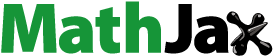
Abstract
Poly(amidoamine)s (PAMAMs) incorporated into a cross-linked poly(ethylene glycol) exhibited excellent CO2 separation properties over H2. However, the CO2 permeability should be increased for practical applications. Monoethanolamine (MEA) used as a CO2 determining agent in the current CO2 capture technology at demonstration scale was readily immobilized in poly(vinyl alcohol) (PVA) matrix by solvent casting of aqueous mixture of PVA and the amine. The resulting polymeric membranes can be self-standing with the thickness above 3 μm and the amine fraction less than 80 wt%. The gas permeation properties were examined at 40 °C and under 80% relative humidity. The CO2 separation performance increased with increase of the amine content in the polymeric membranes. When the amine fraction was 80 wt%, the CO2 permeability coefficient of MEA containing membrane was 604 barrer with CO2 selectivity of 58.5 over H2, which was much higher than the PAMAM membrane (83.7 barrer and 51.8, respectively) under the same operation conditions. On the other hand, ethylamine (EA) was also incorporated into PVA matrix to form a thin membrane. However, the resulting polymeric membranes exhibited slight CO2-selective gas permeation properties. The hydroxyl group of MEA was crucial for high CO2 separation performance.
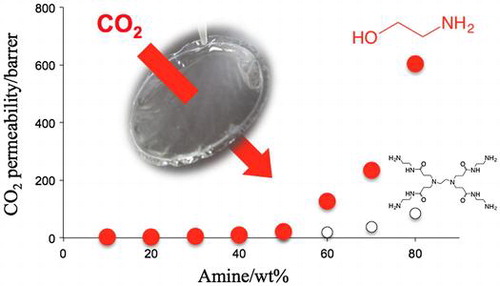
1. Introduction
CO2 is the major component of greenhouse gases (GHGs), which cause the global warming and climate change. Addressing the GHG issue, the Paris agreement has warned to limit a global temperature rise to 1.5 °C [Citation1], and mitigation of the GHG emission is an urgent task to be tackled. Carbon capture and storage (CCS) has been approved as one of the effective solutions toward the issue, and about 20 full-scale CCS demonstrations have been planned or carried out all over the world [Citation2]. In the CCS, CO2 is captured at the mass emission sources without exhausting to atmosphere, such as thermal power plants and steel works, and then injected into aquifer of certain geological structures underground or under seabed [Citation3]. However, for implementation of the CCS, the cost reduction is inevitable, in which the CO2 capture dominates of the total. Liquid amine scrubbing is the most widely investigated CO2 capture method [Citation4,5] and adopted in the large-scale demonstrations [Citation2]. For example, CO2 in the flue gas from a coal-fired plant was captured by aqueous amine over other gaseous species, and then recovered by heating. The heating process is energy intensive even at the thermal power stations, which resulted in high cost. Thus, alternate low-energy CO2 capture technologies have to be developed for the implementation of CCS. Among various CO2 capture technologies, our institute focuses on membrane separation because transmembrane difference in concentration or chemical potential drives the separation and no additional energy is basically required. The smaller footprint of the facility, instant operation and lower maintenance cost are also important advantages in comparison to the current CO2 capture technology. Various CO2 separation membranes have been developed including organic, inorganic and hybrid membranes, such as mixed matrix membranes (MMMs) [Citation6–8].
CO2 separation over H2 by membranes has been a topic for pre-combustion CO2 capture at an integrated gasification combined cycle (IGCC) plant, where the target gas consisted of mostly H2 and CO2 is pressurized, and the membrane separation is going to be suitable with organic or polymeric membranes. For the CO2 capture, CO2 is separated over smaller H2, and porous inorganic membranes and MMMs are not CO2 selective because the separation proceeds by molecular sieving mechanism. Polymeric membranes are dense and non-porous, and the gas permeability is explained by the following expression (Equation (Equation1(1)
(1) )). Ideal gas selectivity for gas i over j αi/j is then given by the ratio of permeabilities as Equation (Equation2
(2)
(2) ).
