ABSTRACT
The ever-growing use of nature-derived materials creates exciting opportunities for novel development in various therapeutic biomedical applications. Living cells, serving as the foundation of nanoarchitectonics, exhibit remarkable capabilities that enable the development of bioinspired and biomimetic systems, which will be explored in this review. To understand the foundation of this development, we first revisited the anatomy of cells to explore the characteristics of the building blocks of life that is relevant. Interestingly, animal cells have amazing capabilities due to the inherent functionalities in each specialized cell type. Notably, the versatility of cell membranes allows red blood cells and neutrophils’ membranes to cloak inorganic nanoparticles that would naturally be eliminated by the immune system. This underscores how cell membranes facilitate interactions with the surroundings through recognition, targeting, signalling, exchange, and cargo attachment. The functionality of cell membrane-coated nanoparticles can be tailored and improved by strategically engineering the membrane, selecting from a variety of cell membranes with known distinct inherent properties. On the other hand, plant cells exhibit remarkable capabilities for synthesizing various nanoparticles. They play a role in the synthesis of metal, carbon-based, and polymer nanoparticles, used for applications such as antimicrobials or antioxidants. One of the versatile components in plant cells is found in the photosynthetic system, particularly the thylakoid, and the pigment chlorophyll. While there are challenges in consistently synthesizing these remarkable nanoparticles derived from nature, this exploration begins to unveil the endless possibilities in nanoarchitectonics research.
IMPACT STATEMENT
We have highlighted the Cell-derived Nanomaterials for Biomedical Applications through the lenses of our team who have experiences with working on cell membrane, thylakoids, and studying the impact of nanoparticles on biological phenomenon such as nanomaterialsinduced endothelial leakiness (NanoEL). In this review, we have discussed the progress and the wide potential of nanoarchitectonics in plant systems, animal cells and microorganisms. Due to our unique backgrounds, our take on this topic may be the novelty of the review.
1. Introduction
Living cells of animals and plants possess remarkable capabilities to function as natural nanofactories, synthesizing and secreting various nanoparticles (NPs) and nanocomposites, making them great candidates for nanoarchitectonics [Citation1]. These cellular processes have inspired scientists and researchers to explore and harness the potential of biological systems for the production of nanomaterials with unique properties and applications [Citation2,Citation3]. Remarkably, animal cells, plant cells and microorganisms have amazing capabilities due to the inherent functionalities in each specialized cell type. They can become the NPs themselves, or support and enhance the process of NP production in a more sustainable or effective way. In the recent years, there has been an increase in nanoarchitectonics development, hence this review will highlight the interesting progress and the future of various technologies.
1.1. Living cells as nanofactories – the foundation of nanoarchitectonics
The concept of living cells as nanofactories stems from the intricate molecular machinery and biochemical pathways that exist within cells. Cells have evolved sophisticated mechanisms to control and regulate the synthesis and assembly of nanoscale structures. These processes often involve the utilization of biological macromolecules, such as proteins, enzymes, and nucleic acids, which act as building blocks or catalysts for the formation of NPs [Citation4,Citation5]. In the realm of animals, certain specialized cells, such as immune cells, have the ability to produce NPs as part of their defense mechanisms. For instance, macrophages can synthesize and release iron oxide NPs to sequester and neutralize pathogens. Similarly, some organisms, like diatoms and radiolarians, possess the inherent ability to create intricate silica-based nanoscale structures, forming intricate skeletal frameworks [Citation6]. Plants, on the other hand, have evolved fascinating mechanisms to synthesize and secrete various NPs and nanocomposites. For example, many plant species can produce phytoliths, which are silica NPs formed within their cells [Citation7]. These phytoliths contribute to the structural integrity of plants and provide protection against herbivores and pathogens. Additionally, plants can also produce metal NPs, such as gold and silver, through the utilization of specialized enzymes and phytochemicals [Citation8].
