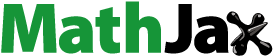
ABSTRACT
Inoculation with arbuscular mycorrhizal fungi (AMF) may enhance crop growth in upland fields, depending on the water regime. To quantify changes in AMF infection rate and subsequent effects on nutrient uptake and growth, we grew rice (wetland crop) and pearl millet (dryland crop) genotypes with or without the commercial inoculant Dr. Kinkon (Glomus sp. R10) in Andosol upland fields in 2020 and 2021. Root infection rates were measured in shallow (0–10 cm) and deep (20–30 cm) layers under three water regimes: well-irrigated, half-irrigated, and non-irrigated. Inoculation enhanced shoot dry weight (SDW), plant height, tiller number, phosphorus (P) uptake, leaf water potential, photosynthetic rate (measured only in 2021) and root transversal area. The increase in SDW with inoculation was higher under well-irrigated than under water-limited conditions. The increment in pearl millet SDW was related to higher P uptake associated with higher infection rates, whereas that in rice SDW was related to maintenance of leaf water potential, greater root transversal area and root length density, and higher P uptake but not to infection rate parameters. Inoculation increased mycorrhizal and vesicular infection rates with the similar tendency for arbuscular infection rate and qPCR. Infection rates were similar across water regimes in both years despite significant differences in plant growth parameters with higher rates in deep than shallow layers. AMF inoculation enhanced infection rates, mostly independent of water regime, but plant growth enhancement was greater under the well-irrigated treatment and was more directly linked with infection rates in pearl millet.
GRAPHICAL ABSTRACT
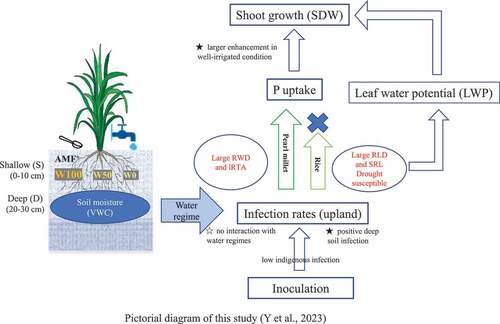
Introduction
Commercial arbuscular mycorrhizal fungal (AMF) inoculants are used for biological nutrient management of crops, but their contribution is much less certain than that of synthetic fertilizers (Salomon et al., Citation2022), and various agro-environmental conditions such as different water regimes may alter their effectiveness. Many studies have examined how water regimes in upland fields, from severe drought to well-irrigated conditions, significantly change the productivity of crops by altering plant growth and physio-morphological features in shoots and roots (e.g. Farooq et al., Citation2009). However, fewer studies have investigated how different water regimes affect the response to AMF inoculation and crop yields in upland fields (e.g. Al-Karaki et al., Citation2004; Sylvia et al., Citation1993).
AMF infect the plant root cortex and form branched structures called arbuscules that provide water and nutrients in exchange for carbon from host plants (Bonfante & Genre, Citation2010). Mycorrhizal infection enhances crop growth through increased uptake of phosphorus (P) (Chandrasekaran, Citation2020; Kobae, Citation2019). As AMF symbiosis may alter root morphological characteristics, such as root branching and thickness (Basyal & Emery, Citation2021; Vallino et al., Citation2014; Zou et al., Citation2017), and provide external hyphae that can better acquire water under drought than plant roots (Kakouridis et al., Citation2022; Zhang et al., Citation2018), crop water status and growth may be improved by inoculation of upland fields. However, the benefits of AMF inoculation may differ between well-irrigated and water-limiting conditions.
Although numerous pot experiments conducted in upland-like conditions showed improved infection under a lower water regime (Augé, Citation2001; Bahmani et al., Citation2018; Oliveira et al., Citation2022), other studies reported improved infection under a higher water regime (e.g. Al-Karaki et al., Citation2004; Karasawa et al., Citation2000) or unchanged infection across water regimes (e.g. Bethlenfalvay et al., Citation1988; Quiroga et al., Citation2017; Sylvia et al., Citation1993). Moreover, few field-level studies of infection response have been conducted (e.g. Sisaphaithong et al., Citation2017), but differences in conditions where soil water content and infection between surface (i.e. drier) and deeper soil layers (i.e. wetter) during drought have not been investigated.
Inoculation with AMF positively affected infection rate and plant growth in many crops (e.g. maize, wheat, barley, soybean, strawberry, and onion), mostly in controlled pot experiments (Augé, Citation2001; Bahadur et al., Citation2019; Begum et al., Citation2019). In rice, inoculation has neutral or positive effects on infection rate and growth under water-limiting conditions in pot experiments (Chareesri et al., Citation2020; Ruiz-Sánchez et al., Citation2010, Citation2021). In pearl millet, AMF inoculation positively affected shoot dry weight and plant water content under a low-water regime in a pot experiment (Fabbrin et al., Citation2015). To our knowledge, no field-level studies of pearl millet or rice have examined the interaction between inoculation and water regime in upland fields. Rice, a wetland crop, and pearl millet, a dryland crop with greater adaptation to low soil moisture (FAO, Citation2022), may show different responses to AMF inoculation across different water regimes and across soil depth profiles.
This study aimed at quantifying changes in AMF infection rates and the effectiveness of inoculation on rice and pearl millet under three different water regimes in upland fields. Specifically, we intended to show whether AMF inoculation to enhance infection rate could improve crop performance across different water regimes in upland fields. In addition, we investigated whether infection rates differed across soil depth profiles and whether crop growth is enhanced by inoculation regardless of water regime to assess interactions between inoculation and water regime.
Materials and methods
Two field experiments were conducted from July to November 2020 and from May to October 2021 in the No. 3NE upland fields at the Institute for Sustainable Agroecosystem Services, The University of Tokyo, Nishitokyo, Japan (35°43′N, 139°32′E). The fields were fallowed in 2019. The field has volcanic ash of the silty Kanto loam type (Humic Andosol) with dark humic silty topsoil (0–35 cm) and red-brown silty clay subsoil (below 35 cm) (Yamagishi et al., Citation2003). The soil chemical properties before planting in spring 2021 in the shallow (0–10 cm) and deep (20–30 cm) layers were, respectively, pH (H2O) 6.1 and 6.0, total C 5.2 and 4.0, total N 0.42 and 0.33, Truog P2O5 3.78 and 1.33, and K2O 30.0 and 9.0 mg/100 g soil.
Plant materials
Two genotypes (hybrid ‘Togo 4’, ‘Koshihikari’) of rice (Oryza sativa L.) and two (ICMB89111, ICMB95444) of pearl millet (Pennisetum glaucum (L.) R. Br.) were used. Koshihikari (KS) is an improved lowland genotype of O. sativa ssp. japonica and Togo 4 (TG) is a high-yielding, good-eating quality hybrid rice with a KS background, developed by the Research Institute of Rice Production & Technology Co., Ltd., Toyoake, Japan. ICMB89111 (891) is a high-tillering inbred genotype, and ICMB95444 (954) is a hybrid genotype, both of which were developed in the International Crop Research Institute for Semi-Arid Tropics (ICRISAT), Hyderabad, India. All four genotypes were used in 2020, but only TG and 891 were used in 2021.
Experimental design
In both years, seedlings were transplanted into the upland fields in a split-split-plot design with irrigation regime as the main plot, genotype as the sub-plot, and inoculation as the sub-sub-plot, with three replications. The main plots were separated from each other by space and Namiita buried by 15 cm depth to prevent water movement. A single plot measured 0.9 m × 0.9 m in 2020 with a main plot size 6.5 m2, a block size about 30 m2 and whole experimental area about 90 m2 inside a pipe house of 5 m × 18 m. The corresponding values in 2021 were 1.5 m × 0.9 m (a single plot), 5.4 m2 (a main plot), 30 m2 (a block), and 90 m2 (whole experimental area) using two smaller different pipe houses.
