Abstract
Cystatin plays a crucial role in immune evasion by parasites. It regulates diverse immune response processes, such as antigen presentation, cytokine and NO production, and phagocytosis. In recent years, an increasing number of parasite cystatins have been identified and studied for the treatment of inflammatory diseases. In contrast, cystatin from Spirometra erinaceieuropaei has received limited research attention. The objective of this study was to utilize bioinformatics tools and molecular biology techniques to predict the biological properties and obtain a recombinant cystatin analogue from Spirometra erinaceieuropaei (SeCystatin). The SeCystatin gene consists of 417 bp and encodes a putative 100-amino acid protein. The predicted molecular weight and isoelectric point of SeCystatin were 10.89 kDa and 6.82, respectively; SeCystatin possesses 12 phosphorylation sites and five post-translational modification sites but has no signal peptide and transmembrane region. The secondary structure of SeCystatin consists of one α-helix, four β-folds and six coils. It also possesses a cystatin-specific conserved domain, QxVxG, which is positioned at the boundary between the first and second β-folds, thereby demonstrating the characteristic features of type I cystatin. In the context of molecular evolution, SeCystatin demonstrates the highest level of evolutionary similarity to Schistocephalus solidus. The SeCystatin gene was fully synthesized, and the recombinant plasmid pET-30a (+)-SeCystatin was constructed to express the target protein in Escherichia coli BL 21. Recombinant SeCystatin was successfully induced for expression by IPTG and subsequently purified using affinity chromatography (Ni-IDA). Our study provides a solid foundation for further studies on the biological functions of SeCystatin.
Introduction
Spirometra erinaceieuropaei, a tapeworm mainly parasitic in felids, can also infect humans as plerocercoids, causing sparganosis. The pathogenicity of intermediate larvae is greater than that of adult worms, making it an important zoonotic parasitic disease [Citation1]. Sparganosis can affect various parts of the human body, and there is a lack of effective diagnostic measures in clinical practice. Most cases are confirmed through postoperative diagnosis, resulting in frequent misdiagnosis and missed diagnoses [Citation2]. Therefore, the development of sensitive and specific immunodiagnostic techniques for the early screening of sparganosis is of great significance. Some key proteins secreted by Spirometra erinaceieuropaei, especially excretory–secretory antigens, can directly interact with the host immune system, serving as crucial inducers of the host’s immune response. Chinese researchers used recombinant Spirometra erinaceieuropaei cysteine protease (rSeCP) protein for the serological diagnosis of sparganosis. The specificity of this method was as high as 98.22% [Citation3], demonstrating that these proteins are ideal candidate diagnostic antigens with excellent prospects and value in clinical applications.
Traditional molecular biology methods have limitations in fully reflecting gene expression differences and functions. They often overlook genes with low expression. However, the key proteins involved in the essential material and energy metabolism of plerocercoids, as well as in triggering the immune response, are likely to be expressed by these neglected genes. Therefore, screening highly specific and sensitive diagnostic antigens using omics technologies, such as high-throughput transcriptomics, has become a current research focus [Citation4]. Next-generation techniques offer several clear advantages, including the detection of a large number of genes, accurate quantification, high repeatability, and the ability to detect low expression genes. Consequently, these technological approaches may also provide a new perspective for exploring potential immunomodulatory proteins within Spirometra erinaceieuropaei.
Cystatin is widely found in trematodes, cestodes, nematodes and protozoa and has unique cysteine protease inhibitory activity and immunomodulatory properties [Citation5]. Cystatin remarkably regulates antigen delivery, cytokine expression and macrophage NO synthesis in the host immune system, thus maintaining its core function in parasite migration and adaptation to parasitic life [Citation6]. As a key component of parasite invasion of host barriers and triggering immune evasion, cystatin has become a widely studied immunomodulatory molecule in “helminth therapy” [Citation7] for treating chronic inflammation-associated diseases [Citation8]. Experimental studies clearly showed the great anti-inflammatory potential of parasite-derived cystatins such as Fasciola gigantica [Citation9], Brugia malayi [Citation10], Schistoma japonicum [Citation11] and Ascaris lumbricoides [Citation12] in various animal disease models such as allergy, rheumatoid arthritis and colitis, indicating a promising future for cystatin as a biotherapeutic drug.
In recent years, advances in bioinformatics technologies have provided new approaches to the study of parasite functional genes. Expressed sequence tag (EST)-based cloning is one of the most popular strategies [Citation13]. Although various parasitic cystatins have been identified and cloned. To the best of our knowledge, these studies mainly focused on trematodes and nematodes. No detailed biological characterization of SeCystatin has been reported. In our previous study, we constructed a full-length cDNA plasmid library of S. erinaceieuropaei using the SMART method [Citation14] and obtained many ESTs and full-length genes, which greatly enriched the biological information of S.erinaceieuropaei.