The unique advantage of using living cells as nanofactories lies in their ability to create nanomaterials with precise control over size, shape, composition, and functionality. By leveraging the inherent capabilities of cells, researchers can explore sustainable and environmentally friendly approaches for the synthesis of NPs and nanocomposites, which are briefly summarised in . Furthermore, the use of living cells allows for the integration of biological components and functionalities into the nanomaterials, enabling the development of bioinspired and biomimetic systems with enhanced properties and novel applications. The field of synthetic biology has emerged as a powerful tool to engineer living cells to produce nanomaterials. By modifying the genetic makeup of cells or introducing specific genes, researchers can enhance or redirect cellular processes to synthesize desired nanomaterials. This approach offers great potential for tailoring nanomaterial properties, expanding the range of materials that can be produced, and exploring new applications in fields such as drug delivery, sensing, catalysis, and energy conversion [Citation9–11].
Figure 1. Cell-derived nanomaterials and their applications. This paper will further focus on cell membranes from animals, and photosynthetic systems which involves chloroplast and thylakoid [Citation12–15].
![Figure 1. Cell-derived nanomaterials and their applications. This paper will further focus on cell membranes from animals, and photosynthetic systems which involves chloroplast and thylakoid [Citation12–15].](/cms/asset/198b7d14-05a7-4335-8541-5c40d0ef148e/tsta_a_2315013_f0001_oc.jpg)
The concept of living cells as natural nanofactories highlights the fascinating abilities of biological systems to synthesize and secrete NPs and their nanocomposites. Understanding and harnessing these natural processes can lead to the development of innovative and sustainable approaches to creating nanomaterials with diverse properties and applications. This interdisciplinary field holds promise for advancing nanotechnology and unlocking new possibilities in various sectors, including healthcare, materials science, and environmental sustainability.
1.2. Breakdown of the cell anatomy
To comprehend how cell components can be reinvented for nanoarchitectonics through extraction and assembly of cell components, it is essential to explore the fundamental building blocks of their respective organisms. This is applicable in both animal and plant cells as they are multicellular eukaryotes with very intricate structures called organelles as shown in . The presence of various organelles is mainly to support the compartmentalisation of functions. This separation of functions allows greater efficiency and control over processes such as energy production, protein synthesis, and waste disposal [Citation16–18]. While they share many similarities in structure and function, they also exhibit several key differences, largely due to their specific roles and requirements within their respective organisms [Citation19].
There are several organelles that are present in both animal and plant cells. The organelle that forms a barrier and acts as the gatekeeper of both cells is the cell membrane. It consists of two layers of phospholipids, with cholesterol and protein channels embedded in a mosaic manner, allowing dynamic movements of components embedded on the membrane. The cell membrane is semi-permeable which allows specific molecules to cross the lipid bilayers via osmosis, down concentration gradient via protein channels, and active transport [Citation20]. The compositions and specific functions of the cell membrane change with cell type. Thus, it is a suitable candidate to be employed and transferred to NPs through nanoarchitectonics, which will be discussed in Section 3. Moving into the interior of the cell is a gel-like substance called cytoplasm, where many cellular processes occur as it is the medium for chemical reactions. It acts as a platform for organelles to operate within the cell, where cell growth, expansion, and replication are carried out [Citation21,Citation22]. The genetic material in both plant and animal cells is housed in the nucleus. This organelle contains all the deoxyribonucleic acid (DNA) which controls all cell activity by regulating the gene expression. This enables the proper functioning of each cell. Inside the nucleus is a spherical structure called nucleolus which produces and assembles the ribosomes and transcribes ribosomal ribonucleic acid (rRNA) genes. All cells contain membrane-bound organelles, including mitochondria, endoplasmic reticulum (ER), Golgi apparatus, and ribosomes. The mitochondria are the powerhouses of the cell as they are responsible for generating energy through cellular respiration. Oxidative phosphorylation occurs in the mitochondria where energy is released from the food consumed and the energy produced is stored in adenosine triphosphate (ATP). ATP is then used as the primary energy source for biochemical, biological, and physiological processes [Citation23]. There are two types of ER, the smooth ER and the rough ER. The rough ER has ribosomes attached to the outer surfaces of the ER making it visually rougher and is more abundant in cells that specialise in producing proteins. The smooth ER supports the production of lipids and steroid hormones. The Golgi apparatus performs modifications, sorts, and packages proteins and lipids for transport within the cell or to be secreted outside of it. Finally, the ribosome is the site of protein synthesis where the ribosomes read the messenger ribonucleic acid (mRNA) sequences and translate the genetic code which determines the arrangement of amino acid chains which would be folded into proteins.
