Abstract
In flooded hypocotyl of soybean (Glycine max), cell division in phellogen and the elongation of these cells are enhanced, and thereby a secondary aerenchyma with high porosity is produced. Auxin controls cell division and cell elongation in many plants, so we studied its role in secondary aerenchyma development. Soybean plants with fully expanded unifoliolate leaves were flooded for 6 d with solutions (100 μM each) of seven anti-auxins. TIBA, NPA, HFCA, 1-NOA, or CHPAA did not restrict the secondary aerenchyma formation, while MH moderately suppressed the aerenchyma development, and PCIB strongly inhibited the development of phellogen and secondary aerenchyma. However, the endogenous IAA concentrations in the flooded hypocotyls did not increase or decrease relative to the controls until 72 h, when a secondary aerenchyma was observed. From these results, it is unclear whether auxin plays an important role in the process of secondary aerenchyma formation under flooding.
1. Introduction
Most wetland species, including rice (Oryza sativa), develop aerenchyma in their roots as an efficient oxygen transport system (snorkel) for root aeration in flooded soils (Justin & Armstrong, Citation1987; Smirnoff & Crawford, Citation1983), so they adapt to the stress and produce high biomass and grain yields in marshes and flooded paddy fields. Soybean (Glycine max), which is a major upland legume crop, is comparatively susceptible to flooding stress, but the plants can acclimate to prolonged flooding because of aerenchyma formation in shoots, roots, and nodules (Hattori et al., Citation2013; Rhine, Stevens, Shannon, Wrather, & Sleper, Citation2010; Shimamura, Mochizuki, Nada, & Fukuyama, Citation2002; Shimamura, Mochizuki, Nada, & Fukuyama, Citation2003; Shimamura, Yamamoto, Nakamura, Shimada, & Komatsu, Citation2010; Thomas, Guerreiro, & Sodek, Citation2005). The aerenchyma is derived from phellogen between stele and cortex, and is called secondary aerenchyma (Fraser, Citation1931).
According to the observations of flooded hypocotyl sections in soybean plants (Shimamura et al., Citation2003, Citation2014), secondary aerenchyma formation occurs basically in three processes: (i) phellogen development between stele and cortex, (ii) aerenchyma cell division from the phellogen, and (iii) aerenchyma cell elongation to develop porous tissues. It is well known that auxin is a plant hormone that controls cell division and cell elongation. The spindly growth of hypocotyls of intact Arabidopsis thaliana seedlings at high temperature (29 °C) results from cell elongation promoted by endogenous auxin (Gray, Östin, Sandberg, Romano, & Estelle, Citation1998) and 1-naphthaleneacetic acid (NAA) stimulates cell elongation and cell division in tobacco (Nicotiana tabacum) cells cultivated in liquid medium (Campanoni & Nick, Citation2005). In well-developing woody stems of Pinus sylvestris (Uggla, Moritz, Sandberg, & Sundberg, Citation1996) and hybrid aspen (Populus tremula × Populus tremuloides) (Tuominen, Puech, Fink, & Sundberg, Citation1997), high indole-3-acetic acid (IAA) concentrations are distributed in the vascular cambial zone, where cell division occurs actively, whereas the mature phloem and xylem contain considerably lower IAA. Because both active cell division and elongation are necessary for secondary aerenchyma formation in this study, we investigated the mechanisms of secondary aerenchyma development in soybean hypocotyls under flooded conditions, focusing on the role of auxin.
2. Materials and methods
2.1. Plant material and growth conditions
The experimental procedures were carried out following the method described by Shimamura et al. (Citation2014). Soybean (Glycine max cv. Asoaogari) seeds were sown in 200 ml of 18–26 mesh silica sand in 400 ml plastic pots (8.5 cm top and 6.5 cm bottom diam. and 10 cm height) with a hole (1 cm diameter) in the bottom (one seed per pot). Chemical fertilizer was not added. The plants were grown in a growth cabinet under artificial light (25 °C, 14 h day/10 h night cycle, 430–590 μmol m−2 s−1 photosynthetically active radiation). After the primary leaves (unifoliolate leaves) had fully expanded (10–11 d after sowing), each treatment was applied. Non-flooded plants (controls) were watered daily, and excess water was dripped from the drainage hole. For the flooding treatment, the planted pot was put on a new one without a hole in the bottom and, prior to flooding with chemical solutions, the sand in the pots was washed with those solutions (approximately 150 ml) to prevent dilution by water in the sand. The plants were maintained under continuously flooded conditions, in which the levels of water or anti-auxin solutions were maintained at 3 cm above the sand surface. Because of evaporation from the pots, the pots were watered daily to maintain the levels of water or anti-auxin solutions. The anti-auxin solutions were renewed after 3 d.