(1)
(1)
(2)
(2)
where S and D are solubility to the polymer matrix and diffusivity in the polymer, respectively [Citation9]. Because the kinetic diameter of H2 (2.89 Å) is smaller than that of CO2 (3.30 Å), D(CO2)/D(H2) is smaller than one. For preferential CO2 permeation, S(CO2) should be much greater than S(H2). Amines have been incorporated physically or chemically into the polymeric membranes to enhance CO2 solubility in the membranes [Citation10–15], where the amines also work as a CO2 carrier to facilitate the gas permeation through the membranes. PAMAM exhibits excellent CO2 separation performance [Citation10,16], and the CO2 separation properties of PAMAM immobilized poly(ethylene glycol) (PEG) membranes have been extensively investigated in this research group [Citation17–20]. The PAMAM membranes indeed exhibited excellent CO2 selectivity over H2, but they had a critical drawback. The CO2 permeability was not enough for the application, such as CO2 capture at the IGCC [Citation21]. For the pre-combustion CO2 capture by membranes, CO2 permeance should be equal or above 100 GPU with 30 in the selectivity in a two-stage membrane separation process [Citation21]. A plausible approach to elevate gas permeability is to reduce the membrane thickness. However, macrophase separation between the amines and PEG matrix on a couple of microns scale did not allow thinning the PEG membranes due to serious leakage of the physically entrapped amines from the matrix [Citation18]. Herein, we described a solution to solve the above shortcoming with an alternate polymer matrix, PVA. PVA itself is known to have gas barrier nature and good membrane formability [Citation22]. It also shows better compatibility to the amines and has been used as a membrane matrix for CO2 capture [Citation11,13–15,21]. In addition, the CO2 separation properties of EA and MEA were compared with PAMAM in the polymer matrix. MEA is inexpensive and one of the most studied amines in liquid amine scrubbing [Citation6,23,24]. Importance of the hydroxyl group was examined in the membrane separation.
2. Materials and methods
2.1. Materials
PAMAM (G 0, 20 wt% in methanol), PEG dimethacrylate (PEGDMA, MW 750), and 1-hydroxycyclohexyl phenylketone (Irgacure 184) were purchased from Sigma-Aldrich (Missouri, USA), and ethylamine (70 wt% in water) was from TCI (Tokyo, Japan). MEA and PVA (Mw: 66,000, saponification: 85–90%) were obtained from Wako (Osaka, Japan). Other organic and inorganic chemicals were reagent grade and used without further purification. Gases with the highest purity grade commercially available were used for gas permeation experiment.
2.2. Membrane preparation process
For amine-containing PEG membranes, PEGDMA and amines were dissolved in ethanol, where the amine:PEGDMA:solvent ratio was kept to 6:4:5 by weight, and the monomer to initiator ratio was also kept by the molar ratio of 60. The reaction mixture was sandwiched with quartz plates with stainless spacers (50 μm in thickness) and exposed to UV light (365 nm, 12 mW/cm2, UVP B-100AP, CA, USA) for 3 min to polymerize PEGDMA [Citation17]. The resulting polymeric membranes were dried under vacuum overnight. On the other hand, with amine-containing PVA membranes, 5 wt% of aqueous PVA was prepared by dissolving predetermined amount of PVA in deionized water at 90 °C overnight under stirring. Amines were then added to the aqueous PVA, where the weight fraction of them was varied from 10 to 90 wt% relative to the polymer. The resulting mixture was cast on a plastic petri dish and dried at ambient temperature for 2 days and then under vacuum at 40 °C for at least 6 h until no weight change was recorded. The membrane thickness was controlled by varying the casting amount and less than 50 μm. The membrane thickness was determined by a Mitutoyo digimatic micrometer (Tokyo, Japan).