Growth conditions
In 2020, the seeds soaked in water were sown in nursery trays on 2 July, which was later than the conventional practice owing to pandemic restrictions. The seedlings were transplanted on 30–31 July at one plant per hill (45 cm × 15 cm) and 12 plants per plot. Time series of plant growth was illustrated in Fig. S1. Basal application of N-P-K fertilizer was 2-0-0 g/m2, lower than the conventional level. Weeds were controlled by hand regularly. The daily minimum and maximum air temperatures were 20.0 and 28.6°C, with daily solar radiation of 13.8 MJ/m2 and total rainfall of 421 mm from 30 July to 28 October (Fig. S2a,b).
In 2021, the seeds soaked in water were sown in nursery trays on 24 May (Fig. S1). The seedlings were transplanted into the field neighbouring the field used in the previous year on 25 June at one plant per hill (30 cm × 15 for rice and 30 cm × 30 cm for pearl millet), with 30 plants per plot for rice and 15 for pearl millet. Fields were topdressed with a basal N-P-K fertilizer, using an amount (6-4.5-8 g/m2) that would provide only 50% of the P used in conventional practice. Weeds were controlled by hand regularly. The daily minimum and maximum air temperatures were 18.8 and 26.8°C, with daily solar radiation of 13.2 MJ/m2 and total rainfall of 592 mm from 30 July to 28 October (Fig. S2c,d).
Inoculation treatment
In the inoculation treatment (I), the commercial inoculant Dr. Kinkon (Glomus sp. R10) (Idemitsu Kosan Co., Ltd., Tokyo, Japan), was added both at sowing and at transplanting (Fig. S1). Before sowing, it was mixed into autoclaved nursery soil at 10 g/kg soil in 2020 and 7 g/kg soil in 2021. Seedlings were transplanted after three weeks. Before transplanting, the inoculant was placed in a small hole of ca. 10 cm depth made with a shovel below the hill position, at 2 g/hill (~30 g/m2 in 2020; ~44 g/m2 in rice and 22 g/m2 in pearl millet in 2021). Seedlings were checked for mycorrhizal infection before transplanting; the infection rate was 0% in 2020 and ~ 4% in 2021 (n = 4). No inoculant was added in the control treatment (C).
Water treatments
The field was maintained in a well-watered condition by using supplementary irrigation in the first five weeks after transplanting. The water treatments were imposed from 8 August to 15 September 2020 (38 days) and from 30 July to 7 September 2021 (39 days) (Fig. S1). The whole field was covered with plastic film during the treatment to exclude rainfall. We applied three irrigation regimes: well-irrigated (W100), half-irrigated (W50), and non-irrigated (W0). Fields were watered by hand every two days with a hose and a hand-held garden sprayer. W100 fields received 7 L/min for about 1.5 min per plot in 2020 and 2.5 min per plot in 2021; W50 fields received the same volume for half the time. The irrigation time for W100 was flexibly changed depending on the weather on the basis of general assumptions of daily crop evapotranspirational demand (Allen et al., Citation1998). A set of SM150 soil moisture sensors (Delta-T Devices Ltd., Cambridge, UK) were inserted in rice plots of each water regime at depths of around 5 and 25 cm, respectively (in one block only), to measure the soil moisture in the shallow (0–10 cm) and deep layers (20–30 cm). The soil moisture values were converted to soil volumetric water content (VWC, %) by making a calibration curve. In 2020, the surface soil moisture of all plots was monitored with a HydraGo portable SM sensor probe (7 cm; Stevens Water Monitoring Systems Inc., Portland, USA). In 2020, soil VWC in the shallow layer was ca. 53% before the water treatments were started and ca. 50% in W100, 38% in W50, and 28% in W0 at the end of the treatments; and that in the deep layer was ca. 51% before the treatments and 43% in W100, 36% in W50, and 27% in W0 after treatment (Fig. S3). During the treatments, the average VWC of the shallow and deep layers was 50% and 48% in W100, 41% and 41% in W50, and 32% and 36% in W0. In 2021, soil VWC in the shallow layer was ca. 40% before treatment and ca. 38% in W100, 30% in W50, and 25% in W0 after treatment; and that in the deep layer was ca. 49% before treatment and 53% in W100, 47% in W50 and 35% in W0 after treatment. During the treatments, the average VWC of the shallow and deep layers was 41% and 50% in W100, 30% and 46% in W50, and 27% and 37% in W0.
Measurements
Shoot growth parameters
Tiller number (TN), plant height (PH, cm), and shoot dry weight (SDW, g/plant) were measured in 1–3 rice plants and 1–2 millet plants in each plot in 2020 and 1–2 plants of each in 2021 at the end of the water treatment on 15–18 September 2020 and 9 September 2021. Measurements of both genotypes were taken at around the flowering stage in both years. Midday leaf water potential (LWP, MPa), as an indicator of plant water status, was measured in the flag leaf (entire leaf blade) from four plants with a Model 600-EXP pressure chamber (PMS Instrument Company, Albany, USA) from late morning to early afternoon on 17–18 September 2020 and 9 September 2021. In 2021, the photosynthetic rate (Pn, μmol/m2s) was also measured on the flag leaf from three plants at around noon on 30 August with a portable photosynthesis system LI6400 (LI-COR, Lincoln, USA). The reference CO2 concentration was set at 400 μmol CO2/mol and matched with the sample CO2 concentration every 30 min. Relative humidity was adjusted to 60%–80%, block temperature to 30–32°C, and light source to 1500 μmol/m2s.
Shoot P concentration and uptake
For P measurements, a whole shoot was ground into powder in a fine mill (Heiko sample mill, TI 300, Fujiwara Seisakusho, Ltd., Tokyo, Japan) and digested in a MARS 6 microwave acid digestion system (CEM, Matthews, NC, USA) using a modified method for plant tissue (www.cem.com). Each sample (~0.1 g powder) was digested in 5 mL of 68% HNO3 +1 mL of 30% H2O2 at 220°C for 60 min. After cooling for 20–30 min, the solution was transferred to a 10-mL tube by rinsing twice with 2 mL of distilled water before filling up 10 mL. Next, the solution was mixed and filtered by a Minisart syringe filter with 0.22 µm pore size (Minisart RC, Sartorius, Germany) up to 6 mL. Standard solutions were prepared as 0, 10, and 50 ppm in three separate 50-mL tubes. All concentrations were then calibrated and measured by inductively coupled plasma-optical emission spectrometry (ICP-OES; SPS3000, Hitachi High-Technologies, Tokyo, Japan). Finally, the measured concentration and dry weight were used to calculate P uptake (mg/plant).
Root growth parameters
Roots down to 60 cm were collected in a linear core sampler (5 cm diameter, 30 cm length; DIK-s110C, Daiki Rika Kogyo Co., Saitama, Japan) around the flowering stage on 15–29 September in 2020 and 7–19 September in 2021. Three cores (one per plot) were taken ~5 cm from the plant base along the row. The collected cores were stored at 4°C before they were cut into six 10-cm-long segments and the roots were separated from the soil by hand. The root pieces were washed with tap water and arranged on a transparent plate to be scanned with an Epson Expression 11000XL scanner set in professional mode, positive film, and 8-bit grayscale at 600 dpi (Nguyen et al., Citation2022). Images were analysed in WinRhizo Pro software (Regent Instruments, Quebec, Canada) to estimate total root length, and root length density (RLD, cm/cm3) was calculated by dividing with the collected core volume. In 2020, roots from rice (C, I) and pearl millet (I) were collected; roots from pearl millet (C) were not collected owing to a minor technical problem. The scanned roots were immersed in 50% ethanol for the following root and infection rate measurements.
