ABSTRACT
Implant-associated infections are threatening and devastating complications that lead to bone destruction and loss. As a smooth surface is suitable for inhibiting bacterial adhesion, endowing antibacterial activity to the Ti surface without any structural changes in the surface topography is an effective strategy for preventing infection. The thin film on the Ti-6Al-4 V surface was functionalized to endow antibacterial activity by immersion in a Cu(OH)2 solution. The resulting surface maintains the surface topography with a surface roughness of 0.03 μm even after the immersion in the Cu(OH)2 solution. Moreover, Cu was detected at approximately 10 atom% from the surface and was present up to a depth of 30 nm of thin film. In vitro experiments revealed that the resulting surface exhibited antibacterial activity against methicillin-resistant Staphylococcus aureus and allowed the cellular proliferation, differentiation, and calcification of MC3T3-E1 cells. Furthermore, in vivo experiments determined that the presence of Cu in the thin film on the Ti-6Al-4 V surface led to no inflammatory reactions, including bone resorption. Thus, immersion in a Cu(OH)2 solution incorporates and immobilizes Cu into the thin film on the Ti-6Al-4 V surface without any structural alternations in the surface topography, and the resulting smooth surface exhibits antibacterial activity and osteogenic cell compatibility without cytotoxicity or inflammatory reactions. Our findings provide fundamental insights into the surface design of Ti-based medical devices, to achieve bone reconstruction and infection prevention.
IMPACT STATEMENT
Passivation of Ti-6Al-4V in Cu(OH)2 solution endowed smooth thin film with antibacterial activity and osteogenic cell compatibility for potentially achieving both bone reconstruction and infection prevention.
1. Introduction
Titanium (Ti) and its alloys are widely used in implant devices for bone reconstruction owing to their high mechanical strength, corrosion resistance, and biocompatibility [Citation1–3]. Implant-associated infections on Ti surfaces, such as peri-implantitis and prosthetic joint infections, are threatening and devastating complications that lead to bone destruction and loss of bone [Citation4–7]. Therefore, an antibacterial Ti surface that can inhibit bacterial growth is an effective strategy for preventing implant-associated infections [Citation8–10].
Surface topography is a key factor in preventing infection. For instance, a surface roughness of less than 0.088 μm can strongly inhibit bacterial adhesion [Citation11,Citation12]. In contrast, a higher surface roughness promotes bacterial adhesion by increasing the surface area and providing an additional niche for bacteria [Citation12,Citation13]. Therefore, a smooth surface with surface roughness below 0.088 μm is one of the most effective topographies for inhibiting bacterial adhesion. However, most surface treatments endow Ti with rough or porous surfaces that promoting osteogenesis [Citation14–21]. Moreover, in the case of peri-implantitis, rough and porous surfaces might cause infection around implants to develop more easily once these surfaces are exposed in the body [Citation22,Citation23]. In other words, although a smooth surface has no structural advantage in bone formation, it may be effective in preventing late infection and long-term implant success. Therefore, direct functionalization of the passive film on the Ti surface is required to impart antibacterial activity without sacrificing the structural advantages of the smooth surface in preventing infection.
The Ti surface is covered by a nanoscale passive film consisting of amorphous TiO2 and lower Ti oxides such as Ti2O3 and TiO [Citation24]. This film forms and immediately covers the Ti surface when the metallic Ti is exposed to the external environment. Furthermore, the ionic radii of Ti4+, Ti3+, and Ti2+ present in the passive film of Ti are 0.53–0.86 Å [Citation25]. Moreover, the passive film on the Ti surface forms hydroxyl groups on its top surfaces [Citation26,Citation27], and these hydroxyl groups dissociate to become negatively charged in alkaline solution [Citation27–29]. Therefore, cations can be incorporated into the passive film and immobilized through the hydroxyl groups when metallic Ti is exposed to an alkaline solution containing ionic radii similar to those of Ti ions.
Copper hydroxide (Cu(OH)2) is one of the antibacterial compounds that is poorly soluble in water (solubility: 1.3 × 10−5 mol·L−1) [Citation30–33], however it can be dissolved in trace amounts in aqueous solutions. The reaction rate increases depending on the temperature, and Cu(OH)2 efficiently dissolves in hot water. Additionally, hydroxide ions are released along with Cu ions during the dissolution of Cu(OH)2, leading to a weakly alkaline aqueous solution. Thus, Cu ions can be immobilized on the Ti surface through the hydroxyl groups when Ti is immersed in a Cu(OH)2 solution. Moreover, the ionic radii of copper (Cu) ions such as Cu+ and Cu2+ are 0.46–0.77 Å [Citation25]; therefore, Cu ions may be incorporated to the passive film of Ti via a passivation process in the Cu(OH)2 solution.