In this study, a thorough bioinformatics analysis of the SeCystatin gene was performed, followed by the construction of prokaryotic expression vectors to produce the recombinant target proteins. The primary objective of our research was to lay the groundwork for subsequent investigations of SeCystatin function.
Materials and methods
Bioinformatic study of SeCystatin
We employed a methodology similar to that described in our previous study [Citation15]. The full-length cDNA sequence of SeCystatin was obtained from GenBank (JQ963188.1). The basal properties of SeCystatin, including physical and chemical properties, isoelectric point, extinction coefficient amino acid composition, estimated half-life, instability index and protein molecular weight, were all predicted by ProtParam (https://web.expasy.org/protparam/). ProtScale (https://web.expasy.org/protscale/) was used to predict the hydrophilicity/hydrophobicity of SeCystatin. The signal peptide and transmembrane domain were predicted using SignalP 6.0 (https://services.healthtech.dtu.dk/services/SignalP-6.0/) and TMHMM Server version 2.0 (http://www.cbs.dtu.dk/services/TMHMM/), respectively. The subcellular locations of SeCystatin were predicted using TargetP (https://services.healthtech.dtu.dk/services/TargetP-2.0/). The phosphorylation and post-translational modification sites were forecasted using NetPho 3.1 (https://services.healthtech.dtu.dk/services/NetPhos-3.1/) and PROSITE (https://prosite.expasy.org/), respectively. Linear B-cell epitopes of SeCystatin were predicted using DNA star and DNAMAN software. SOPMA (https://npsa-prabi.ibcp.fr) and Phyre2 (http://www.sbg.bio.ic.ac.uk/phyre2/) were employed to obtain information on the secondary and tertiary structures of SeCystatin. SAVES, an online structure validation server (https://saves.mbi.ucla.edu), evaluated the quality of the final predicted SeCystatin model. Clustal Omega (https://www.ebi.ac.uk/Tools/msa/clustalo/) was used for multi-sequence alignments, and the result was further processed using Jalview (https://www.jalview.org/). The neighbor-joining method in MEGA X software was used to generate the molecular evolutionary tree, which was subsequently processed with Evolview (https://www.evolgenius.info/evolview-v2/).
Construction of the prokaryotic expression vector
Based on the SeCystatin sequence and the codon preference of Escherichia coli, the full length of the SeCystatin gene was totally synthesized (Merrybio, Nanjing, China). The fragment was then ligated to pET-30a (cat.no. P3120, Solarbio, Beijing, China) via restriction digest sites NdeI (cat. no. R0111V, New England Biolabs, USA) and HindIII (cat. no. R0104V, New England Biolabs, USA). The recombinant expression vector pET-30a (+)-SeCystatin was subsequently transformed into E. coli DH-5α competent cell (cat.no. CB101, Tiangen, Beijing, China). The transformed competent cells were plated on LB plates containing 50 μg/mL kanamycin (cat. no. K1030, Tiangen, Beijing, China) and then incubated at 37 °C for screening. Positive colonies were selected and inoculated into LB broth. The cultures were then incubated overnight on a shaking incubator at 37 °C. Afterward, the bacterial cells were collected through centrifugation.
The resulting pET-30a (+)-SeCystatin plasmid was subsequently extracted using a plasmid extraction kit (cat. no. DP104, Tiangen, Beijing, China) and subjected to commercial Sanger sequencing by Sangon Biotech (Shanghai, China) using an ABI 3730XL sequenator (Applied Biosystems). The sequencing results were compared and analyzed against the target sequence in GeneBank using DNA star software to ensure that the target gene had been synthesized and connected successfully. The pET-30a (+)-SeCystatin plasmid was solubilized in TE buffer and stored at −20 °C.