One root per replication was taken from the stored samples from the 0–10- and 20–30-cm cores and cross-sectioned for observation under a phase-contrast microscope (BX51, Olympus, Hicksville, NY, USA), and images were captured in CellSens standard software (Olympus). Root transverse area (RTA, µm2) was measured by using the polygon tool in ImageJ v. 1.51t software (NIH, Bethesda, MD, USA) (Y et al., Citation2020).
In 2021, additional roots were collected at ~5 cm from the plant base, along the row, down to 30 cm depth with a Mascaro Soil Profile Sampler (30.5 cm deep × 7.6 cm wide × 1.2 cm thick; Turf-Tec International, Tallahassee, FL, USA) on 20 September 2021. The samples were divided into three segments, each 10 cm long, by soil depth. Roots from each segment were washed and oven-dried at 80°C for 3 days; dry weight and root weight density (RWD, mg/cm3) were calculated by dividing by the volume. Specific root length (SRL, cm/mg) was calculated as RLD/RWD for each depth. The sum of the RWD down to 30 cm depth was extrapolated per hill area (i.e. 15 cm × 30 cm in rice, 30 cm × 30 cm in pearl millet) to estimate root dry weight (RDW, g/plant). Root-to-shoot weight ratio (RS) was calculated as RDW/SDW.
Root AMF infection by microscopic observation
The trypan blue method was used for microscopic observation of AMF infection (Phillips & Hayman, Citation1970). In 2020, the remaining roots from the core samples (0.4–0.8 mm diameter), stored immersed in 50% ethanol, were used. In 2021, 7–10 pieces of ~ 5-cm roots in the shallow (0–10 cm) and deep (20–30 cm) layers were randomly collected by trenching, washed, and immediately immersed in hand gel with 70% ethanol (TOA Industry, Co., Ltd., Tokyo, Japan) before staining. Roots (5–10 pieces of ~ 2-cm roots) were cleared with 10% (w/v) KOH by boiling at 110°C for 15 min, rinsed once with water, soaked in 2% (w/v) HCl at room temperature for about 5 min, and dyed with trypan blue solution (0.05% in lactic acid) by boiling at 90°C for 5–10 min. After rinsing three times with water, roots were stored in lactoglycerol with a ratio of lactic acid : glycerol : water = 8:1:1 (v/v/v).
The AMF-infected roots were then quantified with a modified gridline intersection method (Giovannetti & Mosse, Citation1980). In brief, three roots per replication were placed in parallel on a 1-mm × 1-mm micro slide glass with a cover glass and observed at 100× or 200× magnification under a phase-contrast microscope (BX51, Olympus). In total, nine segments of 2-cm root per three replications, ~180 intersections per treatment, were observed. Each intersection was scored for the presence or absence of AMF structures: arbuscules, vesicles, and intraradical hyphae (Fig. S4). Mycorrhizal infection rate (or intraradical hyphal infection rate, M%) was calculated for each sample by dividing the total number of infected root intersections by the total number of observed root intersections (n ≈60). Arbuscular (A%) and vesicular (V%) infection rates were determined by dividing the number of arbuscule- and vesicle-positive intersections by the total number of observed root intersections, as:
Assessment of root AMF infection by quantitative PCR
For quantitative real-time PCR (qPCR), root samples from another core sampling from only control plots (C) of the two genotypes 891 and TG from both shallow (0–10 cm) and deep (20–30 cm) layers on 3 October 2020 were used. In 2021, roots were collected from plants of the same two genotypes, grown in both control (C) and inoculated (I) plots, by trenching. In both years, the collected root samples were washed, wiped, and freshly weighed before ~100 mg was stored in a 5-mL tube in a deep freezer at − 30°C.
The freeze-dried root samples were ground in 2-mL tubes with a metal ball at 1200 rpm for 3 min at around 25°C in a ShakeMaster cell disruption device (Bio Medical Science Inc., Tokyo, Japan), and DNA was extracted from ~10 mg freeze-dried roots with a DNeasy Plant Mini Kit (Qiagen, Redwood, CA, USA) following the manufacturer’s instructions. After extraction, the DNA concentration was determined on a NanoDrop One spectrophotometer (Thermo Fisher Scientific, Waltham, MA, USA) and diluted to 10–20 ng/µL. Primers to amplify 450 bp of the 18S SSU rRNA gene AMF were used: forward primer AMF4.5NF (5′-AAGCTCGTAGTTGAATTTCG-3′) and reverse primer AMVR (5′-CTGACAATTGAATACTAATGCC-3′) (Morimoto et al., Citation2018; Sato et al., Citation2005; Van Geel et al., Citation2014). The primer set AMV4.5NF-AMDGR, first introduced by Sato et al. (Citation2005), successfully amplified rDNA from diverse arbuscular mycorrhizal (AM) fungal species, including the previously challenging to amplify Archaeosporaceae. Subsequent studies, such as those by Van Geel et al. (Citation2014) and Morimoto et al. (Citation2018), affirmed its effectiveness, revealing its superior AMF DNA amplification quality and enhanced specificity through the revised reverse primer AMVR, thereby solidifying the choice of AMV4.5NF-AMVR for quantifying the AMF population.
The reaction was performed using SsoAdvanced Universal SYBR Green Supermix Assay (Bio-Rad, Hercules, CA, USA) on a QuantStudio 3 Real-Time PCR system (Thermo Fisher Scientific, Waltham, MA, USA) in 20 µL containing 10 µL of 2× Premix, 0.4 µL of ROX Dye II, 0.8 µL of each primer (10 µM), 7 µL of sterilized distilled water, and 1 µL of template DNA. PCR conditions used an initial denaturation at 94°C for 2 min, followed by 40 cycles of denaturation at 94°C for 15 s, annealing at 56°C for 30 s, and extension at 60°C for 30 s. The amplification products were evaluated against a standard curve. The rDNA region of Gigaspora margarita strain C (MAFF520054) covering 18S, ITS1 and 2, 5.8S, and the head of 28S was PCR amplified and cloned into the pTA2 vector, with the amplified fragment’s sequence confirmed as homologous to the reported G. margarita sequence. Following propagation, purification, and linearization of the final construct, pTA2-C31 (6244 bp), its concentration was quantified, converted to copies/µL considering its molecular weight (3858 kDa), and used as a qPCR standard after proper dilution. Finally, the amplicon quantity was divided by the DNA template concentration to determine copy number perng (copies/ng).
Statistical analysis
The combined analysis of the two years was done for the common genotypes (891, TG) for the effects of water regime (W100, W50, W0) and inoculation (C, I), and their interactions on shoot growth parameters (SDW, PH, TN, Pn, LWP), P uptake and RS, based on the split-split plot design in GenStat 21st edition software (VSNi, Hemel Hempstead, UK). Soil depth (0–10 cm, 20–30 cm) was included in the ANOVA of root growth parameters (RLD, RWD, RDW, RTA, SRL) and infection rates (M%, A%, V%, qPCR). Multiple comparisons by Tukey’s test (significance set at P < 0.05) were performed. Separate analysis of each year was also conducted, including the two additional genotypes only used in 2020 (954, KS), as shown in Tables S1- 3. In the separate analysis of 2020, as the root parameters and infection rates of pearl millet genotypes (891, 954) were missed harvesting due to unexpected technical problem, unbalanced ANOVA with multiple comparisons (Bonferroni’s test) had to be used.