In the present study, the nano-scale thin film on the Ti-6Al-4 V surface was directly functionalized for realizing antibacterial activity without surface structural alterations through the immersion in the Cu(OH)2 solution at 80°C. The chemical state of Cu in the resulting thin films was characterized by X-ray photoelectron spectroscopy (XPS) and argon (Ar) ion sputtering. In vitro tests were performed to determine the antibacterial activity, cytotoxicity, and osteogenic cell compatibility of the resulting surfaces, and in vivo tests were performed to determine the presence or absence of an inflammatory response.
2. Materials and methods
2.1. Sample preparation
Ti-6Al-4 V alloy rods with a diameter of 10 mm were cut into disks with a thickness of 1.5 mm. The disk surfaces were mechanically mirror-polished using SiC paper with #320, #600, #800, #1000, #1200, #1500 and #2000 grids, and 0.06 μm-colloidal silica suspension. The mirror-polished samples were cleaned via ultrasonication in acetone and ethanol for 10 min. After the cleaning, the samples were immersed immediately in 0 and 10 mmol·L−1 Cu(OH)2 solution (5 mL/sample) at 80°C for 24 h. As Cu(OH)2 did not completely dissolve in the solution, the supernatant of the 10 mmol·L−1 Cu(OH)2 solution after centrifugation at 2600 × g for 10 min was used in this process. The Cu ions concentration in the supernatant was 10 μM. After immersion, the samples were cleaned via ultrasonication in ultrapure water for 30 min. The samples treated with 0 and 10 mM Cu(OH)2 solutions were labeled NT and Cu10, respectively. Pure Cu disks with diameters of 10 mm were used for in vivo evaluation.
2.2. Surface characterization
The arithmetic mean deviation of the surface roughness and topography of each sample were measured using a laser scanning confocal microscope (VK-9710, Keyence, Japan) (n = 3). The surface morphologies and compositions of NT and Cu10 were observed using field-emission scanning electron microscopy with energy-dispersive X-ray spectrometry (FESEM/EDS; JSM-7900F/JED-2300, JEOL, Tokyo, Japan). The water contact angles were measured by a contact angle meter (DM500, Kyowa Interface Science, Saitama, Japan) with a 2 µL per droplet for 1 s (n = 4). Surface chemical states and compositions of the NT and Cu10 were determined with X-ray photoelectron spectroscopy (XPS; JPS-9010MC, JEOL, Tokyo, Japan) with a Mg Kα X-ray source (1253.6 eV; 10 kV; 10 mA) (n = 3). The measurement chamber pressure was 1 × 10−7 Pa. The spectrometer was calibrated against Au 4f7/2 for pure Au, Ag 3d5/2 for pure Ag, and Cu 2p3/2 for pure Cu. The spectra were obtained at a pass energy of 10 eV, and the detection angle with respect to the sample surface was 90°. All the binding energies mentioned here are relative to the Fermi level and were calibrated with a C 1s photoelectron energy region peak derived from the contaminating carbon (285.0 eV). The chemical states of Cu on the NT and Cu10 were determined by the modified Auger parameter (α’) on the Wagner plot and based on those of typical Cu compounds. The α’ of each sample was calculated according to a method described previously [Citation34]. To calculate the integrated intensity of the peaks, the background was subtracted from the measured spectra using CasaXPS software (version 2.3.24, Casa Software Ltd., Teignmouth, United Kingdom) according to Shirley’s method [Citation35]. The composition of the sample was calculated by Casa XPS using the relative sensitivity factor (RSF) as follows: C 1s (1.00), O 1s (2.93), Ti 2p (7.81), and Cu 2p3/2 (16.73). The proportions of the (Cu0+Cu+) and Cu2+ species present on the surface were calculated using a method described previously [Citation36]. The depth profile of Cu10 was measured by alternating XPS analysis and Ar-ion sputtering. The sputtering rate was calibrated to be 30 nm·min−1 for SiO2 as reference.
2.3. In vitro evaluations
Prior to in vitro evaluation, all samples were sterilized in 70% ethanol and then thoroughly rinsed with deionized water. All sterilized samples were immersed in each medium overnight to prevent burst release of ions from the surface. Immersion took place in a physiological saline solution (0.9% NaCl) at 37°C for 24 h. Quantification of the Cu ions released from the samples (n = 4) was conducted using inductively coupled plasma-atomic emission spectrometry (ICP-AES; ICPE-9800; Shimadzu Corp., Kyoto, Japan).
The antibacterial activity of each sample was evaluated as in a previous study [Citation37]. The experiment was approved by the Pathogenic Organisms Safety Management Committee of the Tokyo Medical and Dental University (M22022–002). Methicillin-resistant Staphylococcus aureus (MRSA; ATCC 43,300) was cultured in tryptic soy broth (BD Bacto™, BD, U.S.A.) at 37°C for 24 h. The optical density of the bacterial suspension was measured at 595 nm using a CO8000 Biowave cell density meter (WPA Ltd., Cambridge, UK), with suspensions diluted to obtain concentrations of 2.6 × 107 colony-forming units (CFUs)·mL−1. Bacterial suspension drops measuring 16 μL were added, which were subsequently covered with a sterilized plastic film and incubated at 37°C for 24 h (n = 4). MRSA was then collected from all incubated samples, and viable bacteria were quantified by ATP using the BacTiter-GloTM microbial cell viability assay kit (Promega Corporation, Madison, WI, U.S.A.) and a luminometer (GloMax Navigator system, Promega) according to the manufacturer’s protocol. Bacterial viability was determined by normalizing the number of viable bacteria in the sample after 24 h of incubation with the initial seeding number.