Expression and purification of SeCystatin
The recombinant expression vector pET-30a (+)-SeCystatin was transformed into E. coli BL21(DE3) competent cells (cat. no. EC0114, Thermo Fisher Scientific, USA) and incubated overnight at 37 °C on LB agar plates (50 μg/mL kanamycin). A final concentration of 0.2 mmol/L isopropyl-β-d-thiogalactoside (IPTG, cat.no. IB0168, BBI, Canada) was added to the culture of positive clones once the OD600 reached 0.5-0.8. Following this, the culture was incubated at 37 °C for 16 h to induce expression. The induced culture was harvested and lysed by sonication using lysis buffer containing 50 mmol/L Tris (cat.no. TB0196, BBI, Canada), 300 mmol/L NaCl (cat.no. A100241 Sangon, Shanghai, China), 20 mmol/L imidazole (cat.no. IB0277, BBI, Canada) containing 1% Triton X-100 (cat.no. A110694, Sangon, Shanghai, China),1 mmol/L DTT (cat.no. A620058, Sangon, Shanghai, China), and 1 mmol/L PMSF (cat.no.36978, Thermo Scientific, USA). The supernatant was collected by centrifuging the bacterial lysate and incubated with an affinity chromatography column for 90 min. Before incubation, the Ni-IDA affinity chromatography column (cat.no. 70666, Novagen, USA) was equilibrated with a buffer solution of 50 mmol/L Tris (pH 8.0), 300 mmol/L NaCl, and 20 mmol/L Imidazole. Afterwards, the target protein was washed with equilibrium buffer containing imidazole at concentrations of 50 mmol/L and 300 mmol/L, respectively, at a flow rate of 1 min/mL and a total volume of 5 mL.
SDS-PAGE and Western blot test of SeCystatin
The expression level and purification efficiency of SeCystatin were determined by SDS-PAGE with a 15% separation gel using a dedicated kit (cat.no.PG01510-S, Solarbio, Beijing, China). The procedure was primarily performed following the steps described in the referenced protocol [Citation16]. Each collected elution fraction was first mixed with 5 × SDS-PAGE loading buffer and then boiled at 100 °C for 10 min, 4 μL of each diluted sample were loaded vertically into each gel well, and electrophoresis was conducted at a voltage of 120 V for 2 h. The gel was removed and washed with distilled water before being placed in Coomassie Brilliant Blue R-250 staining solution (cat.no. C8430, Solarbio, Beijing, China). The gel was subjected to staining by horizontal shaking at room temperature (RT) for 4 h. The decolorizing solution (1 L) contained 250 mL of ethanol and 75 mL of glacial acetic acid. The gel was immersed in the decolorizing solution, heated in low power mode in a microwave oven for 1 min, and then the decolorizing solution was replaced after 15 min of standing. These steps were repeated twice to complete the decolorization process until no clear blue background was observed. ImageLab software version (Bio-Rad) was used for image acquisition.
Pure recombinant SeCystatin was collected after purification by Ni-IDA affinity chromatography and dialyzed into 1× PBS buffer (pH 7.4) and 5% glycerol. After filtering SeCystatin through a 0.22 μm filter. The purified SeCystatin was aliquoted and stored frozen at −80 °C for purity and specificity validation. Briefly, 4 μL purified protein along with an equal volume of protein marker (cat. no. MY1397, MerryBio, Nanjing, China) were vertically loaded into gel wells and were first subjected to 15% SDS-PAGE at 100 V in ice water for 1h. The separated protein from the gel was then transferred to the PDVF membrane. After blocking with 5% skim milk powder for 2h at room temperature, western blotting was subsequently performed following the steps described by Kim [Citation17]. The primary antibody used in this study was the mouse anti-6X His tag antibody (cat. no. AF2879, Beyotime Biotechnology, Shanghai, China, https://www.beyotime.com/product/AF2879-200%CE%BCl.htm). The PVDF membrane was incubated in a primary antibody solution (1:500) for 2 h at RT. For the secondary antibody, horseradish peroxidase-labeled goat anti-mouse IgG was used (cat. no. A0216, Beyotime Biotechnology, Shanghai, China https://www.beyotime.com/product/A0216.htm). The PVDF membrane was incubated in the secondary antibody solution (1:1000) for 1 h at RT. The reaction was terminated with deionized water after 3,3′-diaminobenzidine (DAB) color development until the target band appeared.
Results
Basic chemical and physical characteristics of SeCystatin
The SeCystatin gene sequence was 417 bp in length with the biggest ORF starting from 1 bp and ending at 303 bp, which encodes a predicted 100 amino-acid protein (). The molecular formula of SeCystatin is C478H777N133O143S7 with a relative molecular weight of 10.89 kDa and a theoretical pI of 6.82. SeCystatin has an estimated half-life of 30 h in mammals, which is longer than that in E. coli (10 h) and yeast (20 h). The molar extinction coefficient (ε) at 280 nm was 3230 mol−1 cm−1. The aliphatic index was 87.60 and GRAVY was −0.159. Multiple hydrophilic and hydrophobic regions were predicted on SeCystatin (). The hydrophilic areas are predominantly located in 9–20 aa, 30–40 aa, 48–52 aa, 56–67 aa and 90–96 aa. SeCystatin was predicted to be a non-transmembrane protein because signal peptide and transmembrane structures were not predicted by Signal 4.1 and TMHMM (). The probability of SeCystatin being a signal peptide was determined to be 0.0227, whereas the probability of it being a mitochondrial protein was 0.073. Nevertheless, with a maximum likelihood of 0.9043 for localization in other cellular compartments, SeCystatin is primarily a cytosolic protein.