Results
Shoot growth and P uptake
Overall, inoculation, water regime, genotype, and year affected shoot growth parameters (SDW, PH, TN, LWP, Pn) and P uptake (, S1). Inoculation increased SDW by 36% (44% in 2020 and 24% in 2021), and P uptake by 50% (39% and 61%, respectively). Plant P concentration in 2021 was 0.121% in control plots and 0.148% in inoculated plots, and 0.172% for pearl millet and 0.096% for rice, respectively (data not shown). Pearl millet had higher photosynthetic rate (in 2021) and higher midday LWP and SDW than rice. SDW was smaller in 2020 than 2021 due to late transplanting during the pandemic and less fertilization. Inoculation × genotype (I × G) with P < 0.001 and inoculation × water regime (I × W) with P < 0.05 were significant both for SDW and P uptake (); inoculation effects were larger in pearl millet (SDW from 64.2 g to 89.0 g, P uptake from 98.1 mg to 171.0 mg) than rice (SDW from 24.3 g to 26.7 g and P uptake from 23.4 mg to 27.3 mg), and also largest in W100 (SDW from 48.6 g to 69.1 g, P uptake from 71.0 mg to 109.7 mg), followed by W50 (SDW from 43.1 g to 58.0 g, P uptake from 53.4 mg to 103.1 mg) and least in W0 (SDW from 40.9 g to 46.6 g, P uptake from 57.8 mg to 84.6 mg). A decline in LWP led to a decline in SDW in rice ().
Figure 1. Relationship between midday leaf water potential (LWP) and shoot dry weight (SDW) in pearl millet (891, ICMB89111) and rice (TG, Togo 4) with/without inoculation (I, C) under three irrigation regimes (W100, well-irrigated; W50, half-irrigated; W0, non-irrigated) in 2021. Bars show standard errors. Linear regression was shown where significant at P ≤0.05.
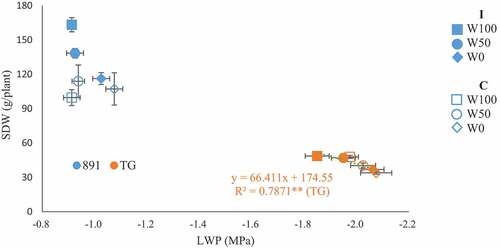
Table 1. Shoot dry weight (SDW), plant height (PH), tiller number (TN), leaf water potential (LWP), photosynthetic rate (Pn), and phosphorous uptake (P uptake) in control (C) and inoculated (I) plants under well-irrigated (W100), half-irrigated (W50), and non-irrigated (W0) conditions in pearl millet (891, ICMB89111) and rice (TG, Togo 4) across 2020 and 2021. Significant effects of inoculation (I), water regime (W), genotype (G) and year (Y) as well as their interactions by four-way ANOVA were also shown.
Root growth
Inoculation enhanced only RTA in both years (, S2). Water regime, genotype, depth and year also affected numbers of root growth parameters (RLD, RWD, SRL, RTA, RDW) except for RS. Pearl millet and rice had contrasting root traits: RWD, RDW, and RTA were higher in pearl millet, and RLD and SRL were higher in rice. Rice had higher RLD in the shallow soil than pearl millet, with significant genotype × depth interactions (G × D) in both years. Water regime × depth interaction showed RLD in shallow layer was largest under W100, followed by under W50, and smallest under W0.
Table 2. Root length density (RLD, cm/cm3), root weight density (RWD, mg/cm3), specific root length (SRL, cm/mg), root transversal area (RTA, m2) at depth (D; 0–10 vs. 20–30 cm) in control (C) and inoculated (I) plants under well-irrigated (W100), half-irrigated (W50), and non-irrigated (W0) conditions in pearl millet (891, ICMB89111) and rice (TG, Togo 4) across 2020 and 2021. Root dry weight (RDW, g/plant) and root-to-shoot weight ratio (RS) in 2021 were also shown without the effect of D. Significant effects of inoculation (I), water regime (W), genotype (G), depth (D), and year (Y) as well as their interactions by five-way ANOVA were also shown.
Root AMF infection
Overall, inoculation, depth and year affected the infection rates (M%, A%, V% and qPCR) (). Inoculation on average of the two years enhanced M% (from 30% to 38%) and V% (from 2.1% to 4.3%), and tended to enhance A% (from 7.5% to 10.8%) and qPCR (831 to 1304 copies/ng) and the separate analysis showed significant increase only in 2021 with M% (from 16% to 33%) and V% (from 1.9% to 5.5%) (cf. Table S3). Infection rates (M%, A%, qPCR) were higher in 2020 than in 2021. Water regime and genotype did not change root AMF infection rates according to either microscopic observation (M%, A%, V%) or molecular quantification (qPCR) (, S3). Deep roots had higher all the infection rates than shallow roots. In 2021, rice had higher M% in deep layer than shallow layer (), as shown by the effect of the genotype × depth interaction (G × D) on M% (Tables S3). Inoculated pearl millet in W100 and inoculated rice in W0 tended to have higher V% in the deep layer (), as shown by the effect of the I × W × G × D interaction on V% (Table S3).
Figure 2. Genotype by depth two-way interaction (G × D) effect on M% (a) and four-way interaction (I × W × G × D) effect on V% (b) in 2021 for pearl millet (891, ICMB89111) and rice (TG, Togo 4) in the shallow (0–10 cm) and deep layers (20–30 cm). To simplify, fig (b) showed only inoculation (I) across the three water regimes (W100, well-irrigated; W50, half-irrigated; W0, non-irrigated). Bars show standard errors, and columns marked with different letters are significantly different at P < 0.05.
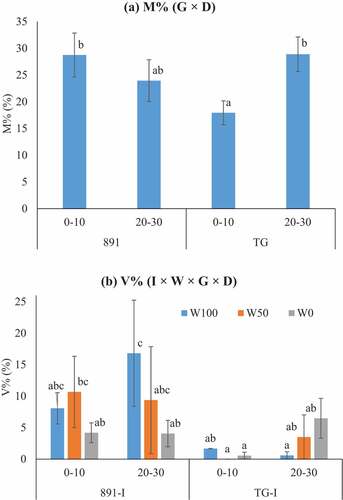
Table 3. Mycorrhizal infection rate (M%, %), arbuscular infection rate (A%, %), vesicular infection rate (V%, %), and quantitative PCR (qPCR, copies/ng) at depth (D; 0–10 vs. 20–30 cm) in control (C) and inoculated (I) plants under well-irrigated (W100), half-irrigated (W50), and non-irrigated (W0) conditions in pearl millet (891, ICMB89111) and rice (TG, Togo 4) across 2020 and 2021. Significant effects of inoculation (I), water regime (W), genotype (G), depth (D), and year (Y) as well as their interactions by five-way ANOVA were also shown.
Relationships among shoot, nutrient uptake, root, and infection rate parameters
In 2021, significant differences in SDW between pearl millet and rice (n = 12) were correlated positively with LWP, shoot P uptake, RTA, RWD and V%, but negatively with RLD and SRL (Table S4). SDW in pearl millet (n = 6) was correlated positively with P uptake, RTA, M% and V%. SDW in rice (n = 6) was correlated positively with LWP, P uptake, RTA and RLD in rice, but not with any of the infection rates. SDW of pearl millet was enhanced by the enhanced P uptake provided by inoculation, especially in W100 plots, whereas SDW and P uptake of rice were lowest under W0 in 2021 (). P uptake was enhanced by the higher M% provided by inoculation in pearl millet, but not in rice (). P uptake in rice tended to be increased by higher RLD () and significantly reduced by declining LWP ().