2.3. Membrane characterizations
The thermal properties of amine-containing membranes were examined by differential scanning calorimetry (DSC) on a Netzsch DCS 204 F1 Phoenix (Netzsch, Kanagawa, Japan). 5–9 mg of the dried specimen was first cooled down to −100 °C, and thermal transitions upon heating were recorded with a heating rate of 10 °C/min from −100 to 250 °C. Because the amines were physically immobilized in the polymer matrices, they would be leached out from the polymer matrix in ethanol. The dried MEA- and PAMAM-containing membranes (ca. 120 mg) were dipped in 50 mL ethanol to remove the amine. The eluting solvent was changed every 12 h for 3 days. Because MEA was volatile under vacuum and heating, the eluted compounds in ethanol for PAMAM-containing membranes were recovered and determined by proton nuclear magnetic resonance (1H NMR) on a 600 MHz Avance III HD (Bruker, Kanagawa, Japan). Morphology of the resulting membrane was studied with a Hitachi S-4800 filed-emission scanning electron microscope (FE-SEM, Hitachi, Tokyo, Japan). The cross section of the membrane was prepared by freeze fracturing in liquid N2, and the obtained surface was coated with Pt before the SEM observation.
2.4. Gas permeation experiment
CO2 separation properties of amine-containing polymeric membranes were examined under isobaric conditions. Amin-containing polymeric membranes were placed on a porous PVDF support with nominal pore size of 0.45 μm (Durapore® membrane filter, HVLP02500, Merck Millipore) and set in a custom made flat sheet membrane cell [Citation20], where the effective membrane area A was 2.27 × 10−4 m2. A CO2/H2 gas mixture (40/60 vol%) was humidified by passing through a water bath and fed to the membrane cell at a flow rate of 100 mL/min and 40 °C, and the CO2 partial pressure was 63 kPa in the operation conditions. Humidified He was supplied to collect the permeate at a flow rate of 10 mL/min and 40 °C. Gaseous species in both of the retentate and permeate were determined on an Agilent 7890A gas chromatograph equipped with a thermal conductivity detector and a pulse discharge helium ionization detector, respectively (Agilent Technologies, Tokyo, Japan). Gas permeability coefficient Pi and permeance Qi were defined by the following equations.(3)
(3)
(4)
(4)
where, Ni, l, t, and Δpi were gas flux, membrane thickness, transmission time, and transmembrane pressure differences of gas i, respectively. The stage cut, a gas flux ratio of permeate/retentate, was controlled to less than 0.01, and under the experimental conditions, the pressure ratios of feed to permeate were sufficient to give the separation factor α(CO2/H2) as an ideal separation factor. The separation factor was expressed by Equation (Equation3(3)
(3) ), where xi and yi were molar fraction of gas i in the feed and the permeate. The gas permeability coefficient and permeance were expressed in barrer and GPU, respectively; 1 barrer = 7.5 × 10−18 m3(STP)·m/(m2 s Pa) and 1 GPU = 7.5 × 10−12 m3(STP)/(m2 s Pa).
(5)
(5)
3. Results and discussion
CO2 separation by amine-containing polymeric membranes has been studied in this research group. Amines were readily immobilized in a cross-linked PEG by UV curing of PEGDMA in the presence of the amines [Citation17]. However, poor compatibility between amines and PEG resulted in forming opaque or translucent membranes, which was due to macrophase separation on a couple of microns scale between amines and the matrix [Citation18]. Thus, when the membrane thickness was reduced less than a few tens microns, leakage of amines were found. As a result, the membrane lost the CO2 separation performance. In comparison to the translucent PEG membranes, the amine-containing PVA membranes were transparent as shown in Figure . The better compatibility between amines and the matrix would reduce the phase separation.
Figure 1. Optical images of (a) MEA-containing PVA, (b) MEA-containing PEG, (c) PAMAM-containing PVA and (d) PAMAM-containing PEG membranes.
