB)Acknowledgements
The authors thank Prof. Qing Meng from Donghua University for generously donating the DNA sequences of MiSp from the spider A. ventricosus. They thank Sangon (Shanghai, China) for primer synthesis and DNA sequencing. They thank Shanghai Majorbio Bio-Pharm Technology Co., Ltd (Shanghai, China) for commercial RNA-seq.
Data availability statement
The data that support the findings from this study are available from the corresponding author [EM], upon reasonable request.
Disclosure statement
No potential conflict of interest was reported by the authors.
Additional information
Funding
References
- Lin JB, Apte RS. Nad + and sirtuins in retinal degenerative diseases: a look at future therapies. Prog Retin Eye Res. 2018;67:1–12.
- Kaur G, Singh NK. The role of inflammation in retinal neurodegeneration and degenerative diseases. Int J Mol Sci. 2021;23(1):386.
- WHO. Blindness and vision impairment. Geneva (Switzerland): World Health Organisation; 2022. Available from: https://www.who.int/en/news-room/fact-sheets/detail/blindness-and-visual-impairment
- Wong WL, Su X, Li X, et al. Global prevalence of age-related macular degeneration and disease burden projection for 2020 and 2040: a systematic review and meta-analysis. Lancet Glob Health. 2014;2(2):e106–e116.
- Fleckenstein M, Keenan TDL, Guymer RH, et al. Age-related macular degeneration. Nat Rev Dis Primers. 2021;7(1):31.
- Papadopoulos Z. Recent developments in the treatment of wet age-related macular degeneration. Curr Med Sci. 2020;40(5):851–857.
- Stahl A. The diagnosis and treatment of age-related macular degeneration. Dtsch Arztebl Int. 2020;117(29–30):513–520.
- Kinnunen K, Petrovski G, Moe MC, et al. Molecular mechanisms of retinal pigment epithelium damage and development of age-related macular degeneration. Acta Ophthalmol. 2012;90(4):299–309.
- Rohiwal SS, Ellederová Z, Ardan T, et al. Advancement in nanostructure-based tissue-engineered biomaterials for retinal degenerative diseases. Biomedicines. 2021;9(8):1005.
- Trese M, Regatieri CV, Young MJ. Advances in retinal tissue engineering. Materials (Basel). 2012;5(1):108–120.
- Christiansen AT, Tao SL, Smith M, et al. Subretinal implantation of electrospun, short nanowire, and smooth poly(-caprolactone) scaffolds to the subretinal space of porcine eyes. Stem Cells Int. 2012;2012:454295.
- Lawley E, Baranov P, Young M. Hybrid vitronectin-mimicking polycaprolactone scaffolds for human retinal progenitor cell differentiation and transplantation. J Biomater Appl. 2015;29(6):894–902.
- Sorkio AE, Vuorimaa-Laukkanen EP, Hakola HM, et al. Biomimetic collagen i and iv double layer langmuir–schaefer films as microenvironment for human pluripotent stem cell derived retinal pigment epithelial cells. Biomaterials. 2015;51:257–269.
- Del Priore LV, Tezel TH, Kaplan HJ. Survival of allogeneic porcine retinal pigment epithelial sheets after subretinal transplantation. Invest Ophthalmol Vis Sci. 2004;45(3):985–992.
- Gandhi JK, Manzar Z, Bachman LA, et al. Fibrin hydrogels as a xenofree and rapidly degradable support for transplantation of retinal pigment epithelium monolayers. Acta Biomater. 2018;67:134–146.
- Kearns VR, Tasker J, Akhtar R, et al. The formation of a functional retinal pigment epithelium occurs on porous polytetrafluoroethylene substrates independently of the surface chemistry. J Mater Sci Mater Med. 2017;28(8):124.
- Lu L, Garcia CA, Mikos AG. Retinal pigment epithelium cell culture on thin biodegradable poly(dl-lactic-co-glycolic acid) films. J Biomater Sci Polym Ed. 1998;9(11):1187–1205.
- Surrao DC, Greferath U, Chau Y-Q, et al. Design, development and characterization of synthetic Bruch’s membranes. Acta Biomater. 2017;64:357–376.
- Redenti S, Neeley WL, Rompani S, et al. Engineering retinal progenitor cell and scrollable poly (glycerol-sebacate) composites for expansion and subretinal transplantation. Biomaterials. 2009;30(20):3405–3414.
- Redenti S, Tao S, Yang J, et al. Retinal tissue engineering using mouse retinal progenitor cells and a novel biodegradable, thin-film poly(e-caprolactone) nanowire scaffold. J Ocul Biol Dis Infor. 2008;1(1):19–29.
- Richbourg NR, Peppas NA, Sikavitsas VI. Tuning the biomimetic behavior of scaffolds for regenerative medicine through surface modifications. J Tissue Eng Regen Med. 2019;13(8):1275–1293.