Figure 3. Relationship between P uptake and shoot dry weight (SDW) (a), mycorrhizal infection rate (M%) and P uptake (b), root length density (RLD) and P uptake (c) and leaf water potential (LWP) and P uptake (d) in 2021 for pearl millet (891, ICMB89111) and rice (TG, Togo 4) with/without inoculation (I, C) across three irrigation levels (W100, well-irrigated; W50, half-irrigated; W0, non-irrigated) in the shallow (0–10 cm) and deep layers (20–30 cm). Bars show standard errors. Linear regressions were shown for both crops (a), for pearl millet 891 (b), for rice TG (c, d) when significant at P ≤ 0.10. +, * indicated significance at P = 0.10 and 0.05, respectively.
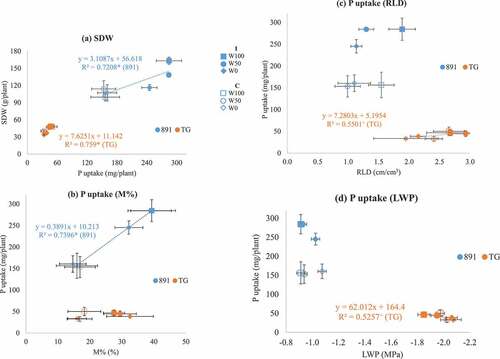
Discussion
Inoculation effects on plant growth in rice and pearl millet
In an Andosol upland field with limited input of P fertilizer, inoculation with Glomus sp. R10 consistently enhanced SDW and other shoot growth parameters of both rice and pearl millet around flowering time in both years (, S1), confirming its potential utility. Enhancement of plant growth by this commercial inoculant has been reported in maize in pot (Karasawa et al., Citation2000) and soybean in long-term bare fallow fields (Hayashi et al., Citation2018). Here, we showed that in rice and pearl millet grown in upland fields, the positive effect of inoculation on SDW was stronger in pearl millet (I × G), and also in well-irrigated conditions (W100) (I × W; , I × W × G in 2021 in Table S1). A similar positive influence of water treatment and inoculation on biomass production was shown in one year in field-grown maize (Sylvia et al., Citation1993), although numerous other studies indicate that inoculation has a greater effect on biomass production under water-limiting conditions in both pot and field environments (Augé, Citation2001; Karasawa et al., Citation2000; Sylvia et al., Citation1993). In our study, inoculation was associated with a larger SDW gain under W100 than under water-limiting conditions; our conditions were also P-limiting because we applied only a half dose (45 kg P/ha) of fertilizer. The higher level of shoot assimilate available to roots and greater P demand in shoots may have increased the need for AMF-mediated acquisition of nutrients. In reports that did not show such a large growth increment under well-watered conditions, the P level appeared sufficient, and AMF-mediated P acquisition was not promoted (e.g. Karasawa et al., Citation2000). In a review, Augé (Citation2001) stated that field-level long-term drought would cause P deficiency and increase AMF infection and AMF-mediated P uptake in lower-water regimes. We did not observe this here, for unknown reasons, but in rice, it may be due to the low plant water status limiting the supply of assimilate to roots, thus discouraging AMF infection.
Strong positive correlations among infection rates (i.e. M% and V% in 2021), P uptake, and SDW in pearl millet (Fig., 3a,b, Table S1) indicated AMF-mediated P acquisition (mycorrhizal pathway), whereas rice tended to acquire P directly (direct pathway) via its thinner and more developed surface root system (i.e. higher RLD) (Smith et al., Citation2011). Isobe and Tsuboki (Citation1998) compared the responses of grass and legume species to AMF infection and showed less dependency upon AMF symbiosis in crops with more extensive root systems. Here, rice developed more extensive thinner and branched roots in the shallow layer than pearl millet, indicated by its higher RLD (, S2); these shallow roots may have directly taken up P without using the AMF-mediated pathway (). In addition, rice is less adapted to upland fields and more susceptible to water deficit than pearl millet, as was clear from its reduction in LWP and SDW under W0 (), which may have limited the assimilate supplied by rice to the AMF (Konvalinková et al., Citation2015; Wang et al., Citation2021).
Inoculation also increased LWP (), which may have been associated with the increment of RTA () through higher root hydraulic conductivity (Table S4). For example, trifoliate oranges inoculated with Diversispora versiformis and grown in pots under with limited water for 6 weeks had increased root diameter, which was positively associated with LWP and levels of hormones such as auxin (Zou et al., Citation2017). Inoculation also increased root diameter in controlled or pot studies in switchgrass (Panicum virgatum), big bluestem (Andropogon gerardii Vitroari) and okra (Abelmoschus esculentus) (Basyal & Emery, Citation2021; Hetrick et al., Citation1988; Jabborova et al., Citation2021).
This study assessed the positive effect of the single AMF inoculant, Glomus sp. R10, on rice and pearl millet under three different water regimes (, ), but there is substantial literature indicating the differential abilities of AMF species in P uptake, within the context of the AMF communities in the agroecosystems (Kobae, Citation2019; Marro et al., Citation2022). In 2021, the metagenomic analysis showed that W0 tended to have a higher abundance of three operational taxonomic units or OTUs (131_Cla_AB812592, 137_Cla_AB665521, 482_Cla_LC416131) of genus Claroideoglomus, whereas W100 increased the abundance of two OTUs of Acaulospora (185_Aca_FR750156) and Diversispora (206_Div_AB640737) (P, Citation2023). Members of Claroideoglomus were reported to increase stomatal conductance under drought in a meta-analysis of 465 studies (Augé et al., Citation2015), while members of Acualospora like Acaulospora laevis and Diversipora like Diversipora spurca benefited both P nutrition and biomass (Marro et al., Citation2022). It is also important to note the complex interactions between multiple AMF species in field conditions, including the inoculant interaction with indigenous AMF species (Verbruggen et al., Citation2013). Future research should better clarify the effects of inoculation with relation to AMF species composition under different water regimes.
Effects of inoculation on infection rates across water regimes
Inoculation increased infection rates (especially M%, V% in 2021) regardless of water regime (, S3). Karasawa et al. (Citation2000) reported a slightly greater enhancement of infection rate in maize in well-watered pots than in drier pots. Conversely, Chareesri et al. (Citation2020) found a greater enhancement of infection rate in rice in drier pots than in near-saturated pots. Because the water regime in upland fields may vary more slowly and less uniformly than that in pot experiments (cf. Fig. S3), the actual AMF infection response may become similar across water regimes in upland fields. As rice and pearl millet are differently adapted to soil drying, it is surprising that they did not show dramatic differences in infection rates in response to different water regimes (, ) (although shoot growth performance did differ, as mentioned in the previous section). We compared only a few genotypes of each crop, and the AMF infection rate should be further assessed by using a wider range of genetic materials; in particular, the AMF infection rate of pearl millet has scarcely been studied.