Phosphorylation and post-translational modification sites of SeCystatin
The predicted SeCystatin contains 12 phosphorylation sites (), seven serine phosphorylation sites located at 8, 9, 14, 26, 36, 62 and 91, three threonine phosphorylation sites located at amino acids 47, 49 and 53, two tyrosine phosphorylation sites located at amino acids 55 and 99. In addition, five post-translational modification motifs were predicted, including two N-myristoylation sites (5–10, 6–11), one protein kinase C phosphorylation site (9–11), and two casein kinase II phosphorylation sites (14–17, 36–39).
Linear B cell epitopes of SeCystatin
As illustrated in , the five consecutive peaks above the horizontal line represent multiple epitopes, suggesting that the potential epitopic areas are located at the following amino acid residue: 5–21, 32–48, 59–68, 75–84 and 86–98. In addition, DNAMAN also obtained five potential B cell epitopes (). Both methods predicted the same number of epitopes, and the antigenic locations largely matched, indicating high confidence in the results.
Table 1. Potential B-cell epitopes of SeCystatin predicted by DNAMAN.
Secondary and three-dimensional structures of SeCystatin
In the secondary structures of SeCystatin, the alpha helix accounted for 26%, while β-strands and random coils accounted for 32% and 34%, respectively (). The 3D structure prediction results showed that SeCystatin has one alpha-helix and four β-folds and six random coils, which are consistent with the analysis of the secondary structure. The conserved sequence QxVxG is positioned at the end of the initial β-fold and the start of the second β-fold (). The ERRAT methods in the SAVES program were selected to validate the structural quality of the predicted SeCystatin. ERRAT2 () showed that the overall quality score of the SeCystatin model is 98.913, which is greater than 95%, indicating a high-quality model structure.
Figure 5. Prediction of protein structure and model quality of SeCystatin. (A) Secondary structure composition predicted by PSIPRED. (B) Three-dimensional structure constructed by Phyre 2 based on homology modeling; the red arrow points to the QxVxG conserved domain. (C) Quality validation of homology modeled SeCystatin structures by Errat. Yellow bar indicates protein regions with misfolding at 95% confidence level.
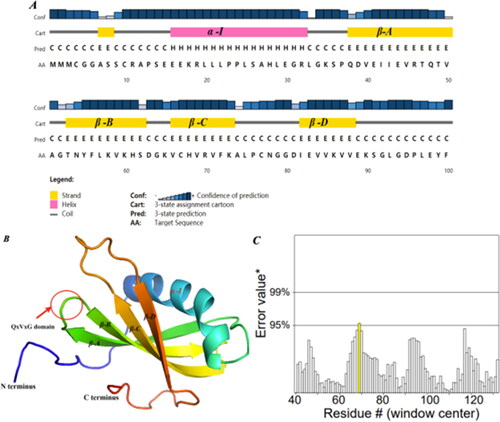
Multiple cystatin sequence alignment and phylogenetic study
Amino acid sequences of cystatin from 10 different parasite species were retrieved from the Pfam database, including Schistocephalus solidus (A0A0X3NVK1), Dibothriocephalus latus (A0A3P7LN44), Fasciola gigantica (C6GC97), Echinococcus granulosus (U6JAU0), Taenia asiatica (A0A0R3W5M0), Fasciola hepatica (A0A2H1BVZ9), Paragonimus westermani (A0A5J4N7Q9), Clonorchis sinensis (A0A419Q945), Schistosoma japonicum (F0UXG3) and Schistosoma mansoni (A0A067XIP6). SeCystatin shares 26 consensus amino acid residues with these selected cystatin sequences (). SeCystatin exhibited the lowest level of sequence similarity, measuring 40.59%, when compared with S. japonicum cystatin. In contrast, the highest level of similarity, amounting to 86.14%, was observed compared with Schistocephalus solidus cystatin. The conserved domains of Secystatin-QxVxG were also located at the 45–49 AA site. Phylogenetic analysis revealed that SeCystatin exhibits a relatively close relationship with cestode species such as Schistocephalus solidus and Dibothriocephalus latus. However, it demonstrates distant relationships with trematode species, including Schistosoma japonicum and Fasciola gigantica ().