After examining organelle similarities in both animal and plant cells, the distinctive organelles of these cell types will be explored. Animal cells possess two specific organelles, centrioles and lysosomes, which are absent from plant cells [Citation22]. Centrioles exist as a pair and are involved in cell division during mitosis and meiosis. To allow smooth and accurate cell division, their main role is to organize microtubules within the cell, to attach and pull chromosomes to opposite ends during cell division, and thus organize the locations of the organelles before the cell divides. Lysosomes, membrane-bound organelles filled with digestive enzymes, break down cellular waste, including worn-out or faulty cell parts, as well as foreign materials like invading viruses and bacteria. On the other hand, organelles that are exclusively present in plant cells are the cell wall, vacuole, and chloroplast. The rigid and primarily cellulose-based cell wall provides structural support and protection for the cell and the entire plant. Plant cells often have a large vacuole, which plays a crucial role in maintaining the turgidity of the cell at the optimal pressure, water storage, and storing various substances produced or taken up, including pigments and toxins. Chloroplast, enclosed by a double membrane called chloroplast envelope, is responsible for photosynthesis, converting sunlight into energy and producing glucose and oxygen as byproducts. Within the double membrane exists a third internal membrane called the thylakoid membrane. This structure optimizes the biochemical reactions, ensuring that all the necessary substrates and enzymes are present. This organelle can also adapt to changes in the environment such as changes to the intensity and quality of light, ensuring optimal photosynthesis. The unique abilities of chloroplast and thylakoid to be involved in nanoarchitectonics are further explored in Section 5.
The presence of organelles is one of the defining characteristics of animal and plant cells, and it plays a crucial role in the complexity and functionality of these cells. Organelles are specialized, membrane-bound compartments within eukaryotic cells, each with specific functions and roles. They are highly specialized, and different organelles compartmentalize various cellular functions. This separation of functions allows for greater efficiency and control over processes such as energy production, protein synthesis, and waste disposal. With the presence of organelles, each component provides a controlled environment for specific biochemical reactions. This optimization ensures that reactions occur in the right place and time, with the necessary substrates and enzymes present. Therefore, there is undiscovered potential for incorporating components and membranes of organelles into the nanoarchitectonics design.
1.3. Advantages of living cells synthesizing nanomaterials
Using living cells as nanofactories for nanomaterial production offers several advantages over traditional synthetic methods. The key advantages are biocompatibility, precision, complexity, sustainability, environmentally friendly properties, integration of biological functionality, versatility, diversity, and potential for dynamic and adaptive systems [Citation15,Citation24–26]. Living cells naturally have a high degree of biocompatibility, making them a reasonable choice for biomedical applications. Thus, nanomaterials produced by living cells are inherently compatible with biological systems and are less likely to trigger immune responses or toxicity issues. This biocompatibility is crucial for applications such as drug delivery systems, tissue engineering scaffolds, and implantable devices [Citation26]. Moreover, living cells have evolved complex molecular machinery that allows precise control over the synthesis of nanomaterials. The cellular processes involved in nanomaterial production, such as enzymatic reactions and self-assembly, enable cells to create nanomaterials with specific sizes, shapes, compositions, and functionality [Citation27,Citation28]. This level of precision is challenging to achieve using traditional synthetic methods. Furthermore, the use of living cells for nanomaterial production offers a more sustainable and environmentally friendly approach. Cells can utilize renewable resources and energy sources, reducing the reliance on non-renewable materials and minimizing the generation of waste. Additionally, the production of nanomaterials within cells often occurs under mild conditions, minimizing the use of harsh chemicals and energy-intensive processes. Living cells can incorporate biological functionalities into the synthesized nanomaterials. This integration allows for the development of bioinspired and biomimetic materials that can perform specific functions or interact