AMF infection has been shown to vary greatly depending on multiple factors, including the species of AMF, water availability, plant host, and phenology (Augé, Citation2001). The decision to collect root samples around the flowering stage in this study was based on earlier studies suggesting this period to be critical for nutrient uptake and allocation (Al-Karaki et al., Citation2004, Niwa et al., Citation2018; Watanarojanaporn et al., Citation2013). There is the possibility of certain species of AMF inoculant developing at similar or different rates, with some exhibiting beneficial effects earlier, while others show such effects later (cf., Glomus mosseae vs Glomus etunicatum in Al-Karaki et al., Citation2004). In this study, Rhizophagus irregularis Glomus sp. R10 was used due to its positive infection and beneficial effects at the flowering stage in the field (Hayashi et al., Citation2018; Niwa et al., Citation2018). Niwa et al. (Citation2018) reported that R10 had typical ruderal traits, which could have an early infection with mass spore production and well-adaptation to agricultural land. There was also evidence that R10 showed an infection rate at the vegetative stage (Karasawa et al., Citation2000), but its infection dynamism across stages has never been observed in the field. Infection rates (either M% or V%) were higher in the deeper soil layer than in the shallower layer (, S3), where the initial inoculants were placed. The higher infection rate in the deeper layer may have resulted from (1) the movement of spores of Glomus sp. R10 downward from the shallower layer their entry into the roots in the deeper layer, (2) the entry of hyphae into roots in the shallower layer and proliferating into roots in the deeper layer, and (3) infection of the deeper roots by indigenous AMF spores present in the deeper layer. Alternatively, the lower P level in the deeper soil layer may have promoted AMF infection there (Rillig & Field, Citation2003). Infection rates in the deeper layer also showed interactions with genotype (i.e. for M%, particularly in 2021, A%, V%; , S3) and with water regime and inoculation (i.e. W x D for M%; , I x W x G x D for V% in 2021; Table S3, ). The soil volumetric water content at 0–10 cm in W0 in 2020 (Fig. S3) was considered to be close to the wilting point of rice (e.g. Kato et al., Citation2006); this may have shifted AMF infection in rice to the deeper profile with more moisture, which was confirmed by the core sampling (data not shown). Recently, the subsoil layer, typically below 20–30 cm depth, was found to have more abundant and unique AMF communities (Luo et al., Citation2021; Oehl et al., Citation2005; Sosa-Hernández et al., Citation2018), which may assist host plants to acquire deep nutrients and water (Sosa-Hernández et al., Citation2018, Citation2019). The capacity of AMF-mediated acquisition of water and nutrients from the deeper soil to ameliorate the effects of surface drought is still unknown, since the amount of roots generally decreases with depth, and the proportion of deep roots infected with AMF was not measured in this study. These aspects need further investigation.
Infection rates across irrigation levels
Water and inoculation conditions significantly affected plant growth, including both shoot and root traits; growth effects were greater in rice than in pearl millet, but the infection rates by both microscopic observation (M%, A%, V%) and molecular quantification (qPCR) were similar in both crop species in both years (, S3), which is a unique finding of this study. Pot studies in rice showed higher infection rates under water-limiting than well-watered conditions (Chareesri et al., Citation2020; Ruiz-Sánchez et al., Citation2010, Citation2021), but scaling up from pots to fields and allowing plants to grow for longer periods (~40 days of water treatment) may mitigate the water conditions, enabling plants and AMF to form symbioses similarly. Another possibility is that the total amounts of infected roots (not quantified here or in most studies) could have been different by water regime; for example, the amounts of infected roots could be larger under water-limiting conditions if roots in contact with drier soils invite more AMF infection. Alternatively, it could be larger under well-watered conditions if assimilate supply from shoots dominantly drives AMF infection belowground. This aspect should be further investigated. Some studies reported small changes in AMF composition under drought (Akyol et al., Citation2019; Müller et al., Citation2019). If AMF species adapted to drier soil can be identified, they might be used to enhance crop growth if they continue to be supplied with assimilate by the host, even under drought. It may be fruitful to study AMF inoculation among drought-adapted germplasms of various crop species, as they may be able to maintain biomass production or alter biomass partitioning to roots during drought.
Supplemental Material
Download (1 MB)Acknowledgments
We thank Associate Professor Daisuke Tsugama (The University of Tokyo, Japan) and Dr. D.K. Gupta (ICRISAT, India) for providing pearl millet seeds, and Dr. K. Jinushi (Research Institute of Rice Production & Technology Co., Ltd., Japan) for providing Togo 4 hybrid rice seeds. We also thank the technical staff of the Institute for Sustainable Agroecosystem Services, The University of Tokyo, for preparing fields and for providing weather data. This study was supported by KAKENHI C (20K05995).
Disclosure statement
No potential conflict of interest was reported by the author(s).
Supplemental material
Supplemental data for this article can be accessed online at https://doi.org/10.1080/1343943X.2023.2251181
Additional information
Funding
References
- Akyol, T. Y., Niwa, R., Hirakawa, H., Maruyama, H., Sato, T., Suzuki, T., Fukunaga, A., Sato, T., Yoshida, S., Tawaraya, K., Saito, M., Ezawa, T., & Sato, S. (2019). Impact of introduction of arbuscular mycorrhizal fungi on the root microbial community in agricultural fields. Microbes and Environments, 34(1), 23–32. https://doi.org/10.1264/JSME2.ME18109
- Al-Karaki, G., McMichael, B., & Zak, J. (2004). Field response of wheat to arbuscular mycorrhizal fungi and drought stress. Mycorrhiza, 14(4), 263–269. https://doi.org/10.1007/s00572-003-0265-2
- Allen, R. G., Pereira, L. S., Raes, D., & Smith, M. (1998). Crop evapotranspiration-Guidelines for computing crop water requirements-FAO irrigation and drainage paper 56. FAO. https://www.fao.org/3/r4082e/r4082e00.htm#Contents
- Augé, R. M. (2001). Water relations, drought and vesicular-arbuscular mycorrhizal symbiosis. Mycorrhiza, 11(1), 3–42. https://doi.org/10.1007/s005720100097
- Augé, R. M., Toler, H. D., & Saxton, A. M. (2015). Arbuscular mycorrhizal symbiosis alters stomatal conductance of host plants more under drought than under amply watered conditions: A meta-analysis. Mycorrhiza, 25(1), 13–24. https://doi.org/10.1007/s00572-014-0585-4
- Bahadur, A., Batool, A., Nasir, F., Jiang, S., Mingsen, Q., Zhang, Q., Pan, J., Liu, Y., & Feng, H. (2019). Mechanistic insights into arbuscular mycorrhizal fungi-mediated drought stress tolerance in plants. International Journal of Molecular Sciences, 20(17), 4199. https://doi.org/10.3390/ijms20174199
- Bahmani, M., Naghdi, R., & Kartoolinejad, D. (2018). Milkweed seedlings tolerance against water stress: Comparison of inoculations with Rhizophagus irregularis and Pseudomonas putida. Environmental Technology & Innovation, 10, 111–121. https://doi.org/10.1016/j.eti.2018.01.001
- Basyal, B., & Emery, S. M. (2021). An arbuscular mycorrhizal fungus alters switchgrass growth, root architecture, and cell wall chemistry across a soil moisture gradient. Mycorrhiza, 31(2), 251–258. https://doi.org/10.1007/s00572-020-00992-6
- Begum, N., Qin, C., Ahanger, M. A., Raza, S., Khan, M. I., Ashraf, M., Ahmed, N., & Zhang, L. (2019). Role of arbuscular mycorrhizal fungi in plant growth regulation: Implications in abiotic stress tolerance. Frontiers in Plant Science, 10, 1068. https://doi.org/10.3389/fpls.2019.01068
- Bethlenfalvay, G. J., Brown, M. S., Ames, R. N., & Thomas, R. S. (1988). Effects of drought on host and endophyte development in mycorrhizal soybeans in relation to water use and phosphate uptake. Physiologia Plantarum, 72(3), 565–571. https://doi.org/10.1111/j.1399-3054.1988.tb09166.x
- Bonfante, P., & Genre, A. (2010). Mechanisms underlying beneficial plant–fungus interactions in mycorrhizal symbiosis. Nature Communications, 1(1), 1–11. https://doi.org/10.1038/ncomms1046
- Chandrasekaran, M. (2020). A meta-analytical approach on arbuscular mycorrhizal fungi inoculation efficiency on plant growth and nutrient uptake. Agriculture, 10(9), 370. https://doi.org/10.3390/agriculture10090370
- Chareesri, A., de Deyn, G. B., Sergeeva, L., Polthanee, A., & Kuyper, T. W. (2020). Increased arbuscular mycorrhizal fungal colonization reduces yield loss of rice (Oryza sativa L.) under drought. Mycorrhiza, 30(2–3), 315–328. https://doi.org/10.1007/s00572-020-00953-z
- Fabbrin, E. G., Gogorcena, Y., Mogor, Á. F., Garmendia, I., & Goicoechea, N. (2015). Pearl millet growth and biochemical alterations determined by mycorrhizal inoculation, water availability and atmospheric CO2 concentration. Crop and Pasture Science, 66(8), 831–840. https://doi.org/10.1071/CP14089
- FAO. (2022). International Year of Millets 2023. In Communication Handbook and Toolkit, FAO (p. 21). Rome, Italy.