Figure 6. Multiple sequence alignments of cystatin. Different colored columns represent conserved sites among cystatin sequences. The letters in the consensus sequence represent the identical amino acid residues in the compared sequences. "ID%" refers to the percentage of similarity between each sequence and SeCystatin. All sequences were aligned using Clustal Omega and edited using Jalview.
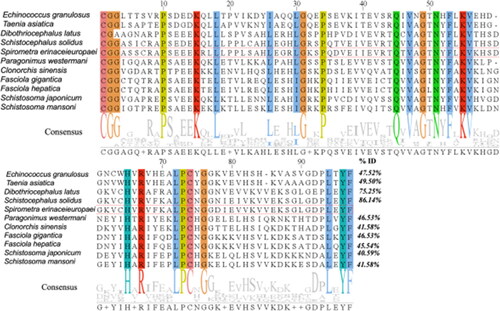
Purification and quality verification of SeCystatin
As illustrated in , SeCystatin was highly expressed and predominantly present in the soluble supernatant, and the clearest band was observed in lane 5, indicating that 300 mmol/L imidazole exerted the best purification effect. The purified protein was analyzed by SDS-PAGE and western blotting (anti-His-tag); two clear specific bands were detected at approximately 11 kDa, consistent with the expected molecular weight. Both validations confirmed that SeCystatin exists as a monomer with a high level of purity ().
Figure 8. Expression, purification, and verification of SeCystatin. (A) Expression and purification results of SeCystatin analyzed by SDS-PAGE. Lane M: SDS-PAGE protein marker (cat. no. MY1397, MerryBio, Nanjing, China). Lane1: Supernatant after centrifugation of whole bacteria. Lane 2: Supernatant after incubation with Ni-IDA for 90 min. Lanes 3–4: Elution fraction of 50 mmol/L imidazole. Lane 5: Elution fraction of 300 mmol/L imidazole. (B) Quality and specificity of SeCystatin were verified by SDS-PAGE and Western blot analysis. Lane 1-2: Purified SeCystatin. M1: SDS-PAGE protein marker (cat. no. MY1397, MerryBio, Nanjing, China). M2: Western blot marker (cat. no. MY00521, MerryBio, Nanjing, China).
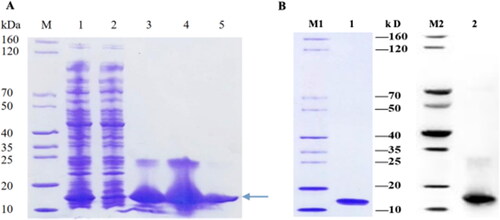
Discussion
Parasitic cystatin plays a crucial role in inducing immunosuppression during parasite invasion and exhibits significant immunomodulatory effects [Citation18]. Its main mechanisms of action involve inhibiting antigen processing and presentation, suppressing pattern recognition receptors and activating the NF-κB dependent pathway, ultimately leading to the release of proinflammatory cytokines [Citation19]. Based on molecular mass, size, structure and subcellular localization, cystatins are divided into three families: stefins (type I), cystatins (type II) and kininogens (type III) [Citation20]. Type I and type II cystatins are more abundant in parasites, with molecular weights ranging from 10 to 15 kDa. Stefins are intracellular proteins lacking glycosylation sites and disulfide bonds and consist of a single structural domain [Citation21]. Cystatins primarily exist as secretory proteins, possessing two disulfide bonds at the C-terminus and frequently containing glycosylation and phosphorylation sites [Citation22]. Kininogens have larger molecular weights, multiple structural domains and are mainly found in mammals, with limited distribution in parasites [Citation23].
In this study, the complete open reading frame of the cystatin gene was obtained from a full-length cDNA library of Spirometra erinaceieuropaei, and its biochemical properties were predicted using bioinformatics methods. The predicted SeCystatin has a molecular mass of 10.9 kDa and exhibits a highly conserved domain, QxVxG, within the cystatin superfamily. Furthermore, SeCystatin cannot form a disulfide bond because of the presence of a conserved Met residue at the N-terminal end and only one Phe residue at the C-terminal end. These findings indicate that SeCystatin is a type I cystatin. It is worth noting that although most stefins are expressed intracellularly, some stefins can also exhibit immunomodulatory functions in a cystatin-like manner. For instance, CsStefin-1 from Clonorchis sinensis [Citation24] is an active cysteine protease inhibitor that protects the parasite from excessive damage caused by endogenous cysteine proteases. Although SeCystatin lacks a signal peptide and is an intracellular protein, we speculated that it may play a significant role in intercellular signaling. However, whether SeCystatin also plays a functional role similar to that of CsStefin-1 requires further functional investigation.