2.2. Treatment with anti-auxins and measurement of secondary aerenchyma formation
Seven anti-auxins were selected: 2,3,5-triiodobenzoic acid (TIBA), N-1-naphthylphthalamic acid (NPA), 9-hydroxy-fluorene-9-carboxylic acid (HFCA), 1-naphthoxyacetic acid (1-NOA), 3-chloro-4-hydroxyphenylacetic acid (CHPAA), maleic hydrazide (MH), and α-(p-chlorophenoxy) isobutyric acid (PCIB). These chemicals have been most widely used as anti-auxins (Jodinskienė & Anisimovienė, Citation2007; Katekar & Geissler, Citation1980; Laňková et al., Citation2010; Leopold and Klein, Citation1951; Mattsson, Sung, & Berleth, Citation1999; Ni, Wang, Xu, & Xia, Citation1999; Oono et al., Citation2003; Ottenschläger et al., Citation2003; Parry et al., Citation2001; Samantarai & Nanda, Citation1979; Zhao & Hasenstein, Citation2010). Although concentrations from low (a few μM) to high (≧100 μM) of these chemicals are applied in many plants, there is little information about these applications to soybean. In order to clear the role of auxin in secondary aerenchyma development, high concentration (100 μM) was adopted as a treatment for anti-auxins in this experiment. Soybean plants were flooded with 100 μM of each anti-auxin solution. After 6 d of treatment, the hypocotyl surfaces and fresh cross sections (100–300 μm in thickness) from five independent plants in each treatment were observed under a stereoscopic microscope (SZX-ILLB100, Olympus Optical Co., Ltd, Tokyo, Japan). Sections of hypocotyls were cut at 1.5 cm above the sand surface using a plant microtome (MTH-1, Nippon Medical & Chemical Instruments Co. Ltd., Osaka, Japan). Some sections were stained with 0.05% (w/v) toluidine blue O and then viewed using a light microscope (DM750, Leica Microsystems, Wetzlar, Germany). To measure the area of each tissue (stele, secondary aerenchyma including phellogen, and cortex including epidermis), digital images were analyzed with ImageJ 1.48v software (National Institutes of Health, Bethesda, MD, USA).
2.3. Measurement of endogenous IAA concentrations in the hypocotyls
The plants were flooded using water or grown without flooding. Flooding treatment was started at 1 h after the initiation of lighting in the growth cabinet. Hypocotyl sections (5 mm, center 1.5 cm above the sand surface, 4–10 independent plants) were excised at 0, 24, 48, 72, and 96 h after treatment.
To extract free IAA from the tissues, the hypocotyl sections were placed in 2-ml microtubes containing 300 μl of cold 80% methanol with 0.1 mg ml−1 of 2,6-ditert-butyl-4-methylphenol and 1.0 ng of 13C6-IAA (Cambridge Isotope Laboratories, Andover, MA, USA), in addition to 6 stainless beads (3 mm diameter) and 10 zirconia beads (2 mm diameter). The tubes were tightly sealed and vigorously shaken using a bead cell disruptor (Micro Smash, TOMY, Tokyo, Japan) at 4,000 rpm four times for 1 min at 4 °C. After centrifugation (15,000 rpm), the supernatants were collected in 2-ml tubes and then evaporated under a N2 gas flow stream. One milliliter of diethyl ether and 500 μl of 2% NaHCO3 were added to the samples, and the mixtures were shaken for 1 min. The diethyl ether phase was removed after centrifugation at 15,000 rpm for 3 min. The aqueous phase was acidified with 1 N HCl to pH 2–3, suspended with diethyl ether, and shaken for 1 min. After centrifugation (15,000 rpm), the diethyl ether phase was evaporated, and then the samples were dried under a N2 gas stream. After the dried solutes were dissolved in 0.8 ml of 50% methanol, IAA was purified using HPLC. IAA fractions were derivatized using N-methyl-N-(trimethylsilyl) trifluoroacetamide and quantified using gas chromatography-selected ion monitoring-mass spectrometry (GC-SIM-MS) (QP5050A, Shimadzu, Kyoto, Japan).