with biological systems in a tailored manner. For example, incorporating specific proteins or enzymes into nanomaterials can enable enzymatic reactions, targeting capabilities, or responsive behavior. Living cells offer a wide range of options for nanomaterial production due to the diversity of biological systems. Different cell types, organisms, and their genetic modifications can be employed to produce a variety of nanomaterials with distinct properties. This versatility allows for the synthesis of nanomaterials with diverse compositions, structures, and functionalities, expanding the range of applications in fields such as medicine, electronics, energy, and environmental remediation. Furthermore, living cells possess inherent dynamic and adaptive properties that can be leveraged for nanomaterial production. Cells can respond to environmental cues, self-repair, or undergo genetic modifications to adapt and optimize the production of nanomaterials. This adaptability opens possibilities for the development of responsive and self-regulating nanomaterials that can adapt to changing conditions or stimuli. While using living cells as nanofactories offers significant advantages, there are also challenges associated with scalability, control, and standardization. However, ongoing advancements in synthetic biology, genetic engineering, and bioprocessing techniques are addressing these challenges and paving the way for the translation of cell-based nanomaterial production into practical applications.
Overall, harnessing the capabilities of living cells for nanomaterial production provides a promising avenue for the development of advanced nanotechnologies, with the potential to revolutionize fields such as medicine, materials science, and environmental sustainability.
1.4. Mechanisms of nanoparticle synthesis in living cells
Living cells employ various mechanisms for nanoparticle synthesis, which can be broadly categorized into intracellular biosynthesis and extracellular biosynthesis. In intracellular biosynthesis, NPs are synthesized within the cytoplasm or organelles of living cells [Citation29]. This process often relies on the utilization of enzymes, proteins, and other biomolecules present within the cells. Intracellular biosynthesis can occur through different mechanisms, such as enzymatic reduction, biomineralization, and intracellular assembly. Some cells possess enzymes capable of reducing metal ions to form metallic NPs. For instance, silver NPs can be synthesized by reducing silver ions using enzymes like nitrate reductase or reductases present in certain bacteria or plant cells [Citation30]. In biomineralization, cells control the formation of NPs by directing the precipitation of inorganic materials. For example, diatoms and radiolarians can produce intricate silica-based nanoscale structures within specialized compartments called silica deposition vesicles [Citation31]. Cells can synthesize NPs through intracellular assembly where biomolecules act as building blocks. For instance, ferritin, a protein involved in iron storage, can assemble and store iron oxide NPs within its protein shell [Citation32].
In extracellular biosynthesis, NPs are synthesized outside the cells [Citation33]. Cells release specific biomolecules that participate in the synthesis and assembly of NPs in the extracellular environment. Some mechanisms of extracellular biosynthesis include microbial extracellular synthesis and plant-mediated synthesis. Certain microorganisms, such as fungi and bacteria, can secrete metabolites or enzymes that mediate the formation of NPs [Citation30]. These metabolites or enzymes interact with precursor compounds to induce nanoparticle nucleation and growth. For example, some bacteria produce extracellular enzymes that convert metal ions into metallic NPs [Citation34]. Plants can also facilitate the extracellular synthesis of NPs. They release phytochemicals and specialized enzymes that induce the reduction and stabilization of metal ions, leading to the formation of NPs [Citation35]. Plant extracts have been used extensively in green synthesis approaches for nanoparticle production [Citation35–38].
Amino acids are enantiomeric and are one of the important naturally occurring chiral molecular groups. The recognition of L- and D-enantiomers of amino acids has been crucial in uncovering the mechanisms of life sciences and is used for the modulation of biological systems [Citation39]. During the growth of MoS2 nanosheets, thiol amino acids with chiral properties were able to modulate the asymmetric growth of gold on Au/MoS2 heterostructures. Eventually, this resulted in the nanostructure having the ability to have enantioselective recognition, synthesizing chiral dendritic heterostructures, and having a controlled drug release system all in one [Citation40].