The 3D structural prediction of SeCystatin revealed one α-helix and four β-folds structure, consistent with the structural features of previously reported type I cystatins in parasites [Citation25]. The conserved sequence QxVxG, located at the transition between the first and second β-folds, indicates that the active site for protease inhibition is likely exposed on the protein surface. The β-fold and random coil structures, which predominantly reside on the protein surface, are relatively loose and protruding. The combination of these two structures contributes to approximately 70% of the secondary structure of SeCystatin. These structural features can easily serve as antigenic epitopes and facilitate the antibody binding [Citation26]. Thus, we successfully predicted five potential B-cell linear epitopes using both methods, suggesting that the structure of SeCystatin is highly antigenic.
In recent years, an increasing number of studies have investigated the use of various cystatins as novel antigens in vaccine development, demonstrating promising application prospects. During an Onchocerca volvulus challenge, recombinant onchocystatin exhibited a remarkable capacity to induce a substantial 49% decrease in the worm population [Citation27]. In a mouse model infected with Litomosoides sigmodontis, vaccination with a DNA vaccine based on L. sigmodontis cystatin provided significant protection following an L3 challenge, resulting in a reduction in adult worm numbers and peripheral microfilaremia [Citation28]. Following subcutaneous challenge of Meriones unguiculatus with Brugia malayi infective larvae, vaccination with recombinant Brugia malayi cystatin induces changes in worm migration, homing, and final niche selection [Citation29].
In addition, parasitic cystatin has remarkable immunomodulatory capabilities. Acanthocheilonema viteae cystatin reduces the aggregation of inflammatory cells in the lungs, inhibits the production of IgE, IL-4, and bronchial mucus, significantly decreasing allergic and inflammatory reactions in disease models [Citation30]. Trichinella spiralis Cystatin1 can induce Th2 immune responses, balancing the Th1 immune response induced by TNBS and thus alleviating TNBS-induced colitis [Citation31]. Because of the anti-inflammatory effects of nematode cystatins, they have become a new strategy for treating IBD. The rich B-cell linear epitopes of SeCystatin may also provide a theoretical basis for the future development of epitope vaccines against S. erinaceieuropaei infection. However, whether SeCystatin can also exert the protective effects of a vaccine and the efficacy of an immunomodulatory drug requires further in-depth research.
Using traditional methods to isolate and collect parasitic proteins is a complex and time-consuming process, often resulting in low yields. Furthermore, because of the presence of other components within parasitic organisms, obtaining high-purity proteins is challenging. These impure protein samples greatly constrain subsequent functional studies [Citation32]. Chung et al. [Citation33] employed conventional approaches to obtain cystatin from crude extracts of spargana, the plerocercoid of S. erinaceieuropaei. However, because of limited access to purified cystatin, only partial characterization of this protein was performed. Molecular biology techniques offer precise control over protein expression, resulting in highly pure parasite proteins with reduced impurities [Citation34]. Recombinant proteins produced using molecular biology techniques exhibit improved stability and consistency compared with those produced using traditional methods [Citation35].
In this study the full-length SeCystatin gene was synthesized, and the recombinant plasmid pET-30a (+)-SeCystatin was constructed. As anticipated, recombinant SeCystatin was expressed in the soluble form, and a distinct band with a molecular weight of approximately 11 kDa was detected using SDS-PAGE and western blotting, confirming the successful purification of recombinant SeCystatin. In comparison with traditional techniques, the specificity and sensitivity of SeCystatin were significantly enhanced, providing a reliable and stable source of SeCystatin for subsequent investigations into its protein function.
Conclusions
Our study provides a bioinformatics analysis of SeCystatin from S. erinaceieuropaei for the first time, providing a theoretical basis for future studies to reveal the invasion and immune evasion mechanisms of S. erinaceieuropaei. The successful expression and purification of the recombinant SeCystatin protein also laid a solid foundation for future in-depth studies on the protein function of SeCystatin and the potential development of vaccines and immunomodulatory drugs.
Author contributions section
L.H. performed the bioinformatic analysis of SeCystatin, drafted and revised the manuscript, and contributed to the acquisition of funding for the project [Grant number: HYPY2020029]. L.M. performed the expression and purification of recombinant SeCystatin. G.L. contributed to the interpretation of the findings and provided assistance in the revision and editing of the manuscript. X.C. conceived, designed, and supervised the study, including conceptualizing the research objectives and experimental design, and contributed to the acquisition of funding for the project [Grant number: 819QN235]. All authors have read and approved the final version of the paper.