3. Results and discussion
3.1. Effects of anti-auxins on secondary aerenchyma formation
In the controls, neither hypertrophy lenticels on the hypocotyls nor well-developed phellogen between stele and cortex was observed; thus, a secondary aerenchyma was not absolutely formed (Figure A, F and K, and Table ). On the other hand, a secondary aerenchyma (white spongy tissues) was observed through the hypertrophy lenticels under flooded conditions, and phellogen and porous secondary aerenchyma were fully developed out of stele in the cross sections (Figure B, G and L, and Table ).
Figure 1. Surfaces and transverse sections of soybean hypocotyls after 6 d of flooding. The surfaces were photographed under a stereoscopic microscope (A–E and P–S). The sections were excised from hypocotyls at 1.5 cm above the sand surface, and then photographed under a stereoscopic microscope (F–J and T–W) and a light microscope (K–O and X–α). Five plants were used in each treatment. The photographs are surfaces and typical sections from soybean plants grown without flooding (control; A, F, and K) or under flooding with water (B, G, and L), TIBA (C, H, and M), NPA (D, I, and N), HFCA (E, J, and O), 1-NOA (P, T, and X), CHPAA (Q, U, and Y), MH (R, V, and Z) or PCIB (S, W, and α). ae: aerenchyma, co: cortex, pf: phloem fiber, ph: phellogen, xy: xylem. Scale bar = 2 mm (A–E and P–S), 1 mm (F–J and T–W), or 0.2 mm (K–O and X–α).
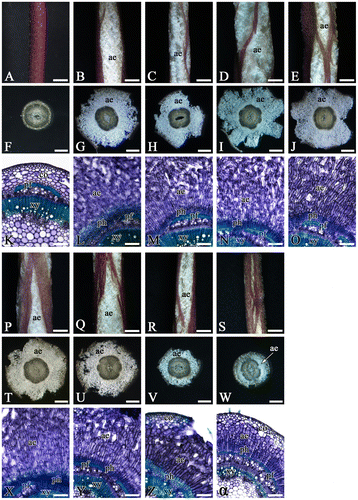
Table 1. Areas of stele, secondary aerenchyma, cortex, and total, as well as the Ae/St ratio in cross sections of soybean hypocotyls.
The plants flooded with 100 μM of each anti-auxin solution had different morphological responses than the plants flooded with water. In the plants flooded with TIBA (Figure C, H and M), NPA (Figure D, I and N), HFCA (Figure E, J and O), 1-NOA (Figure P, T and X), or CHPAA (Figure Q, U and Y) solutions, the secondary aerenchyma was exposed to the atmosphere through the hypertrophy lenticels on hypocotyls, and the area of secondary aerenchyma was 6.79 mm2 (TIBA)–10.53 mm2 (NPA) in the cross sections (Table ), similar to the morphological responses of plants flooded with water. No significant difference in the area of the secondary aerenchyma or Ae/St ratio was observed between water and these chemicals. In addition, these chemicals did not restrict stele growth. Although lenticels and secondary aerenchyma were also observed in the plants flooded with MH (Figure R, V, and Z), aerenchyma formation was considerably inhibited, and both the aerenchyma area and the Ae/St ratio were significantly (approximately 40%) smaller than in the plants flooded with water (Table ). PCIB treatment, however, strongly restricted both lenticels and secondary aerenchyma formation, and the aerenchyma cells differentiated from phellogen were compact or slightly elongated (Figure S, W, and α). In one of the five plants, phellogen developed slightly and secondary aerenchyma cells did not develop at all. Compared to plants flooded with water, the degree of aerenchyma development decreased significantly to 15% in aerenchyma area and 20% in Ae/St ratio, and the growth of stele was also restricted (Table ). During the experiment, no plant died from the water flooding or anti-auxin treatments.