As described in our previous study [Citation38], MC3T3-E1 cells (Riken BioResource Center, Ibaraki, Japan) were maintained in a cell culture medium which was an alpha modification of Eagle’s minimum essential medium (α-MEM; Gibco, Grand Island, NY, U.S.A.) supplemented with 10% fetal bovine serum (Gibco, U.S.A.), 100 U·mL−1 penicillin, 100 µg·mL−1 streptomycin, and 0.25 µg·mL−1 amphotericin B (Fujifilm Wako). The cells were incubated at 37°C in a humidified atmosphere containing 5% CO2. After incubation, cell suspensions were diluted to obtain concentrations of 1.0 × 104 cells·mL−1 and 1 mL of these cell suspensions were added to the samples. The number of viable cells in each sample after 1, 3, and 7 days of incubation was determined using a water-soluble tetrazolium salt (Cell Counting Kit-8, DOJINDO, Kumamoto, Japan) (n = 4) to reveal the proliferation behaviors. Cell viability is determined by normalizing the number of viable cells after 24 h of incubation with the initial seeding number. The cells in the samples after 7 days of incubation were fixed with 4% paraformaldehyde for 15 min. The actin and nuclei of attached cells were stained with Actistain 555 phalloidin (Cytoskeleton Inc., Denver, U.S.A.) and Hoechst 33,342 (Dojindo, Kumamoto, Japan), respectively. For alkaline phosphatase (ALP) activity and calcification test, cells were incubated in the culture medium supplemented with 10 mmol·L−1 β-glycerophosphate (Fujifilm Wako), 50 μg·mL−1 L-ascorbic acid (Fujifilm Wako), and 150 nmol·L−1 dexamethasone (Fujifilm Wako) to induce osteogenic differentiation. The medium was changed every 3 days. After 7 days of incubation, the ALP activity in the cells on the samples was evaluated using the LabAssay™ ALP method (Fujifilm Wako) as per the manufacturer’s protocol (n = 4). After 21 days of incubation, the calcified area on each sample surface was stained with Alizarin Red S (ARS; Sigma-Aldrich, St. Louis, MO, U.S.A.) (n = 4). The percentages of ARS-stained areas on NT and Cu10 were measured using the image J software.
2.4. In vivo evaluations
Animal experiments were approved by the Animal Care and Use Committee of Kyushu University (no. A23-265-1). The six male Wister rats (12 weeks of age) were housed at the Center of Biomedical Research, Research Center for Human Disease Modeling, Graduate School of Medical Sciences, Kyushu University. Rats were fed a standard diet with ad libitum access to food and water. The rats were anesthetized with 2.5% isoflurane. The skin of the calvaria was cut, and NT, Cu10, and pure Cu disks were implanted in contact with the periosteum. After healing for 4 weeks, the animals were euthanized, the implanted calvaria were collected, and soft tissue and samples were removed. The implanted calvaria were subsequently fixed in a 10% formalin neutral buffer solution to perform the histological evaluation. For histological evaluation, the specimens were first decalcified with 0.5 mol‧L−1 of ethylenediaminetetraacetic acid. After decalcification, the specimens were embedded in paraffin and sliced into 3-µm-thick pieces. Hematoxylin and eosin (HE)-stained sections were prepared after deparaffinization. The histological evaluation of H&E-stained specimens was performed using a BZ-X710 microscope and digital analysis software (BZ-X, Keyence, Osaka, Japan) to reveal the presence and absence of inflammatory reaction caused by implantation of the sample.
2.5. Statistical analysis
Statistical analyses were performed using Kaleida Graph software version 4.1.1 (Synergy Software, Eden Prairie, MN, U.S.A.). Student’s t-test was performed for two-group comparisons, and a one-way analysis of variance with the Student – Newman – Keuls multiple-comparison test was performed for comparisons between more than two groups. Statistical significance was set at p < 0.05 this study.
3. Results and discussion
A smooth surface with approximately 0.03 mm of surface roughness was confirmed from each sample (). Thus, smooth topography was maintained even following immersion in the Cu(OH)2 solution at 80°C, indicating the corrosion reaction that changed the surface topography did not occur on the Ti-6Al-4 V surface. Moreover, the water contact angle of Cu10 is significantly higher than that of NT (). Therefore, immersion in the Cu(OH)2 solution increases the water contact angle of the Ti-6Al-4 V surface. The SEM images revealed that there were no precipitates on Cu10, similar to NT (). Moreover, Ti, Al, and V were detected, while Cu was not detected on each sample surface, indicating that the concentration of Cu on Cu10 was lower than the detection limit of EDS.