Acknowledgements
We would like to acknowledge Merrybio (Nanjing, China) for their assistance in synthesizing the full-length SeCystatin gene and constructing the recombinant plasmid pET-30a (+)-SeCystatin, and Sangon Biotech (Shanghai, China) for commercial Sanger sequencing.
Disclosure statement
No potential conflict of interest was reported by the author(s)
Data availability statement
The datasets analyzed in this study are available from the corresponding author upon reasonable request.
Additional information
Funding
References
- Badri M, Olfatifar M, KarimiPourSaryazdi A, et al. The global prevalence of Spirometra parasites in snakes, frogs, dogs, and cats: a systematic review and meta-analysis. Vet Med Sci. 2022;8(6):1–10. doi: 10.1002/vms3.932.
- Liu W, Gong T, Chen S, et al. Epidemiology, diagnosis, and prevention of sparganosis in Asia. Animals. 2022;12(12):1578. doi: 10.3390/ani12121578.
- Liu LN, Wang ZQ, Zhang X, et al. Characterization of Spirometra erinaceieuropaei plerocercoid cysteine protease and potential application for serodiagnosis of sparganosis. PLoS Negl Trop Dis. 2015;9(6):e0003807. doi: 10.1371/journal.pntd.0003807.
- Liu SN, Su XY, Chen WQ, et al. Transcriptome profiling of plerocercoid and adult developmental stages of the neglected medical tapeworm Spirometra erinaceieuropaei. Acta Trop. 2022;232:106483. doi: 10.1016/j.actatropica.2022.106483.
- Klotz C, Ziegler T, Daniłowicz-Luebert E, et al. Cystatins of parasitic organisms. Cyst Prot Pathog Org 2011;712:208–221.
- Joshi P, Mishra PKK. Functional diversity of the excretory/secretory proteins of nematode parasites. Acta Parasitol. 2022;67(2):619–627. doi: 10.1007/s11686-022-00523-7.
- Shi W, Xu N, Wang X, et al. Helminth therapy for immune-mediated inflammatory diseases: current and future perspectives. J Inflamm Res. 2022;15:475–491. doi: 10.2147/JIR.S348079.
- Khatri V, Chauhan N, Kalyanasundaram R. Parasite cystatin: immunomodulatory molecule with therapeutic activity against immune mediated disorders. Pathogens. 2020;9(6):431. doi: 10.3390/pathogens9060431.
- Chantree P, Tarasuk M, Prathaphan P, et al. Type I cystatin derived from Fasciola gigantica suppresses macrophage-mediated inflammatory responses. Pathogens. 2023;12(3):395. doi: 10.3390/pathogens12030395.
- Khatri V, Amdare N, Tarnekar A, et al. Brugia malayi cystatin therapeutically ameliorates dextran sulfate sodium-induced colitis in mice. J Dig Dis. 2015;16(10):585–594. doi: 10.1111/1751-2980.12290.
- Gao S, Li H, Xie H, et al. Therapeutic efficacy of Schistosoma japonicum cystatin on sepsis-induced cardiomyopathy in a mouse model. Parasit Vect. 2020;13(1):260. doi: 10.1186/s13071-020-04104-3.
- Caraballo L, Zakzuk J, Acevedo N. Helminth-derived cystatins: the immunomodulatory properties of an Ascaris lumbricoides cystatin. Parasitology. 2021;148(14):1–13. doi: 10.1017/S0031182021000214.
- Britton C, Laing R, McNeilly TN, et al. New technologies to study helminth development and host–parasite interactions. Int J Parasitol. 2023;53(8):393–403. doi: 10.1016/j.ijpara.2022.11.012.
- LV Gang, Y-J, Lu, Z-G, Fan, et al. Construction and identification of a full-length cDNA library from Spirometra erinaceieuropaei. Chinese J Parasitol. 2010;28:393–394.
- Huang L, Mai L, Zhong K, et al. Bioinformatics analysis, cloning and expression of Spirometra erinaceieuropaei fatty acid-binding protein. PJZ. 2022;54(3):1027–1035. doi: 10.17582/journal.pjz/20210303090323.
- Simpson RJ. SDS-PAGE of proteins. Cold Spring Harb Protoc. 2006;2006(1):pdb.prot4313. doi: 10.1101/pdb.prot4313.
- Kim B. Molecular profiling. Methods in molecular biology. New York (NY): Humana Press; 2017. Chapter 24, Western blot techniques; p. 133–139.
- Tang B, Liu M, Wang L, et al. Characterisation of a high-frequency gene encoding a strongly antigenic cystatin-like protein from Trichinella spiralis at its early invasion stage. Parasit Vect. 2015;8(1):78. doi: 10.1186/s13071-015-0689-5.