As shown in Figure and Table , TIBA, NPA, HFCA, 1-NOA, and CHPAA did not significantly restrict aerenchyma development as compared with water flooding. This suggests that these anti-auxins are non-negative regulators of the process of secondary aerenchyma formation. The typical morphological effects of TIBA on 31-day-old vegetative soybean plants were reported, such as the shortening of internodes, loss of apical dominance, epinasty of young leaves, and premature abscission of apical leaves and buds (Galston, Citation1947). We also observed the epinasty of primary leaves when soybean plants were flooded with TIBA, HFCA, and NPA solutions (Figure ), indicating the uptake of these chemicals by flooded soybean. The leaf epinasty is closely related to auxin (Kawano, Kawano, & Lapeyrie, Citation2003), so these chemicals probably had an effect on auxin transport in our experiment. In contrast, MH moderately suppressed the degree of secondary aerenchyma development, whereas PCIB strongly inhibited the development of not only phellogen and secondary aerenchyma but also stele (Figure and Table ). A high concentration of endogenous IAA is locoregional in the vascular cambial zone in the stems of woody plants, suggesting that auxin plays important roles in xylem development, such as cell division and xylem fiber expansion (Tuominen et al., Citation1997; Uggla et al., Citation1996). These results support the possibility that PCIB affects the stele development as an anti-auxin in soybean hypocotyls. In addition, root gravitropism was inhibited by auxin polar transport inhibitors (TIBA, NPA, and CHPAA) and an auxin antagonist (PCIB), while root hydrotropism is inhibited only by PCIB in A. thaliana seedlings, suggesting that the auxin response, apart from its polar transport, plays a definite role in the hydrotropic response (Kaneyasu et al., Citation2007). The results of that experiment on hydrotropic response for anti-auxins were similar to ours because auxin polar transport inhibitors (TIBA, NPA, HFCA, 1-NOA, and CHPAA) are supposed to be non-negative regulators of the process of secondary aerenchyma formation. However, the root hydrotropism in cucumber (Cucumis sativus) is restricted not only by PCIB but also by polar transport inhibitors (TIBA and HFCA) (as noted by Takahashi, Miyazawa, & Fujii, Citation2009), so the mode of anti-auxin responses depends on the plant species. Although MH and PCIB might be negative regulators of the process of secondary aerenchyma formation as anti-auxins, it is important to demonstrate the locoregional endogenous IAA in active phellogen as well as the IAA level in the hypocotyls under anti-auxins in order to clarify auxin’s involvement in secondary aerenchyma formation in soybean plants.
3.2. Endogenous IAA concentrations in the flooded hypocotyls
The endogenous IAA levels in the hypocotyls were quantified at several time points during 96 h after the onset of flooding (Figure ). There were no clear changes in endogenous IAA concentrations after flooding. At 24 and 48 h after the onset of flooding, the IAA concentration of the hypocotyls was slightly, not significantly, higher than that in the controls. The range of IAA concentrations was 26–34 ng g−1 fresh wt. in the controls, whereas it was 27–34 ng g−1 fresh wt. under flooding during the experiment, and we could not detect any significant difference between the control and flooding samples throughout the experiment.
Figure 3. Endogenous IAA concentrations in soybean hypocotyls after flooding. The hypocotyl sections were excised at 0, 24, 48, 72, and 96 h after flooding. Values are shown as means (±SE, n = 4–10). There is no statistical difference in IAA concentration between control and flooded plants at any sampling time as determined by Student’s t-test (p < 0.05).