- Farooq, M., Wahid, A., Kobayashi, N., Fujita, D., & Basra, S. M. A. (2009). Plant drought stress: Effects, mechanisms and management. Agronomy for Sustainable Development, 29(1), 185–212. https://doi.org/10.1051/agro:2008021
- Giovannetti, M., & Mosse, B. (1980). An evaluation of techniques for measuring vesicular arbuscular mycorrhizal infection in roots. New Phytologist, 84(3), 489–500. https://doi.org/10.1111/j.1469-8137.1980.tb04556.x
- Hayashi, M., Niwa, R., Urashima, Y., Suga, Y., Sato, S., Hirakawa, H., Yoshida, S., Ezawa, T., & Karasawa, T. (2018). Inoculum effect of arbuscular mycorrhizal fungi on soybeans grown in long-term bare-fallowed field with low phosphate availability. Soil Science and Plant Nutrition, 64(3), 306–311. https://doi.org/10.1080/00380768.2018.1473007
- Hetrick, B. A. D., Leslie, J. F., Wilson, G. T., & Kitt, D. G. (1988). Physical and topological assessment of effects of a vesicular-arbuscular mycorrhizal fungus on root architecture of big bluestem. New Phytologist, 110(1), 85–96. https://doi.org/10.1111/j.1469-8137.1988.tb00240.x
- Isobe, K., & Tsuboki, Y. (1998). The relationship between growth promotion by arbuscular mycorrhizal fungi and root morphology and phosphorus absorption in gramineous and leguminous crops. Japanese Journal of Crop Science, 67(3), 347–352. in Japanese with English abstract. https://doi.org/10.1626/jcs.67.347.
- Jabborova, D., Annapurna, K., Al-Sadi, A. M., Alharbi, S. A., Datta, R., & Zuan, A. T. K. (2021). Biochar and arbuscular mycorrhizal fungi mediated enhanced drought tolerance in okra (Abelmoschus esculentus) plant growth, root morphological traits and physiological properties. Saudi Journal of Biological Sciences, 28(10), 5490–5499. https://doi.org/10.1016/j.sjbs.2021.08.016
- Kakouridis, A., Hagen, J. A., Kan, M. P., Mambelli, S., Feldman, L. J., Herman, D. J., Weber, P. K., Pett‐Ridge, J., & Firestone, M. K. (2022). Routes to roots: Direct evidence of water transport by arbuscular mycorrhizal fungi to host plants. New Phytologist, 236(1), 210–221. https://doi.org/10.1111/nph.18281
- Karasawa, T., Takebe, M., & Kasahara, Y. (2000). Arbuscular mycorrhizal (AM) effects on maize growth and AM colonization of roots under various soil moisture conditions. Soil Science and Plant Nutrition, 46(1), 61–67. https://doi.org/10.1080/00380768.2000.10408762
- Kato, Y., Kamoshita, A., Yamagishi, J., & Abe, J. (2006). Growth of three rice (Oryza sativa L.) cultivars under upland conditions with different levels of water supply 1. Nitrogen content and dry matter production. Plant Production Science, 9(4), 422–434. https://doi.org/10.1626/pps.9.422
- Kobae, Y. (2019). Dynamic phosphate uptake in arbuscular mycorrhizal roots under field conditions. Frontiers in Environmental Science, 6, 159. https://doi.org/10.3389/fenvs.2018.00159
- Konvalinková, T., Püschel, D., Janoušková, M., Gryndler, M., & Jansa, J. (2015). Duration and intensity of shade differentially affects mycorrhizal growth- and phosphorus uptake responses of Medicago truncatula. Frontiers in Plant Science, 6(FEB), 65. https://doi.org/10.3389/fpls.2015.00065
- Luo, X., Shi, S., Liu, Y., Yang, H., Li, N., Dong, Z., Zhu, B., & He, X. (2021). Arbuscular mycorrhizal fungal communities of topsoil and subsoil of an annual maize-wheat rotation after 15-years of differential mineral and organic fertilization. Agriculture, Ecosystems & Environment, 315, 107442. https://doi.org/10.1016/j.agee.2021.107442
- Marro, N., Grilli, G., Soteras, F., Caccia, M., Longo, S., Cofré, N., Borda, V., Burni, M., Janoušková, M., & Urcelay, C. (2022). The effects of arbuscular mycorrhizal fungal species and taxonomic groups on stressed and unstressed plants: A global meta‐analysis. New Phytologist, 235(1), 320–332. https://doi.org/10.1111/nph.18102
- Morimoto, S., Uchida, T., Matsunami, H., & Kobayashi, H. (2018). Effect of winter wheat cover cropping with no-till cultivation on the community structure of arbuscular mycorrhizal fungi colonizing the subsequent soybean. Soil Science and Plant Nutrition, 64(5), 545–553. https://doi.org/10.1080/00380768.2018.1486171
- Müller, A., Eltigani, A., & George, E. (2019). The abundance of arbuscular mycorrhizal fungal species in symbiosis with okra plants is affected by induced drought conditions in a calcareous substrate. Rhizosphere, 10, 100150. https://doi.org/10.1016/j.rhisph.2019.100150
- Nguyen, H. T. A., Kamoshita, A., Ramalingam, P., & Y, P. (2022). Genetic analysis of root vascular traits in a population from two temperate japonica rice ecotypes. Plant Production Science. https://doi.org/10.1080/1343943X.2022.2085588
- Niwa, R., Koyama, T., Sato, T., Adachi, K., Tawaraya, K., Sato, S., Hirakawa, H., Yoshida, S., & Ezawa, T. (2018). Dissection of niche competition between introduced and indigenous arbuscular mycorrhizal fungi with respect to soybean yield responses. Scientific Reports, 8, 1–11. https://doi.org/10.1038/s41598-018-25701-4
- Oehl, F., Sieverding, E., Ineichen, K., Ris, E., Boller, T., & Wiemken, A. (2005). Community structure of arbuscular mycorrhizal fungi at different soil depths in extensively and intensively managed agroecosystems. New Phytologist, 165(1), 273–283. https://doi.org/10.1111/j.1469-8137.2004.01235.x
- Oliveira, T. C., Cabral, J. S. R., Santana, L. R., Tavares, G. G., Santos, L. D. S., Paim, T. P., Müller, C., Silva, F. G., Costa, A. C., Souchie, E. L., & Mendes, G. C. (2022). The arbuscular mycorrhizal fungus Rhizophagus clarus improves physiological tolerance to drought stress in soybean plants. Scientific Reports, 12(1), 1–15. https://doi.org/10.1038/s41598-022-13059-7
- P, Y. (2023). Effects of water regime and inoculation with arbuscular mycorrhizal fungi on growth and mycorrhizal communities of rice and pearl millet. Doctoral Dissertation, The University of Tokyo.