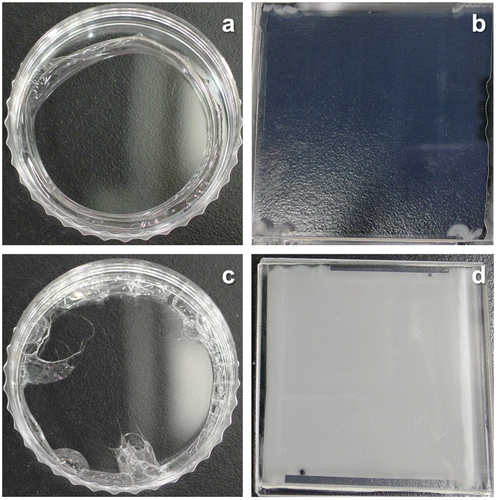
One approach to examine the macrophase separation was expected to see thermal properties on DSC. The properties of the amine-containing PVA membranes would be different from pristine PVA due to change in the heat capacity upon phase mixing. Figure displayed the DSC profiles of various amine-containing polymeric membranes. While pristine PVA membrane showed the glass transition temperature (Tg) at 55 °C in Figure (e), the thermal transition of the amine-containing PVA membranes became ambiguous (Figure (a)–(c)). The glassy PVA became rubbery upon complexation with the amines. On the contrary, the amine-containing PEG membranes showed a clear thermal transition between −60 and −50 °C in Figure (d) and (e), which was corresponded to the Tg of PEG of the polymer matrix. Due to poor miscibility between the amines and PEG, a PEG-rich domain was formed, and the PEG matrix thus kept the inherent thermal properties even after the amine loading. An exothermic and endothermic peaks at −30 and 3 °C indicated crystallization and melting of MEA in Figure (d). The melting peak of PAMAM overlapped with the Tg of PEG and was not clear in Figure (e).
Figure 2. DSC thermographs of (a) MEA-containing PVA, (b) PAMAM-containing PVA, (c) EA-containing PVA, (d) MEA-containing PEG, (e) PAMAM-containing PEG and (f) pristine PVA membranes at heating rate of 10 °C/min.
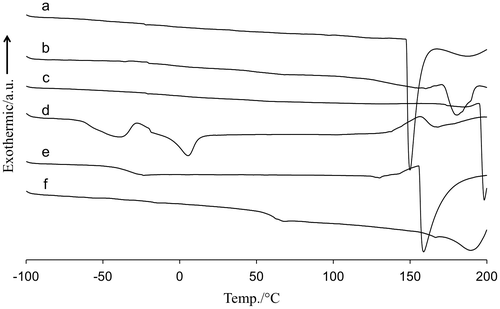
The macrophase separation was also studied by eluting out the incorporated amines in ethanol [Citation25], which was a poor solvent for the polymer matrices. When the amine-containing PEG and PVA membranes were immersed in ethanol, physically immobilized amines came out into the solvent. For the PAMAM-containing membranes, the solutes were collected and determined as the incorporated amines in the matrices by 1H NMR as shown in Figure . This result suggested that the amines stayed intact in the polymer matrices even during the photopolymerization process to fabricate PEG membranes. In other words, Michael addition reaction between amines and vinyl compounds were negligible in the UV curing conditions. With the PAMAM-containing PEG membrane in Figure (top spectrum), a peak was found at 3.6 ppm, which was the resonance of methylene protons of PEG. Oligomeric PEG would be released from the matrix. Because peaks of allyl protons of PEGDMA at 6.2–5.5 ppm were not seen, the monomer conversion would be completed.
Figure 3. 600 MHz 1H NMR spectra of residues in ethanol after amine elution test of (top) PAMAM-containing PEG and (bottom) PAMAM-containing PVA membranes in methanol-d4 at 25 °C, and the assignment of the peaks.
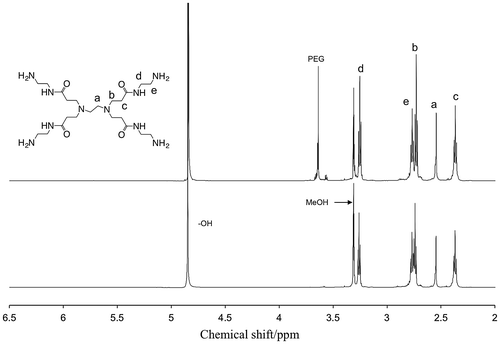
On the other hand, with the MEA-containing membranes, MEA was found in ethanol by 1H NMR: 3.57 and 2.71 ppm for O–CH2 and N–CH3, respectively, in deuterated methanol. However, the amine was evaporated with ethanol during dry process, and the recovery was thus not precisely determined. In the PEG membranes, it was confirmed that the matrices were quantitatively recovered or physically entrapped amines were completely eluted out by the amine leaching experiment. On the contrary, while the amine fraction was 60 wt%, the weight reductions were 41 and 44% for the MEA- and PAMAM-containing PVA membranes. Better compatibility of the matrix to the amines suppressed the leakage from the membranes.