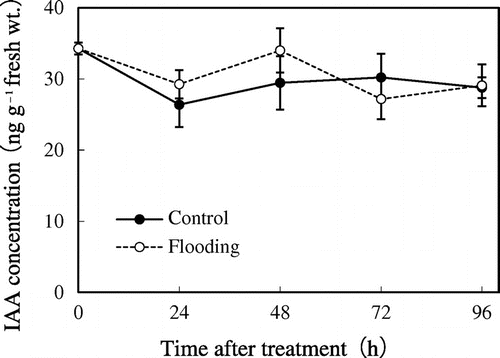
According to Vidoz, Loreti, Mensuali, Alpi, and Perata (Citation2010), ethylene entrapped in flooded tissues stimulates auxin transport and leads to auxin accumulation at the flooded hypocotyls of the tomato (Solanum lycopersicum); this induces the initiation of adventitious root primordia, after which adventitious roots develop. Similarly, in sunflower (Helianthus annuus) plants, adventitious root formation is stimulated by an accumulation of auxin, which might be induced by increase in ethylene, in hypocotyls under flooding (Wample & Reid, Citation1979). In soybean plants, exogenous auxins promote adventitious rooting from hypocotyls in cuttings from 7-d-old plants without root systems (Chen, Cheng, Chen, Yiu, & Liu, Citation2002; Chou, Huang, & Liu, Citation2010), and flooded stems and hypocotyls develop new adventitious roots easily (Bacanamwo & Purcell, Citation1999; Henshaw, Gilbert, Scholberg, & Sinclair, Citation2007; Shimamura et al., Citation2003; Thomas et al., Citation2005). However, no adventitious rooting was observed at the hypocotyl segments at 1 to 2 cm above the sand surface (see Figure B), suggesting no auxin accumulation for adventitious rooting in our experiments. Actually, the level of endogenous IAA in the hypocotyls of flooded soybean plants was not significantly different from that in control hypocotyls for 96 h after flooding (Figure ). In a similar time course, phellogen and secondary aerenchyma developed in flooded hypocotyls within 48 and 72 h after treatment, respectively (Shimamura et al., Citation2003, Citation2014). These results might indicate that the accumulation of auxin is not necessary to develop phellogen or secondary aerenchyma in flooded hypocotyls of soybean, though locoregional endogenous IAA should be considered.
4. Conclusion
The endogenous IAA concentration in the flooded hypocotyls of soybean plants did not increase or decrease until the secondary aerenchyma was observed. Several anti-auxins have no apparent effect on cell division in phellogen or cell elongation in aerenchymatous tissues, whereas MH and PCIB restricted aerenchyma development, suggesting that morphological responses are not common among anti-auxins. From the present results, it is difficult to conclude that auxin plays an important role in the process of secondary aerenchyma formation. Therefore, a demonstration of locoregional endogenous IAA in active phellogen and the IAA level in the hypocotyls under anti-auxins would clearly solve the problem.
Abbreviations
CHPAA, 3-chloro-4-hydroxyphenylacetic acid; PCIB, α-(p-chlorophenoxy) isobutyric acid; GC-SIM-MS, gas chromatography-selected ion monitoring-mass spectrometry; HFCA, 9-hydroxy-fluorene-9-carboxylic acid; IAA, indole-3-acetic acid; MH, maleic hydrazide; NAA, 1-naphthaleneacetic acid; 1-NOA, 1-naphthoxyacetic acid; NPA, N-1-naphthylphthalamic acid; TIBA, 2,3,5-triiodobenzoic acid.
Funding
This research was supported in part by a Grant-in-Aid for Young Scientists (B) (No. 17780017 to S.S.) from the Japan Society for the Promotion of Science.
Disclosure statement
No potential conflict of interest was reported by the authors.
References
- Bacanamwo, M., & Purcell, L. C. (1999). Soybean root morphological and anatomical traits associated with acclimation to flooding. Crop Science, 39, 143–149.10.2135/cropsci1999.0011183X003900010023x
- Campanoni, P., & Nick, P. (2005). Auxin-dependent cell division and cell elongation. 1-Naphthaleneacetic acid and 2,4-dichlorophenoxyacetic acid activate different pathways. Plant Physiology, 137, 939–948.10.1104/pp.104.053843
- Chen, L. M., Cheng, J. T., Chen, E. L., Yiu, T. J., & Liu, Z. H. (2002). Naphthaleneacetic acid suppresses peroxidase activity during the induction of adventitious roots in soybean hypocotyls. Journal of Plant Physiology, 159, 1349–1354.10.1078/S0176-1617(04)70364-8
- Chou, C. H., Huang, Y. C., & Liu, Z. H. (2010). Peroxidase genes differentially respond to auxin during the formation of adventitious roots in soybean hypocotyls. Plant Growth Regulation, 60, 151–161.10.1007/s10725-009-9431-7
- Fraser, L. (1931). The reaction of Viminaria denudata to increased water content of the soil. Proceedings of the Linnean Society of New South Wales, 56, 391–406.