- Das NC, Sen Gupta PS, Biswal S, et al. In-silico evidences on filarial cystatin as a putative ligand of human TLR4. J Biomol Struct Dyn. 2022;40(19):8808–8824. doi: 10.1080/07391102.2021.1918252.
- Buša M, Matoušková Z, Bartošová-Sojková P, et al. An evolutionary molecular adaptation of an unusual stefin from the liver fluke Fasciola hepatica redefines the cystatin superfamily. J Biol Chem. 2023;299(3):102970. doi: 10.1016/j.jbc.2023.102970.
- Turk V, Turk D, Dolenc I, et al. Characteristics, structure, and biological role of stefins (type-1 cystatins) of human, mammal, and parasite origin. ACSI. 2019;66(1):5–17. doi: 10.17344/acsi.2018.4639.
- Yang X, Liu J, Yue Y, et al. Cloning, expression and characterisation of a type II cystatin from Schistosoma japonicum, which could regulate macrophage activation. Parasitol Res. 2014;113(11):3985–3992. doi: 10.1007/s00436-014-4064-9.
- Jang SW, Cho MK, Park MK, et al. Parasitic helminth cystatin inhibits DSS-induced intestinal inflammation via IL-10+ F4/80+ macrophage recruitment. Kor J Parasitol. 2011;49(3):245–254. doi: 10.3347/kjp.2011.49.3.245.
- Kang J-M, Lee K-H, Sohn W-M, et al. Identification and functional characterization of CsStefin-1, a cysteine protease inhibitor of Clonorchis sinensis. Mol Biochem Parasitol. 2011;177(2):126–134. doi: 10.1016/j.molbiopara.2011.02.010.
- Ilgová J, Jedličková L, Dvořáková H, et al. A novel type I cystatin of parasite origin with atypical legumain-binding domain. Sci Rep. 2017;7(1):17526. doi: 10.1038/s41598-017-17598-2.
- Zhou P, Zhou Z, Huayu M, et al. A multi-epitope vaccine GILE against Echinococcus multilocularis infection in mice. Front Immunol. 2022;13:1091004. doi: 10.3389/fimmu.2022.1091004.
- Lustigman S, James ER, Tawe W, et al. Towards a recombinant antigen vaccine against Onchocerca volvulus. Trends Parasitol. 2002;18(3):135–141. doi: 10.1016/s1471-4922(01)02211-5.
- Babayan SA, Luo H, Gray N, et al. Deletion of parasite immune modulatory sequences combined with immune activating signals enhances vaccine mediated protection against filarial nematodes. PLoS Negl Trop Dis. 2012;6(12):e1968. doi: 10.1371/journal.pntd.0001968.
- Arumugam S, Zhan B, Abraham D, et al. Vaccination with recombinant Brugia malayi cystatin proteins alters worm migration, homing and final niche selection following a subcutaneous challenge of mongolian gerbils (Meriones unguiculatus) with B. malayi infective larvae. Parasit Vect. 2014;7(1):43. doi: 10.1186/1756-3305-7-43.
- Schnoeller C, Rausch S, Pillai S, et al. A helminth immunomodulator reduces allergic and inflammatory responses by induction of IL-10-producing macrophages. J Immunol. 2008;180(6):4265–4272. doi: 10.4049/jimmunol.180.6.4265.
- Xu JY, Liu MY, Yu PC, et al. Effect of recombinant Trichinella spiralis cysteine proteinase inhibitor on TNBS-induced experimental inflammatory bowel disease in mice. Int Immunopharmacol. 2019;66(66):28–40. doi: 10.1016/j.intimp.2018.10.043.
- Heiber A, Spielmann T. Preparation of parasite protein extracts and Western blot analysis. Bio-protocol. 2014;4(11):e1136–e1136. doi: 10.21769/BioProtoc.1136.
- Chung Y-B, Yang H-J. Partial purification and characterization of a cysteine protease inhibitor from the plerocercoid of Spirometra erinacei. Kor J Parasitol. 2008;46(3):183–186. doi: 10.3347/kjp.2008.46.3.183.
- Karakavuk T, Gül C, Karakavuk M, et al. Biotechnological based recombinant protein vaccines developed against toxoplasmosis. Turkiye Parazitol Derg. 2022;46(4):342–357. doi: 10.4274/tpd.galenos.2022.41636.
- Striepen B. Switching parasite proteins on and off. Nat Methods. 2007;4(12):999–1000. doi: 10.1038/nmeth1207-999.