- Warnke PH, Alamein M, Skabo S, et al. Primordium of an artificial bruch’s membrane made of nanofibers for engineering of retinal pigment epithelium cell monolayers. Acta Biomater. 2013;9(12):9414–9422.
- Pritchard CD, Arnér KM, Langer RS, et al. Retinal transplantation using surface modified poly(glycerol-co-sebacic acid) membranes. Biomaterials. 2010;31(31):7978–7984.
- Hotaling NA, Khristov V, Wan Q, et al. Nanofiber scaffold-based tissue-engineered retinal pigment epithelium to treat degenerative eye diseases. J Ocul Pharmacol Ther. 2016;32(5):272–285.
- Sun H, Mei L, Song C, et al. The in vivo degradation, absorption and excretion of pcl-based implant. Biomaterials. 2006;27(9):1735–1740.
- Xu L, Crawford K, Gorman CB. Effects of temperature and ph on the degradation of poly(lactic acid) brushes. Macromolecules. 2011;44(12):4777–4782.
- Lasprilla AJR, Martinez G, Lunelli BH, et al. Poly-lactic acid synthesis for application in biomedical devices—a review. Biotechnol Adv. 2012;30(1):321–328.
- Labet M, Thielemans W. Synthesis of polycaprolactone: a review. Chem Soc Rev. 2009;38(12):3484–3504.
- Zhang M, Chang Z, Wang X, et al. Synthesis of poly(l-lactide-co-ε-caprolactone) copolymer: tructure, toughness, and elasticity. Polymers. 2021;13(8):1270.
- Fernández J, Etxeberria A, Sarasua J-R. Synthesis, structure and properties of poly(l-lactide-co-ε-caprolactone) statistical copolymers. J Mech Behav Biomed Mater. 2012;9:100–112.
- Wang X, Liu J, Jing H, et al. Biofabrication of poly(l-lactide-co-ε-caprolactone)/silk fibroin scaffold for the application as superb anti-calcification tissue engineered prosthetic valve. Mater Sci Eng C Mater Biol Appl. 2021;121:111872.
- Zhang D, Ni N, Chen J, et al. Electrospun sf/plcl nanofibrous membrane: a potential scaffold for retinal progenitor cell proliferation and differentiation. Sci Rep. 2015;5(1):14326.
- Wohlrab S, Müller S, Schmidt A, et al. Cell adhesion and proliferation on rgd-modified recombinant spider silk proteins. Biomaterials. 2012;33(28):6650–6659.
- Belbéoch C, Lejeune J, Vroman P, et al. Silkworm and spider silk electrospinning: a review. Environ Chem Lett. 2021;19(2):1737–1763.
- Leem JW, Fraser MJ, Kim YL. Transgenic and diet-enhanced silk production for reinforced biomaterials: a metamaterial perspective. Annu Rev Biomed Eng. 2020;22(1):79–102.
- Hardy JG, Leal-Egaña A, Scheibel TR. Engineered spider silk protein-based composites for drug delivery. Macromol Biosci. 2013;13(10):1431–1437.
- Leal-Egaña A, Lang G, Mauerer C, et al. Interactions of fibroblasts with different morphologies made of an engineered spider silk protein. Adv. Eng. Mater. 2012;14(3):B67–B75.
- Liu C-J, Jiang H, Wu L, et al. Oepr cloning: an efficient and seamless cloning strategy for large- and multi-fragments. Sci Rep. 2017;7:44648.
- Li J, Li S, Huang J, et al. Spider silk-inspired artificial fibers. Adv Sci. 2022;9(5):2103965.
- Vendrely C, Scheibel T. Biotechnological production of spider-silk proteins enables new applications. Macromol Biosci. 2007;7(4):401–409.
- Stark M, Grip S, Rising A, et al. Macroscopic fibers self-assembled from recombinant miniature spider silk proteins. Biomacromolecules. 2007;8(5):1695–1701.
- Zhou Y, Rising A, Johansson J, et al. Production and properties of triple chimeric spidroins. Biomacromolecules. 2018;19(7):2825–2833.
- Jia Q, Wen R, Meng Q. Novel highly soluble chimeric recombinant spidroins with high yield. Int J Mol Sci. 2020;21(18):6905.
- Rising A, Johansson J. Toward spinning artificial spider silk. Nat Chem Biol. 2015;11(5):309–315.
- Hayashi CY, Blackledge TA, Lewis RV. Molecular and mechanical characterization of aciniform silk: Uniformity of iterated sequence modules in a novel member of the spider silk fibroin gene family. Mol Biol Evol. 2004;21(10):1950–1959.
- Guinea GV, Elices M, Plaza GR, et al. Minor ampullate silks from nephila and argiope spiders: Tensile properties and microstructural characterization. Biomacromolecules. 2012;13(7):2087–2098.
- Tan YSE, Shi PJ, Choo C-J, et al. Tissue engineering of retina and bruch’s membrane: a review of cells, materials and processes. Br J Ophthalmol. 2018;102(9):1182–1187.