- Galston, A. W. (1947). The effect of 2,3,5-triiodobenzoic acid on the growth and flowering of soybeans. American Journal of Botany, 34, 356–360.10.2307/2437695
- Gray, W. M., Östin, A., Sandberg, G., Romano, C. P., & Estelle, M. (1998). High temperature promotes auxin-mediated hypocotyl elongation in Arabidopsis. Proceedings of the National Academy of Sciences of the United States of American, 95, 7197–7202.10.1073/pnas.95.12.7197
- Hattori, R., Matsumura, A., Yamawaki, K., Tarui, A., & Daimon, H. (2013). Effects of flooding on arbuscular mycorrhizal colonization and root-nodule formation in different roots of soybeans. Agricultural Science, 4, 673–677.
- Henshaw, T. L., Gilbert, R. A., Scholberg, J. M. S., & Sinclair, T. R. (2007). Soya bean (Glycine max L. Merr.) genotype response to early-season flooding: I. Root and nodule development. Journal of Agronomy and Crop Science, 193, 177–188.10.1111/jac.2007.193.issue-3
- Jodinskienė, M., & Anisimovienė, N. (2007). Peculiarities of two different IAA binding sites functioning in kidney bean hypocotyl cell plasmalemma. Biologija, 53, 44–47.
- Justin, S. H. F. W., & Armstrong, W. (1987). The anatomical characteristics of roots and plant response to soil flooding. New Phytologist, 106, 465–495.10.1111/nph.1987.106.issue-3
- Kaneyasu, T., Kobayashi, A., Nakayama, M., Fujii, N., Takahashi, H., & Miyazawa, Y. (2007). Auxin response, but not its polar transport, plays a role in hydrotropism of Arabidopsis roots. Journal of Experimental Botany, 58, 1143–1150.10.1093/jxb/erl274
- Katekar, G. F., & Geissler, A. E. (1980). Auxin transport inhibitors: IV. Evidence of a common mode of action for a proposed class of auxin transport inhibitors: the phytotropins. Plant Physiology, 66, 1190–1195.10.1104/pp.66.6.1190
- Kawano, N., Kawano, T., & Lapeyrie, F. (2003). Inhibition of the indole-3-acetic acid-induced epinastic curvature in tobacco leaf strips by 2,4-dichlorophenoxyacetic acid. Annals of Botany, 91, 465–471.10.1093/aob/mcg043
- Laňková, M., Smith, R. S., Pešek, B., Kubeš, M., Zažímalová, E., Petrášek, J., & Hoyerová, K. (2010). Auxin influx inhibitors 1-NOA, 2-NOA, and CHPAA interfere with membrane dynamics in tobacco cells. Journal of Experimental Botany, 61, 3589–3598.
- Leopold, A. C., & Klein, W. H. (1951). Maleic hydrazide as an anti-auxin. Science, 114, 9–10.10.1126/science.114.2949.9
- Mattsson, J., Sung, Z. R., & Berleth, T. (1999). Responses of plant vascular systems to auxin transport inhibition. Development, 126, 2979–2991.