Once the NPs are formed, they may interact with living cells, undergo cellular uptake, and subsequently traffic within the intracellular environment. The cellular uptake mechanisms and intracellular trafficking pathways depend on factors such as nanoparticle properties, cell types, and endocytic pathways. Some common mechanisms include endocytosis, intracellular trafficking, and exocytosis. NPs can be internalized by cells through various endocytic pathways, such as phagocytosis, caveolae-mediated endocytosis, clathrin-mediated endocytosis, or macropinocytosis [Citation41]. These processes involve the invagination of the cell membrane to form endocytic vesicles containing the NPs. Following endocytosis, NPs may undergo intracellular trafficking within the cells. This involves interactions with various cellular compartments, such as endosomes, lysosomes, or specific organelles, depending on the nanoparticle type and cell type. Trafficking pathways can influence nanoparticle stability, degradation, or release from cells. In some cases, cells can actively transport NPs out of the cytoplasm through exocytosis. This process involves the fusion of intracellular vesicles containing NPs with the cell membrane, leading to the release of NPs into the extracellular environment. It is paramount to note that the cellular uptake and intracellular trafficking mechanisms can vary significantly depending on factors such as nanoparticle size, surface properties, surface modifications, and the specific cell type involved. Thus, understanding these mechanisms is crucial for designing nanomaterials with desired cellular interactions, targeted delivery, and controlled release.
In summary, living cells utilize intracellular and extracellular biosynthesis mechanisms to synthesize NPs. These NPs can interact with cells, undergo cellular uptake through various endocytic pathways, traffic within the intracellular environment, and may eventually be released from cells through exocytosis. Studying these processes helps in developing strategies for nanoparticle synthesis, cellular targeting, and biomedical applications.
2. Animal cell-derived nanoparticles
2.1. Animal cell lines for nanoparticle production
Various types of animal cell lines have been employed for nanoparticle production, each offering distinct advantages and limitations. Here are some commonly used cell lines and their characteristics including mammalian cell lines, insect cell lines, and marine organisms.
Mammalian cell lines, such as HeLa cells, the first immortal human cell lines cultured, or Chinese hamster ovary (CHO) cells, are extensively employed in the field of nanoparticle synthesis [Citation42,Citation43]. Mammalian cells demonstrate a high degree of compatibility with human biology, rendering them suitable for various biomedical applications. Mammalian cells possess the necessary machinery to perform intricate post-translational modifications on proteins. This capability enables the production of functionalized NPs with specific properties. Additionally, mammalian cell lines exhibit elevated levels of protein expression, which proves advantageous in the synthesis of protein-based NPs. Nevertheless, it is important to acknowledge that mammalian cell lines also have certain limitations, including, higher cost and complexity, lower growth rates, and genetic stability. The maintenance of mammalian cell cultures can incur greater expenses and demand more technical expertise compared to other cell lines. Mammalian cells generally display slower growth rates in comparison to other cell lines. This characteristic can have an impact on the overall productivity of nanoparticle synthesis. Some mammalian cell lines may exhibit genetic instability, resulting in variations in nanoparticle production over time.
Insect cell lines, particularly derived from the Spodoptera frugiperda (Sf9) or High Five (Trichoplusia ni) cell lines, are commonly used in conjunction with the baculovirus expression system for nanoparticle production [Citation44,Citation45]. Insect cells can produce high levels of recombinant proteins, including viral coat proteins used for nanoparticle synthesis. Moreover, insect cell culture can be easily scaled up to large volumes, facilitating industrial production. Additionally, the baculovirus expression system allows for the efficient production of recombinant proteins and the assembly of viral NPs. However, insect cell lines have certain limitations. Insect cells may not always perform the same post-translational modifications as mammalian cells, which can affect the functionality and properties of certain NPs. Although insect cells are useful for various applications, their compatibility with human biology may be limited for certain biomedical purposes.