Figure 1. 3D laser microscopy images and surface roughness (a), quantification and optical images of water contact angles (b), scanning electron microscopy images and Ti, Al, V, O, and Cu mappings (c) obtained from NT and Cu10.
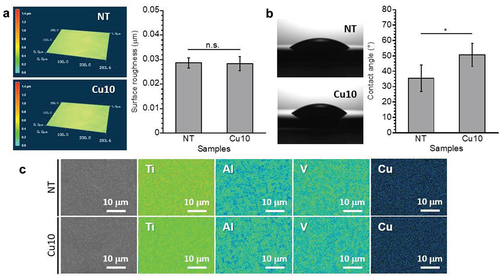
From the XPS analysis, peaks located in the C 1s, Ti 2p, and O 1s energy regions were detected on each sample surface (). Additionally, peaks located around Cu 2p and Cu LMM were detected only for Cu10 (). This indicates that Cu was present only on the Cu10 surface. The binding energy of Cu 2p3/2 photoelectron peak and the kinetics energy of Cu LMM Auger electron peak were 934.5 ± 0.1 eV and 916.8 ± 0.2 eV, respectively (). Furthermore, the α‘ value calculated from the binding energy of Cu 2p3/2 photoelectron peak and the kinetics energy of Cu LMM Auger electron peak was 1851.2 ± 0.3 eV (). This indicates that Cu mainly exists as Cu2+ on the Cu10 surface [Citation34]. The surface concentration of Cu on Cu10 was 10.0 ± 0.3 atom%. The proportion of (Cu0 + Cu+) and Cu2+ on Cu10 were 11.8 ± 1.7% and 88.2 ± 1.7%, respectively (). Thus, the proportion of the Cu species was consistent with the results of the chemical state analysis of Cu. The XPS depth profile revealed that the intensity of the peak attributed to Ti4+ decreased, whereas that of Ti0 increased with sputtering time (). Additionally, a Cu 2p peak was detected even after sputtering for 60 s, which disappeared after 120 s (). It is considered that the slight amount of Cu exists up to the depth of around 30 nm as the sputtering rate was 30 nm·min−1. Thus, the film thickness of Cu10 was thicker than that formed by 24 h of immersion in ultrapure water [Citation39], indicating that the 24 h of immersion in Cu(OH)2 solution at 80°C promoted surface oxidation. Moreover, the intensity of the Cu 2p peak was highest before sputtering (). The O 1s spectra obtained from NT and Cu10 were composed of three peaks (Figure S1), originating from O2−, OH−, and H2O [Citation39]. The area percentage of OH− in Cu10 was higher than that of NT (Table S1), indicating that the OH group was more present in Cu10. In contrast, the water contact angle in Cu10 was higher than that in NT (). These results indicate that the Cu ions were immobilized on the surface hydroxyl groups of Ti-6Al-4 V. Moreover, precipitates were not detected on the Cu10 surface in the 3D laser microscopy and SEM observations (. Thus, the Cu ions were incorporated into the thin film and immobilized on the surface hydroxyl groups without any surface structural alterations.
Figure 2. X-ray photoelectron spectroscopy (XPS) survey scan spectra obtained from NT and Cu10 (a), Cu 2p (b), Cu LMM (c) energy region spectra and the Wagner plot of Cu (D) obtained from Cu10.
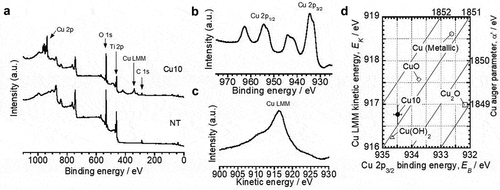
Figure 3. The proportion of Cu species calculated from Cu 2p energy region spectrum of Cu10 (a). Ti 2p (b) and Cu 2p (c) energy region spectra obtained from Cu10 before and after sputtering during 0 to 120 s.