The resulting matrices after the amine leaching were observed by SEM, and the obtained cross-section images were displayed in Figure . With the amine-containing PEG membranes, porous structures were found in the resulting matrix in Figure (b) and (d). The Tg of PEG was −60 °C and would be flexible during the amine leaching experiment at ambient conditions. However, cross-linking reaction confined the rearrangement of PEG chains, and the PEG-rich phase was fixed. As a result, the monolith like structure with micron-sized pores was remained, and the pore was originally filled with the amines immobilized in the UV curing of PEGDMA. The macrophase separated structures on a couple of microns scale resulted in the translucent membranes.
Figure 4. Cross-section SEM images of (a) MEA-containing PVA, (b) MEA-containing PEG, (c) PAMAM-containing PVA and (d) PAMAM-containing PEG membranes after amine elution test (bar: 10 μm).
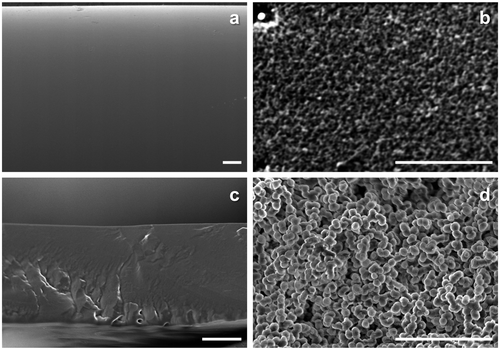
On the other hand, the amine-containing PVA membranes were transparent. Here, when the amines and PVA would be incompatible and have a similar photorefractive index, diffused reflection of light would be suppressed at the interface, and the resulting mixture would be transparent. In the case of the PVA membranes, quantitative recovery of the amines from the membranes was also confirmed after the ethanol immersion, and a flat and smooth surface was seen in Figure (a) and (b). When the entrapped amines formed the rich phase upon macrophase separation similar to the PEG membranes, a porous structure would be found because PVA was insoluble in the solvent and in a grassy state (Tg: 55 °C in Figure (f)) in the experimental conditions. Thus, combined with the DSC results, it could be concluded that the amines and PVA were miscible on a micron scale, and a thinner membrane preparation was expected to be possible without amine leakage for the PVA matrix.
Gas permeation properties of amine-containing membranes were then investigated under isobaric conditions at 40 °C. The gas permeabilities changed with time by absorbing water under humidity and mostly reached equilibrium after 12 to 20 h incubation at the operation conditions as shown in Figure . Table described a comparison of CO2 separation properties of MEA-containing PVA and PEG membranes over H2. The membrane thickness was measured immediately after the gas permeation test under humidified conditions. The PEG membranes were 52.7 μm in thickness, which was the minimum to prevent the amine leakage from the PEG matrix, and much thicker than the PVA ones (5.7 μm). The CO2 permeance of the PEG membranes was 7.9 GPU and lower than that of the PVA membranes (16.2 GPU). The higher CO2 selectivity of the PEG membranes was explained by higher amount of the amines, which worked as a CO2 carrier to facilitate the gas transportation through the membranes. On the other hand, the PVA membranes retained the CO2 separation properties over H2 by reducing the thickness to ca. 6 μm to give higher CO2 permeance.
Figure 5. Change in gas transport properties of MEA-containing PVA membranes at 40 °C and 85% relative humidity (Δp(CO2) = 63 kPa) as a function of time.
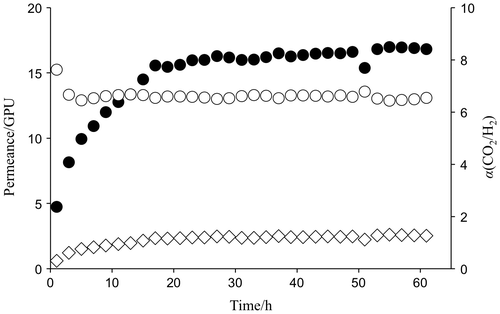
Table 1. CO2 separation properties of MEA-containing PVA and PEG membranes over H2 at 40 °C and 90% relative humidity.