- Ni, D. A., Wang, L. J., Xu, Z. H., & Xia, Z. A. (1999). Foliar modifications induced by inhibition of polar transport of auxin. Cell Research, 9, 27–35.10.1038/sj.cr.7290003
- Oono, Y., Ooura, C., Rahman, A., Aspuria, E. T., Hayashi, K., Tanaka, A., & Uchimiya, H. (2003). p-Chlorophenoxyisobutyric acid impairs auxin response in Arabidopsis root. Plant Physiology, 133, 1135–1147.10.1104/pp.103.027847
- Ottenschläger, I., Wolff, P., Wolverton, C., Bhalerao, R. P., Sandberg, G., Ishikawa, H., … Palme, K. (2003). Gravity-regulated differential auxin transport from columella to lateral root cap cells. Proceedings of the National Academy of Sciences of the United States of American, 100, 2987–2991.10.1073/pnas.0437936100
- Parry, G., Delbarre, A., Marchant, A., Swarup, R., Napier, R., Perrot-Rechenmann, C., & Bennett, M. J. (2001). Novel auxin transport inhibitors phenocopy the auxin influx carrier mutation aux1. The Plant Journal, 25, 399–406.10.1046/j.1365-313x.2001.00970.x
- Rhine, M. D., Stevens, G., Shannon, G., Wrather, A., & Sleper, D. (2010). Yield and nutritional responses to waterlogging of soybean cultivars. Irrigation Science, 28, 135–142.10.1007/s00271-009-0168-x
- Samantarai, B., & Nanda, B. K. (1979). Evaluation of the role of hormonal factors in secondary growth of dicots. The Botanical Magazine Tokyo, 92, 13–22.10.1007/BF02488297
- Shimamura, S., Mochizuki, T., Nada, Y., & Fukuyama, M. (2002). Secondary aerenchyma formation and its relation to nitrogen fixation in root nodules of soybean plants (Glycine max) grown under flooded conditions. Plant Production Science, 5, 294–300.10.1626/pps.5.294
- Shimamura, S., Mochizuki, T., Nada, Y., & Fukuyama, M. (2003). Formation and function of secondary aerenchyma in hypocotyl, roots and nodules of soybean (Glycine max) under flooded conditions. Plant and Soil, 251, 351–359.10.1023/A:1023036720537
- Shimamura, S., Yamamoto, R., Nakamura, T., Shimada, S., & Komatsu, S. (2010). Stem hypertrophic lenticels and secondary aerenchyma enable oxygen transport to roots of soybean in flooded soil. Annals of Botany, 106, 277–284.10.1093/aob/mcq123
- Shimamura, S., Yoshioka, T., Yamamoto, R., Hiraga, S., Nakamura, T., Shimada, S., & Komatsu, S. (2014). Role of abscisic acid in flood-induced secondary aerenchyma formation in soybean (Glycine max) hypocotyls. Plant Production Science, 17, 131–137.10.1626/pps.17.131
- Smirnoff, N., & Crawford, R. M. M. (1983). Variation in the structure and response to flooding of root aerenchyma in some wetland plants. Annals of the Botany, 51, 237–249.
- Takahashi, H., Miyazawa, H., & Fujii, N. (2009). Hormonal interactions during root tropic growth: hydrotropism versus gravitropism. Plant Molecular Biology, 69, 489–502.10.1007/s11103-008-9438-x
- Thomas, A. L., Guerreiro, S. M. C., & Sodek, L. (2005). Aerenchyma formation and recovery from hypoxia of the flooded root system of nodulated soybean. Annals of Botany, 96, 1191–1198.10.1093/aob/mci272
- Tuominen, H., Puech, L., Fink, S., & Sundberg, B. (1997). A radial concentration gradient of indole-3-acetic acid is related to secondary xylem development in in hybrid aspen. Plant Physiology, 115, 577–585.
- Uggla, C., Moritz, T., Sandberg, G., & Sundberg, B. (1996). Auxin as a positional signal in pattern formation in plants. Proceedings of the National Academy of Sciences of the United States of American, 93, 9282–9286.10.1073/pnas.93.17.9282
- Vidoz, M. L., Loreti, E., Mensuali, A., Alpi, A., & Perata, P. (2010). Hormonal interplay during adventitious root formation in flooded tomato plants. The Plant Journal, 63, 551–562.10.1111/j.1365-313X.2010.04262.x
- Wample, R. L., & Reid, D. M. (1979). The role of endogenous auxin and ethylene in the formation of adventitious roots and hypocotyl hypertrophy in flooded sunflower plants (Helianthus annuus). Physiologia Plantarum, 45, 219–226.10.1111/ppl.1979.45.issue-2
- Zhao, Y., & Hasenstein, K. H. (2010). Physiological interactions of antiauxins with auxin in roots. Journal of Plant Physiology, 167, 879–884.10.1016/j.jplph.2010.01.012