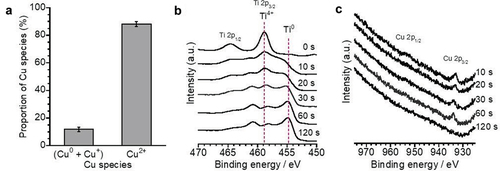
To determine the Cu ion release behavior of the samples, NT, Cu10, and pure Cu were immersed in physiological saline for 24 h. As shown in , the amount of Cu ions released from each sample increased in the order of pure Cu > Cu10. No Cu ions were detected in the NT group. Therefore, Cu10 is thought to release fewer Cu ions than pure Cu in vivo. The antibacterial activities and cytotoxicities of NT, Cu10, and pure Cu were evaluated in MRSA and MC3T3-E1 cells. The viability of MRSA in NT group was higher than 100% (), indicating MRSA proliferated on NT after 24 h of incubation. In contrast, the viabilities of MRSA on Cu10 and pure Cu groups were lower than 100% (), indicating MRSA was killed on these sample surfaces after the 24 h of incubation. The viabilities of MRSA on Cu10 and pure Cu were significantly lower than that on NT. This indicates that the presence of Cu in the thin film on Cu10 resulted in antibacterial activity against MRSA, similar to that of pure Cu. In this study, the release of Cu ions from Cu10 was detected, implying that the antibacterial activity of Cu10 could have primarily contributed to by Cu ions releasing from the surface. Furthermore, since Cu releases Cu ions caused by the contact effect between bacteria and Cu, it is thought that antibacterial activity was also expressed through contact killing [Citation34]. In other words, Cu is possibly immobilized through hydroxyl groups and Cu in the thin film, as revealed by surface characterization, exerts antibacterial activity through different mechanisms. Moreover, the viability of MC3T3-E1 cells on NT and Cu10 groups was at the same level and was higher than 100% (), indicating that MC3T3-E1 cells proliferated on NT and Cu10 after 24 h of incubation. In contrast, the viability of MC3T3-E1 cells on the pure Cu was less than 100%, indicating MC3T3-E1 cells were killed on the pure Cu surface after the 24 h of incubation. Thus, antibacterial activity and cytocompatibility were achieved only with Cu10. NT showed cytocompatibility without antibacterial activity, and pure Cu showed antibacterial activity with cytotoxicity.
Figure 4. The amount of Cu ions released from the NT, Cu10 and pure Cu into physiological saline during the 24 h of immersion (a). The viability of MRSA (b) and MC3T3-E1 cells (c) on the NT, Cu10 and pure Cu during 24 h of incubation.
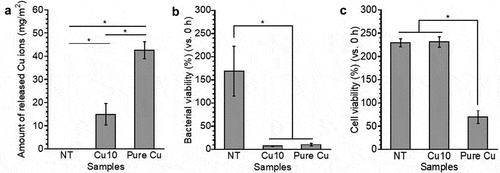
Cytocompatibility was observed for NT and Cu10; therefore, these samples were used for in vitro evaluations of bone formation. The proliferation, differentiation, and calcification behaviors of MC3T3-E1 cells on NT and Cu10 were evaluated. After 1, 3, and 7 d of incubation, the cell densities of MC3T3-E1 cells on Cu10 and NT increased over time and were at the same level between the samples in all incubation periods (). After 7 d of incubation, most of the NT and Cu10 were covered with MC3T3-E1 cells (). These results indicate that MC3T3-E1 cells proliferated on Cu10, similar to that on NT.
Figure 5. Cellular proliferation of MC3T3-E1 cells on NT and Cu10 after 1, 3, and 7 days of incubation (a). Fluorescence images of the MC3T3-E1 cells attached to the NT and Cu10 after 7 days of incubation (b).
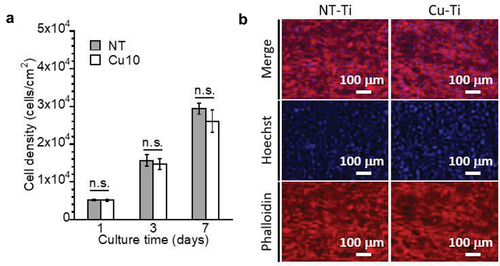
After 7 days of incubation, the ALP activity of MC3T3-E1 cells on NT and Cu10 was at the same level (), indicating that approximately 10 atom% of Cu on Cu10 did not affect the early osteoblast differentiation [Citation40]. After 21 days of incubation, the calcified area on each sample was stained with ARS and quantified as the area percentage (). There was no significant difference between the percentages of ARS-stained areas on NT and Cu10, indicating that the cells were calcified on Cu10, similar to those on NT. Thus, the in vitro experiments revealed that Cu10 had no effect on the proliferation, differentiation, or calcification of MC3T3-E1 cells.
Figure 6. Alkaline phosphatase (ALP) activity levels in MC3T3-E1 cells on the samples after 7 days of incubation (a). The area percentage of the alizarin red S (ARS) stained area on the samples after 21 days of incubation, and the optical microscope images of ARS-stained samples (b).