In the PVA membrane preparation process, the amine content was varied from 10 to 90 wt% relative to PVA matrix, and self-standing membranes were obtained with the amine content of equal or less than 80 wt%. The amine leakage was negligible under the gas permeation operation conditions. Effect of amine loading on the CO2 transport properties was then investigated with self-standing membranes, and the results were shown in Figure . Because the membrane thicknesses of the specimens were from 3 to 43 μm depending on the amines and the contents, the gas permeability was expressed by permeability coefficient P, which was permeance normalized by the membrane thickness, 1 barrer corresponded to 1 GPU when the thickness was 1 μm.
Figure 6. Effect of amine content on gas permeation properties of (a) EA- and (b) PAMAM and (c) MEA-containing PVA membranes at 40 °C and 80% relative humidity (Δp(CO2) = 63 kPa).
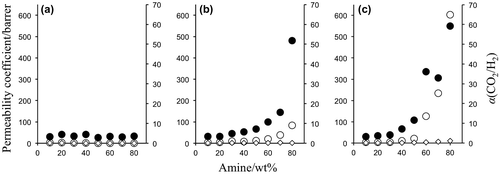
For MEA- and PAMAM-containing PVA membranes, the CO2 permeance and separation factor increased with increase of the amine content, which indicated that the preferential CO2 permeation was based on facilitated transportation by the amines. The CO2 transport mechanism was different from those of the PEG membranes [Citation17]. In the case of PAMAM-containing PEG membranes, the CO2 permeability slightly increased with increase of PAMAM content in the similar operation conditions. On the other hand, the H2 permeability significantly decreased, and as a result, the separation factor increased drastically with increase of the amine content. In the PEG membranes, the PAMAM-rich phase was formed upon microphase separation [Citation18,25], and CO2 migrated the amine-rich phase rather than the PEG-rich phase due to difference in the CO2 solubility. When CO2 was fed to the membranes, dissolved CO2 reacted to the primary amines of PAMAM with the formation of carbamate as illustrated in Figure . PAMAM was crosslinked by the resulting carbamate ion pairs, and the quasi-crosslinks restricted the H2 permeation to provide a quite high CO2 selectivity by a CO2-selective molecular gate function [Citation16,19].
On the contrary, the amines were compatible to PVA and did not form such amine-rich phase in the membranes confirmed by the SEM observations in Figure . The amines were diffused homogeneously through the PVA membranes and worked as a CO2 carrier to provide higher gas permeability, which was more preferable rather than the higher selectivity for practical CO2 capture [Citation26].
Chemical structure of the amines was one of the crucial factors to characterize the CO2 separation performance in the PVA membranes. As depicted in Figure , MEA gave the highest CO2 permeation properties among the amines tested in the same weight fraction. With increase of MEA content from 10 to 80 wt%, the CO2 permeance and separation factor went up from 3.6 to 604 barrer and from 3.3 to 59, respectively. EA had the same carbon skeleton as MEA but no hydroxyl group, and the amine-containing membranes did not have effective CO2 separation performance. Thus, hydroxyl group of the alkanolamine would be a key to exhibit preferential CO2 permeation properties. The CO2 separation performance of the MEA-containing PVA membranes was actually higher than that of the PAMAM membranes. The CO2 permeability of the PAMAM membranes was less than 100 barrer and far below of that of the MEA membranes, while the separation factor was relatively smaller at the same amine weight fraction. A hydroxyl group in β-carbon adjacent to amino group helped to form a seven-membered ring when CO2 interacted to the amino group in the polymeric membranes [Citation27,28]. The ring formation reduced the activation energy of the interaction and facilitated both of the association and dissociation between CO2 and the amino group, resulting in enhancing CO2 diffusion in the membranes.
4. Conclusions
The PAMAM-containing membranes have been studied for effective CO2 separation over H2. To reduce macrophase separation between the amine and the matrix, PVA was employed to improve compatibility to the amine. The resulting PVA membranes were transparent, and the DSC and the amine elution experiments clarified suppression of the macrophase separation. A thinner membrane formation was then possible with PVA matrix by reducing the phase separation, and an increase in CO2 permeability was confirmed. Furthermore, it was suggested that MEA exhibited higher CO2 permeability and selectivity than PAMAM in the polymer matrices. Hydroxyl group adjacent to the amino group would be a key to enhance the CO2 separation performance.