- Phillips, J. M., & Hayman, D. S. (1970). Improved procedures for clearing roots and staining parasitic and vesicular-arbuscular mycorrhizal fungi for rapid assessment of infection. Transactions of the British Mycological Society, 55(1), 158–IN18. https://doi.org/10.1016/S0007-1536(70)80110-3
- Quiroga, G., Erice, G., Aroca, R., Chaumont, F., & Ruiz-Lozano, J. M. (2017). Enhanced drought stress tolerance by the arbuscular mycorrhizal symbiosis in a drought-sensitive maize cultivar is related to a broader and differential regulation of host plant aquaporins than in a drought-tolerant cultivar. Frontiers in Plant Science, 8(June), 1–15. https://doi.org/10.3389/fpls.2017.01056
- Rillig, M. C., & Field, C. B. (2003). Arbuscular mycorrhizae respond to plants exposed to elevated atmospheric CO2 as a function of soil depth. Plant and Soil, 254(2), 383–391. https://doi.org/10.1023/A:1025539100767
- Ruiz-Sánchez, M., Aroca, R., Muñoz, Y., Polón, R., & Ruiz-Lozano, J. M. (2010). The arbuscular mycorrhizal symbiosis enhances the photosynthetic efficiency and the antioxidative response of rice plants subjected to drought stress. Journal of Plant Physiology, 167(11), 862–869. https://doi.org/10.1016/j.jplph.2010.01.018
- Ruiz-Sánchez, M., Cabrera-Rodríguez, J. A., Dell Amico-Rodríguez, J. M., Muñoz-Hernández, Y., Aroca-Álvarez, R., & Ruiz-Lozano, J. M. (2021). Categorization of the water status of rice inoculated with arbuscular mycorrhizae and with water deficit. Agronomy Mesoamerican, 32(2), 339–355. https://doi.org/10.15517/am.v32i2.42066
- Salomon, M. J., Demarmels, R., Watts-Williams, S. J., McLaughlin, M. J., Kafle, A., Ketelsen, C., Soupir, A., Bücking, H., Cavagnaro, T. R., & van der Heijden, M. G. A. (2022). Global evaluation of commercial arbuscular mycorrhizal inoculants under greenhouse and field conditions. Applied Soil Ecology, 169, 104225. https://doi.org/10.1016/j.apsoil.2021.104225
- Sato, K., Suyama, Y., Saito, M., & Sugawara, K. (2005). A new primer for discrimination of arbuscular mycorrhizal fungi with polymerase chain reaction-denature gradient gel electrophoresis. Grassland Science, 51(2), 179–181. https://doi.org/10.1111/j.1744-697X.2005.00023.x
- Sisaphaithong, T., Hanai, S., Tomioka, R., Kobae, Y., Tanaka, A., Yano, K., Takenaka, C., & Hata, S. (2017). Varietal differences in the growth responses of rice to an arbuscular mycorrhizal fungus under natural upland conditions. Plant Signaling and Behavior, 12(1), e1274483. https://doi.org/10.1080/15592324.2016.1274483
- Smith, S. E., Jakobsen, I., Grønlund, M., & Smith, F. A. (2011). Roles of arbuscular mycorrhizas in plant phosphorus nutrition: Interactions between pathways of phosphorus uptake in arbuscular mycorrhizal roots have important implications for understanding and manipulating plant phosphorus acquisition. Plant Physiology, 156(3), 1050–1057. https://doi.org/10.1104/PP.111.174581
- Sosa-Hernández, M. A., Leifheit, E. F., Ingraffia, R., & Rillig, M. C. (2019). Subsoil arbuscular mycorrhizal fungi for sustainability and climate-smart agriculture: A solution right under our feet? Frontiers in Microbiology, 10(APR), 744. https://doi.org/10.3389/fmicb.2019.00744
- Sosa-Hernández, M. A., Roy, J., Hempel, S., & Rillig, M. C. (2018). Evidence for subsoil specialization in arbuscular mycorrhizal fungi. Frontiers in Ecology and Evolution, 6(MAY), 67. https://doi.org/10.3389/fevo.2018.00067
- Sylvia, D. M., Hammond, L. C., Bennett, J. M., Haas, J. H., & Linda, S. B. (1993). Field response of maize to a VAM fungus and water management. Agronomy Journal, 85(2), 193–198. https://doi.org/10.2134/agronj1993.00021962008500020006x
- Vallino, M., Fiorilli, V., & Bonfante, P. (2014). Rice flooding negatively impacts root branching and arbuscular mycorrhizal colonization, but not fungal viability. Plant, Cell & Environment, 37(3), 557–572. https://doi.org/10.1111/pce.12177
- Van Geel, M., Busschaert, P., Honnay, O., & Lievens, B. (2014). Evaluation of six primer pairs targeting the nuclear rRNA operon for characterization of arbuscular mycorrhizal fungal (AMF) communities using 454 pyrosequencing. Journal of Microbiological Methods, 106, 93–100. https://doi.org/10.1016/j.mimet.2014.08.006
- Verbruggen, E., van der Heijden, M. G., Rillig, M. C., & Kiers, E. T. (2013). Mycorrhizal fungal establishment in agricultural soils: Factors determining inoculation success. New Phytologist, 197(4), 1104–1109. https://doi.org/10.1111/j.1469-8137.2012.04348.x
- Wang, Y., Bao, X., & Li, S. (2021). Effects of arbuscular mycorrhizal fungi on rice growth under different flooding and shading regimes. Frontiers in Microbiology, 12(October), 1–15. https://doi.org/10.3389/fmicb.2021.756752
- Watanarojanaporn, N., Boonkerd, N., Tittabutr, P., Longtonglang, A., Young, J. P. W., & Teaumroong, N. (2013). Effect of rice cultivation systems on indigenous arbuscular mycorrhizal fungal community structure. Microbes and Environments, 28(3), 316–324. https://doi.org/10.1264/jsme2.ME13011
- Yamagishi, J., Nakamoto, T., & Richner, W. (2003). Stability of spatial variability of wheat and maize biomass in a small field managed under two contrasting tillage systems over 3 years. Field Crops Research, 81(2–3), 95–108. https://doi.org/10.1016/S0378-4290(02)00213-7
- Y, P., Kamoshita, A., Norisada, M., & Deshmukh, V. (2020). Eco-physiological evaluation of Stele transversal area 1 for rice root anatomy and shoot growth. Plant Production Science, 23(2), 202–210. https://doi.org/10.1080/1343943X.2020.1727754
- Zhang, F., Zou, Y. N., & Wu, Q. S. (2018). Quantitative estimation of water uptake by mycorrhizal extraradical hyphae in citrus under drought stress. Scientia Horticulturae, 229, 132–136. https://doi.org/10.1016/j.scienta.2017.10.038
- Zou, Y. N., Wang, P., Liu, C. Y., Ni, Q. D., Zhang, D. J., & Wu, Q. S. (2017). Mycorrhizal trifoliate orange has greater root adaptation of morphology and phytohormones in response to drought stress. Scientific Reports, 7(1), 1–10. https://doi.org/10.1038/srep41134