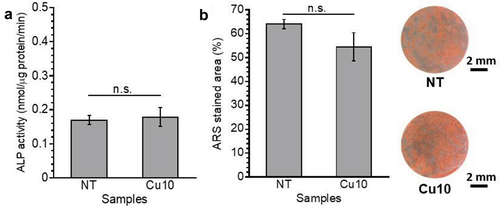
To determine the biological responses to NT, Cu10, and pure Cu, in vivo experiments were performed using experimental animals. NT, Cu10, and pure Cu were implanted in contact with the periosteum on rat calvaria, and the presence or absence of an inflammatory response in each sample was determined from the HE-stained sections prepared from each group (). No inflammatory reaction was observed in the NT and Cu10 groups, and the periosteum and bone tissues were observed (. Moreover, osteocytes were detected and the bone tissue showed a lamellar structure (. Thus, the presence of Cu in the thin film did not affect the bone tissue. In contrast, fibrous tissue infiltrated the bone, indicating that bone resorption occurred in the pure Cu group (. The results of our in vivo experiments using pure Cu are in agreement with those of a previous study using different animal models. Trindade et al. implanted pure Cu into rabbit tibia and reported bone resorption and fibrous tissue penetration after a healing period of 10 days of healing period [Citation41]. In general, Cu is toxic to living tissues and cells in a concentration-dependent manner [Citation42], which limits its direct application in biomaterials [Citation43]. In contrast, the Cu10 group showed a biological response similar to that of the NT group, meaning no adverse effects were observed. Thus, the presence of Cu in the thin film and the Cu ions released from Cu10 did not affect the in vitro and in vivo bone formation. To date, a suitable amount of Cu on a biomaterial surface has achieved simultaneous antibacterial and osteogenic activities [Citation44–46]. Thus, immersion in the Cu(OH)2 solution endowed the Ti-6Al-4 V surface with antibacterial and osteogenic activities owing to the incorporation and immobilization of appropriate amounts of Cu on the Ti-6Al-4 V surface.
Figure 7. Hematoxylin-eosin-stained sections 4 weeks after the implantation of NT (a, d), Cu10 (b, e), and pure Cu (c, f). (d – f) magnified views of the regions indicated by black squares in panels (a – c).
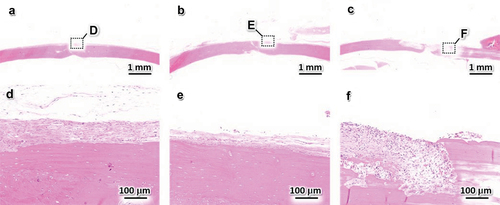
Immersion in the Cu(OH)2 solution endowed the Ti-6Al-4 V surface with antibacterial activity and osteogenic cell compatibility without any structural alteration of the surface topography. The antibacterial activity of Cu10 was attenuated after autoclaving (Figure S2). However, Cu10 suppressed MRSA growth compared to NT, indicating that the limited antibacterial activity of Cu10 will continue. Further studies are required to reveal and realize durable antibacterial activities. Moreover, our animal experiments did not reveal bone formation on Cu10; therefore, Cu10 should be implanted into the bone tissue in future studies.
In this study, Cu was incorporated and immobilized on a Ti-6Al-4 V surface via passivation in a Cu(OH)2 solution. Immersion in the Cu(OH)2 solution retained the surface topography because it did not lead to either the precipitation of Cu or the corrosion reaction that changed the surface topography. The resulting surface exhibited an antibacterial activity similar to that of pure Cu and allowed cellular proliferation, differentiation, and calcification similar to that of NT. Moreover, Cu10 did not induce inflammatory reactions such as bone resorption; thus, it simultaneously exhibits antibacterial activity and osteogenic cell compatibility. Our findings will be useful for the surface design of metallic biomaterials via a simple surface functionalization process without structural alterations of the surface topography and can provide novel medical devices for the reconstruction of bone tissue and prevention of infection.
4. Conclusion
Herein, Cu was incorporated into the thin film on the Ti-6Al-4 V surface based on the similar ionic radii of copper and titanium ions, and was immobilized through surface hydroxide groups on the thin film on the Ti-6Al-4 V surface. Surface characterizations revealed that immersion in the Cu(OH)2 solution did not affect the surface topography. The XPS analysis revealed that approximately 10 atom% of Cu was detected, and Cu was present until a depth of 30 nm of thin film. Moreover, in vitro and in vivo experiments revealed that the Cu-incorporated thin films exhibited both antibacterial activity and osteogenic cell compatibility without cytotoxicity or inflammatory reactions. Thus, the passivation process in the Cu(OH)2 solution endowed the Ti-6Al-4 V surface with antibacterial and osteogenic activities. Our findings provide fundamental insights into the surface design of Ti-based medical devices to achieve bone reconstruction and infection prevention.
Supplemental Material
Download PDF (45 KB)Disclosure statement
No potential conflict of interest was reported by the author(s).