In this research, the CO2 separation properties over H2 was investigated under isobaric conditions, which was lower than the CO2 partial pressure of syngas after water-gas shift reaction at an IGCC. Thus, the separation performance under pressure was not clear. On the other hand, this membrane would hold potential to capture CO2 in the industrial H2 production process by steam reforming of light hydrocarbons. For example, H2 is generated from CH4 by the steam reforming followed by the shift reaction, and then purified over CO2 by pressure-swing adsorption (PSA). The PSA off-gas comes out at ambient pressure and contains certain amount of H2, which is used as fuel to keep the reformer at elevated temperature, and CO2 is in the off-gas eventually emitted. A feasibility study revealed that the CO2 separation properties observed could meet the required values to separate CO2 in the off-gas [Citation29] and that the amine-containing PVA membranes would be applicable to make the current H2 process carbon-free.
Disclosure statement
No potential conflict of interest was reported by the authors.
Funding
This work was supported by Grant-in-Aid for Scientific Research (C) [grant number JP17899334]; the Advanced Carbon Technology Research and Development Program from Japan Science and Technology Agency; The International Institute for Carbon-Neutral Energy Research (WPI-I2CNER), World Premier International Research Center Initiative (WPI), MEXT, Japan.
Supplemental data
Supplemental data for this article can be accessed at https://doi.org/10.1080/14686996.2017.1399045
Supplemental_material.docx
Download MS Word (128.1 KB)Acknowledgements
The authors thank Shigenori Fujikawa for use of the SEM apparatus.
References
- Framework Convention on Climate Change. United Nations: The Paris Agreement; [cited 2017 Jun 15]. Available from: http://bigpicture.unfccc.int/#content-the-paris-agreemen
- International Energy Agency. 20 years of carbon capture and storage. [cited 2017 Jun 15]. Available from: https://www.iea.org/publications/freepublications/publication/20-years-of-carbon-capture-and-storage.html
- Metz B, Davidson O, de Coninck H, et al. Carbon dioxide capture and storage: IPCC special report of the Intergovernmental Panel on Climate Change. Cambridge: Cambridge University Press; 2005.
- Kumar S, Cho JH, Moon IL. Ionic liquid-amine blends and CO2BOLs: prospective solvents for natural gas sweetening and CO2 capture technology – a review. Int J Greenhouse Gas Control. 2014;20:87–116.10.1016/j.ijggc.2013.10.019
- Sharma SD, Azzi M. A critical review of existing strategies for emission control in the monoethanolamine-based carbon capture process and some recommendations for improved strategies. Fuel. 2014;121:178–188.10.1016/j.fuel.2013.12.023
- Yang HQ, Xu ZH, Fan MH, et al. Progress in carbon dioxide separation and capture: a review. J Environ Sci. 2008;20:14–27.10.1016/S1001-0742(08)60002-9
- Li JR, Ma YG, McCarthy MC, et al. Carbon dioxide capture-related gas adsorption and separation in metal-organic frameworks. Coord Chem Rev. 2011;15–16:1791–1823.10.1016/j.ccr.2011.02.012
- Wang S, Li X, Wu H, et al. Advances in high permeability polymer-based membrane materials for CO2 separations. Energy Environ. Sci. 2016;9:1863–1890.10.1039/C6EE00811A
- Yampolskii Y, Pinnau I, Freeman BD. Materials science of membranes. West Sussex: Wiley; 2007.