- Greiner A, Wendorff JH. Electrospinning: a fascinating method for the preparation of ultrathin fibers. Angew Chem Int Ed Engl. 2007;46(30):5670–5703.
- Henrique Lima T, Fernandes-Cunha GM, Jensen CEDM, et al. Bioactive glass nanoparticles-loaded poly (ɛ-caprolactone) nanofiber as substrate for arpe-19 cells. J Nanomater. 2016;2016:1–12.
- Krishna L, Nilawar S, Ponnalagu M, et al. Fiber diameter differentially regulates function of retinal pigment and corneal epithelial cells on nanofibrous tissue scaffolds. ACS Appl Bio Mater. 2020;3(2):823–837.
- Huang S, Lu W, Ge D, et al. A new microrna signal pathway regulated by long noncoding rna tgfb2-ot1 in autophagy and inflammation of vascular endothelial cells. Autophagy. 2015;11(12):2172–2183.
- Chen S, Li Y, Zhu Y, et al. Serpine1 overexpression promotes malignant progression and poor prognosis of gastric cancer. J Oncol. 2022;2022:2647825.
- Feng Y, Gao Y, Yu J, et al. Ccdc85b promotes non-small cell lung cancer cell proliferation and invasion. Mol Carcinog. 2019;58(1):126–134.
- Assinder SJ, Stanton J-A, Prasad PD. Transgelin: an actin-binding protein and tumour suppressor. Int J Biochem Cell Biol. 2009;41(3):482–486.
- Arnaud L, Benech P, Greetham L, et al. Apoe4 drives inflammation in human astrocytes via tagln3 repression and nf-κb activation. Cell Rep. 2022;40(7):111200.
- Sina C, Arlt A, Gavrilova O, et al. Ablation of gly96/immediate early gene-x1 (gly96/iex-1) aggravates dss-induced colitis in mice: Role for gly96/iex-1 in the regulation of nf-κb. Inflamm Bowel Dis. 2010;16(2):320–331.
- Aass KR, Kastnes MH, Standal T. Molecular interactions and functions of il-32. J Leukoc Biol. 2021;109(1):143–159.
- Hong JT, Son DJ, Lee CK, et al. Interleukin 32, inflammation and cancer. Pharmacol Ther. 2017;174:127–137.
- Allain EP, Rouleau M, Lévesque E, et al. Emerging roles for udp-glucuronosyltransferases in drug resistance and cancer progression. Br J Cancer. 2020;122(9):1277–1287.
- Ricklin D, Reis ES, Lambris JD. Complement in disease: a defence system turning offensive. Nat Rev Nephrol. 2016;12(7):383–401.
- Escobar-Alvarez S, Goldgur Y, Yang G, et al. Structure and activity of human mitochondrial peptide deformylase, a novel cancer target. J Mol Biol. 2009;387(5):1211–1228.
- Lee MD, She Y, Soskis MJ, et al. Human mitochondrial peptide deformylase, a new anticancer target of actinonin-based antibiotics. J. Clin. Invest. 2004;114(8):1107–1116.
- Sangshetti JN, Khan FAK, Shinde DB. Peptide deformylase: a new target in antibacterial, antimalarial and anticancer drug discovery. Curr Med Chem. 2015;22(2):214–236.
- Tanaka D, Ikeda Y, Ikeda E, et al. Effect of amelotin on bone growth in the murine Calvarial defect model. Ann Biomed Eng. 2021;49(12):3676–3684.
- Bragulla HH, Homberger DG. Structure and functions of keratin proteins in simple, stratified, keratinized and cornified epithelia. J Anat. 2009;214(4):516–559.
- Fu S-J, Shen S-L, Li S-Q, et al. Hornerin promotes tumor progression and is associated with poor prognosis in hepatocellular carcinoma. BMC Cancer. 2018;18(1):815.
- To JC, Chiu AP, Tschida BR, et al. Zbtb20 regulates wnt/ctnnb1 signalling pathway by suppressing pparg during hepatocellular carcinoma tumourigenesis. JHEP Rep. 2021;3(2):100223.
- Cannizzaro M, Jarošová J, De Paepe B. Relevance of solute carrier family 5 transporter defects to inherited and acquired human disease. J Appl Genet. 2019;60(3–4):305–317.
- Čepelak I, Dodig S, Pavić I. Filaggrin and atopic march. Biochem Med (Zagreb). 2019;29(2):020501.
- Rossi AC, Mammucari C, Argentini C, et al. Two novel/ancient myosins in mammalian skeletal muscles: Myh14/7b and myh15 are expressed in extraocular muscles and muscle spindles. J Physiol. 2010;588(Pt 2):353–364.
- Rathan-Kumar S, Roland JT, Momoh M, et al. Rab11fip1-deficient mice develop spontaneous inflammation and show increased susceptibility to Colon damage. Am J Physiol Gastrointest Liver Physiol. 2022;323(3):G239–G254.