Supplementary material
Supplemental data for this article can be accessed online at https://doi.org/10.1080/14686996.2024.2303327
Additional information
Funding
References
- Hanawa T. Titanium–tissue interface reaction and its control with surface treatment. Front Bioeng Biotechnol. 2019;7:170. doi: 10.3389/fbioe.2019.00170
- Kaur M, Singh K. Review on titanium and titanium based alloys as biomaterials for orthopaedic applications. Mater Sci Eng C. 2019;102:844–9. doi: 10.1016/j.msec.2019.04.064
- RP V. Titanium based biomaterial for bone implants: a mini review. Mater Today Proc. 2020;26:3148–3151. doi: 10.1016/j.matpr.2020.02.649
- Schwarz F, Derks J, Monje A, et al. Peri-implantitis. J Clin Periodontol. 2018;45(S20):S246–S266. doi: 10.1111/jcpe.12954
- Kapadia BH, Berg RA, Daley JA, et al. Periprosthetic joint infection. Lancet. 2016;387(10016):386–394. doi: 10.1016/S0140-6736(14)61798-0
- Ahmadabadi HY, Yu K, Kizhakkedathu JN. Surface modification approaches for prevention of implant associated infections. Colloids Surf B Biointerfaces. 2020;193:111116. doi: 10.1016/j.colsurfb.2020.111116
- Zimmerli WJ. Clinical presentation and treatment of orthopaedic implant-associated infection. J Intern Med. 2014;276(2):111–119. doi: 10.1111/joim.12233
- Chouirfa H, Bouloussa H, Migonney V, et al. Review of titanium surface modification techniques and coatings for antibacterial applications. Acta Biomater. 2019;83:37–54. doi: 10.1016/j.actbio.2018.10.036
- Shimabukuro M. Antibacterial property and biocompatibility of silver, copper, and zinc in titanium dioxide layers incorporated by one-step micro-arc oxidation: a review. Antibiotics. 2020;9(10):716. doi: 10.3390/antibiotics9100716
- Souza JGS, Bertolini MM, Costa RC, et al. Targeting implant-associated infections: titanium surface loaded with antimicrobial. IScience. 2021;24(1):102008. doi: 10.1016/j.isci.2020.102008
- Rimondini L, Farè S, Brambilla E, et al. The effect of surface roughness on early in vivo plaque colonization on titanium. J Periodontol. 1997;68(6):556–562. doi: 10.1902/jop.1997.68.6.556
- Dhaliwal JS, Rahman NA, Knights J, et al. The effect of different surface topographies of titanium implants on bacterial biofilm: a systematic review. Sci. 2019;1(6):1–16. doi: 10.1007/s42452-019-0638-6
- Al-Ahmad A, Wiedmann-Al-Ahmad M, Fackler A, et al. In vivo study of the initial bacterial adhesion on different implant materials. Arch Oral Biol. 2013;58(9):1139–1147. doi: 10.1016/j.archoralbio.2013.04.011
- Gil J, Pérez R, Herrero-Climent M, et al. Benefits of residual aluminum oxide for sand blasting titanium dental implants: osseointegration and bactericidal effects. Materials. 2021;15(1):178. doi: 10.3390/ma15010178
- Liao PB, Cheng HC, Huang CF, et al. The cell culture of titanium alloy surface modifications by micro-powder blasting and co-blast techniques. Surf Eng. 2019;35(7):643–651. doi: 10.1080/02670844.2019.1587570
- Dong S, Zhao T, Wu W, et al. Sandblasted/acid-etched titanium surface modified with calcium phytate enhances bone regeneration in a high-glucose microenvironment by regulating reactive oxygen species and cell senescence. Sci Eng. 2023;9(8):4720–4734. doi: 10.1021/acsbiomaterials.3c00385
- Ramaglia L, Postiglione L, Di Spigna G, et al. Dent Mater J. 2011; 30:183–192.
- Nishiguchi S, Nakamura T, Kobayashi M. The effect of heat treatment on bone-bonding ability of alkali-treated titanium. Biomaterials. 1999;20(5):491–500. doi: 10.1016/S0142-9612(98)90203-4
- Kawashita M, Iwabuchi Y, Suzuki K, et al. Surface structure and in vitro apatite-forming ability of titanium doped with various metals. Colloids Surf A. 2018;555:558–564. doi: 10.1016/j.colsurfa.2018.07.027
- Shimabukuro M, Kobayashi M, Kawashita M. Metallic substrate influences on the osteogenic cell compatibility and antibacterial activity of silver-incorporated porous oxide layers formed by micro-arc oxidation. ACS Appl Eng Mater. 2023;1(8):2288–2294. doi: 10.1021/acsaenm.3c00332
- Shen X, Fang K, Ru Yie KHR, et al. Bioact Mater. 2022;10:405–419. doi:10.1016/j.bioactmat.2021.08.031.