- Kovvali AS, Sirkar KK. Dendrimer liquid membranes: CO2 separation from gas mixtures. Ind Eng Chem Res. 2001;40:2502–2511.10.1021/ie0010520
- Zou J, Ho WSW. CO2-selective polymeric membranes containing amines in crosslinked poly(vinyl alcohol). J Membr Sci. 2006;286:310–321.10.1016/j.memsci.2006.10.013
- Myers C, Pennline H, Luebke D, et al. High temperature separation of carbon dioxide/hydrogen mixtures using facilitated supported ionic liquid membranes. J Membr Sci. 2008;322:28–31.10.1016/j.memsci.2008.04.062
- Deng LY, Kim TJ, Hagg MB. Facilitated transport of CO2 in novel PVAm/PVA blend membrane. J Membr Sci. 2009;340:154–163.10.1016/j.memsci.2009.05.019
- Hussain A, Hagg MB. A feasibility study of CO2 capture from flue gas by a facilitated transport membrane. J Membr Sci. 2010;359:140–148.10.1016/j.memsci.2009.11.035
- Tong Z, Ho WSW. Facilitated transport membranes for CO2 separation and capture. Sep Purif Technol. 2017;52:156–167.
- Kovvali AS, Chen H, Sirkar K. Dendrimer membranes: a CO2-selective molecular gate. J Am Chem Soc. 2000;122:7594–7595.10.1021/ja0013071
- Taniguchi I, Duan S, Kazama S, et al. Facile fabrication of a novel high performance CO2 separation membrane: immobilization of poly(amidoamine) dendrimers in poly(ethylene glycol) networks. J Membr Sci. 2008;322:277–280.10.1016/j.memsci.2008.05.067
- Taniguchi I, Duan S, Kai T, et al. Effect of phase-separated structure on CO2 separation performance of poly(amidoamine) dendrimer immobilized in a poly(ethylene glycol) network. J Mater Chem A. 2013;1:14514–14523.10.1039/c3ta13711b
- Taniguchi I, Urai H, Kai T, et al. A CO2-selective molecular gate of poly(amidoamine) dendrimer immobilized in a poly(ethylene glycol) network. J Membr Sci. 2013;444:96–100.10.1016/j.memsci.2013.05.017
- Taniguchi I, Kai T, Duan S, et al. A compatible crosslinker for enhancement of CO2 capture of poly(amidoamine) dendrimer-containing polymeric membranes. J Membr Sci. 2015;475:175–183.10.1016/j.memsci.2014.10.015
- Duan S, Taniguchi I, Kai T, et al. Poly(amidoamine) dendrimer/poly(vinyl alcohol) hybrid membranes for CO2 capture. J Membr Sci. 2012;423–424:107–112.10.1016/j.memsci.2012.07.037
- Gaume J, Wong-Wah-Chung P, Rivaton A, et al. Photochemical behavior of PVA as an oxygen-barrier polymer for solar cell encapsulation. RSC Adv. 2011;1:1471–1481.10.1039/c1ra00350j
- Reynolds AJ, Verheyen TV, Adeloju SB, et al. Towards commercial scale postcombustion capture of CO2 with monoethanolamine solvent: key considerations for solvent management and environmental impacts. Environ Sci Technol. 2012;46:3643–3654.10.1021/es204051s
- Zhao M, Minett AI, Harris AT. A review of techno-economic models for the retrofitting of conventional pulverised-coal power plants for post-combustion capture (PCC) of CO2. Energy Environ Sci. 2013;6:25–40.10.1039/C2EE22890D
- Taniguchi I, Kazama S, Jinnai H. Structural analysis of poly(amidoamine) dendrimer immobilized in cross-linked poly(ethylene glycol). J Polym Sci B. 2012;50:1156–1164.10.1002/polb.v50.16
- Merkel TC, Lin H, Wei X, et al. Power plant post-combustion carbon dioxide capture: an opportunity for membranes. J Membr Sci. 2010;359:126–139.10.1016/j.memsci.2009.10.041
- Yamada H, Chowdhury FA, Goto K, et al. CO2 solubility and species distribution in aqueous solutions of 2-(isopropylamino)ethanol and its structural isomers. Int J Greenhouse Gas Control. 2013;17:99–105.10.1016/j.ijggc.2013.03.027
- Xie H-B, Johnson JK, Perry RJ, et al. A computational study of the heats of reaction of substituted monoethanolamine with CO2. J Phys Chem A. 2011;115:342–350.10.1021/jp1081627
- Kimura S, Honda K, Kitamura K, et al. Preliminary feasibility study for on-site hydrogen station with distributed CO2 capture and storage system. Energy Proc. 2014;63:4575–4584.10.1016/j.egypro.2014.11.490