- Teughels W, Van Assche N, Sliepen I, et al. Effect of material characteristics and/or surface topography on biofilm development. Clin Oral Implants Res. 2006;17(S2):68–81. doi: 10.1111/j.1600-0501.2006.01353.x
- Dank A, Aartman IH, Wismeijer D, et al. Effect of dental implant surface roughness in patients with a history of periodontal disease: a systematic review and meta-analysis. Int J Implant Dent. 2019;5(1):1–11. doi: 10.1186/s40729-019-0156-8
- Pouilleau J, Devilliers D, Garrido F, et al. Structure and composition of passive titanium oxide films. Mat Sci Eng B. 1997;47(3):235–243. doi: 10.1016/S0921-5107(97)00043-3
- Shannon RD, CT P. Effective ionic radii in oxides and fluorides. Acta Crystallogr B Struct Sci. 1969;25(5):925–946. doi: 10.1107/S0567740869003220
- Hanawa T. Biocompatibility of titanium from the viewpoint of its surface. Sci Tech Adv Mate. 2022;23(1):457–472. doi: 10.1080/14686996.2022.2106156
- Boehm HP. Angew Chem Int Ed Engl. 1966;5(6):533–544. doi: 10.1002/anie.196605331
- Parfitt GD. The surface of titanium dioxide. In: Progress in surface and membrane science. Vol. 11. Elsevier; 1976. p. 181–226. https://www.sciencedirect.com/science/article/abs/pii/B9780125718110500091.
- Boehm HP. Acidic and basic properties of hydroxylated metal oxide surfaces. Faraday Soc. 1971;52:264–275. doi: 10.1039/df9715200264
- Akhavan O, Azimirad R, Safa S, et al. CuO/Cu(OH)2 hierarchical nanostructures as bactericidal photocatalysts. J Mater Chem. 2011;21(26):9634–9640. doi: 10.1039/c0jm04364h
- Phan DN, Dorjjugder N, Saito Y, et al. Antibacterial mechanisms of various copper species incorporated in polymeric nanofibers against bacteria. Mater Today Commun. 2020;25:101377. doi: 10.1016/j.mtcomm.2020.101377
- Chen Y, Sun R, Yan W, et al. Sci Total Environ. 2022;817:152897. doi:10.1016/j.scitotenv.2021.152897.
- Cudennec Y, Lecerf ASSS. The transformation of Cu(OH)2 into CuO, revisited. Solid State Sci. 2003;5(11–12):1471–1474. doi: 10.1016/j.solidstatesciences.2003.09.009
- Shimabukuro M, Manaka T, Tsutsumi Y, et al. Corrosion behavior and bacterial viability on different surface states of copper. Mater Trans. 2020;61(6):1143–1148. doi: 10.2320/matertrans.MT-M2020008
- DA S. High-resolution X-Ray photoemission spectrum of the valence bands of gold. Phys Rev B. 1972;5(12):4709–4714. doi: 10.1103/PhysRevB.5.4709
- Biesinger MC. Advanced analysis of copper X-ray photoelectron spectra. Surf Interface Anal. 2017;49(13):1325–1334. doi: 10.1002/sia.6239
- Shimabukuro M, Tsutsumi Y, Yamada R, et al. Investigation of realizing both antibacterial property and osteogenic cell compatibility on titanium surface by simple electrochemical treatment. Sci Eng. 2019;5(11):5623–5630. doi: 10.1021/acsbiomaterials.8b01058
- Shimabukuro M, Hayashi K, Kishida R, et al. No-observed-effect level of silver phosphate in carbonate apatite artificial bone on initial bone regeneration. ACS Infect Dis. 2022;8(1):159–169. doi: 10.1021/acsinfecdis.1c00480
- Shimabukuro M, Ito H, Tsutsumi Y, et al. The effects of various metallic surfaces on cellular and bacterial adhesion. Metals. 2019;9(11):1145. doi: 10.3390/met9111145
- Harris H. The human alkaline phosphatases: what we know and what we don’t know. Clinica Chimica Acta. 1990;186(2):133–150. doi: 10.1016/0009-8981(90)90031-M
- Trindade R, Albrektsson T, Galli S, et al. Bone immune response to materials, part I: titanium, PEEK and copper in comparison to sham at 10 days in Rabbit Tibia. J Clin Med. 2018;7(12):526. doi: 10.3390/jcm7120526
- Scheiber I, Dringen R, Mercer JF. Copper: effects of deficiency and overload. Interrelations between essential metal ions and human diseases. 2013;359–387.
- Wang Y, Zhang W, Yao Q. Copper-based biomaterials for bone and cartilage tissue engineering. J Orthop Transl. 2021;29:60–71. doi: 10.1016/j.jot.2021.03.003
- Shimabukuro M, Hayashi K, Kishida R, et al. Surface functionalization with copper endows carbonate apatite honeycomb scaffold with antibacterial, proangiogenic, and pro-osteogenic activities. Biomater Adv. 2022;135:212751. doi: 10.1016/j.bioadv.2022.212751
- Shimabukuro M, Tsutsumi Y, Nozaki K, et al. Investigation of antibacterial effect of copper introduced titanium surface by electrochemical treatment against facultative anaerobic bacteria. Dent Mater J. 2020;39(4):639–647. doi: 10.4012/dmj.2019-178
- Aoki S, Shimabukuro M, Kishida R, et al. Electrochemical Deposition of Copper on Bioactive Porous Titanium Dioxide Layer: antibacterial and pro-osteogenic activities. ACS Appl Bio Mater. 2023;6(12):5759–5767. doi: 10.1021/acsabm.3c00860