Marine organisms, such as diatoms and radiolarians, offer unique advantages for nanoparticle synthesis [Citation46,Citation47]. These organisms naturally produce intricate silica-based nanoscale structures. Marine organisms provide a natural source of NPs, such as silica-based structures, which can be utilized or modified for specific applications. In addition, the marine environment hosts a vast array of organisms, offering a wide range of nanoparticle structures and properties. However, working with marine organisms for nanoparticle synthesis also presents challenges, such as limited scalability, and difficulties in extraction and purification. Cultivating marine organisms can be more challenging and less scalable compared to traditional cell lines. Extracting and purifying NPs from marine organisms can be complex and time-consuming. The choice of cell line for nanoparticle synthesis depends on the specific requirements of the intended application, where nanoparticle composition, desired properties, scalability, biocompatibility, and cost come into consideration.
In summary, mammalian cell lines offer biocompatibility and post-translational modifications, insect cell lines provide high protein expression and scalability, and marine organisms offer natural nanoparticle synthesis. Understanding the advantages and limitations of each cell line aids researchers in selecting the most suitable system for nanoparticle production based on their specific goals and requirements.
2.2. Nanoparticles synthesized by animal cells
Animal cells have the capacity to synthesize various types of NPs, including metal NPs, quantum dots, lipid-based and protein-based NPs. These NPs are produced through intricate biochemical and cellular processes within animal cells [Citation48]. Here is an overview of the different types of NPs synthesized by animal cells and the underlying mechanisms involved in metal NPs, quantum dots, and lipid-based NPs.
Animal cells can synthesize metal NPs through processes similar to those observed in plant cells. The underlying mechanisms include: (i) Enzymatic Reduction: Animal cells produce enzymes, such as reductases, which catalyze the reduction of metal ions into metal NPs [Citation8]. These enzymes utilize cofactors like NADPH to facilitate the reduction reaction. (ii) Biomolecule Interaction: Biomolecules within animal cells, including proteins, peptides, and small molecules, interact with metal ions and act as reducing agents and stabilizers, resulting in the formation of metal NPs. (iii) Intracellular and Extracellular Synthesis: Metal NPs can be synthesized both inside and outside animal cells. Intracellular synthesis can occur within organelles such as the endoplasmic reticulum or Golgi apparatus, while extracellular synthesis can take place on the cell surface or within extracellular vesicles [Citation49].
Quantum dots are semiconductor NPs with unique electronic and optical properties. Animal cells can synthesize quantum dots through different processes [Citation5]. (i) Cellular uptake of precursors: Animal cells can take up precursor molecules, such as heavy metal ions and semiconductor precursors, from the extracellular environment via various transport mechanisms. (ii) Intracellular processing: Once inside the cell, these precursor molecules undergo specific intracellular processing steps, including ligand exchange, nucleation, and growth processes, leading to the formation of quantum dots. (iii) Surface modification: Quantum dots synthesized within animal cells can be further modified with biomolecules or surface coatings to enhance their stability, biocompatibility, and targeting properties. Through biomineralization-assisted bottom-up strategy, mercapto group from cysteine protein sources, such as bovine serum albumin, was able to fabricate high-density defects in quantum dots in the form of blue photoluminescent molybdenum sulfide (MoS2) quantum dots [Citation50]. This defect engineering strategy demonstrates the potential of another parameter to control material properties in quantum dots. Additionally, a biodegradable cobalt sulfide quantum dot was engineered to be a sulfur defect and proved to be a powerful chemodynamic therapy against cancer [Citation51]. It has improved photothermal and hyperthermal abilities which gave rise to having selective therapeutic efficiency and with low side effects.
Animal cells can also produce lipid-based NPs, such as liposomes and exosomes, through complex cellular processes. For instance, animal cells can remodel their cellular membranes via membrane remodeling, allowing the formation of lipid bilayers or lipid vesicles. This process involves the controlled rearrangement of lipids and membrane proteins. Moreover, it has reported the encapsulation of cargo assay [Citation52]. Lipid-based NPs, such as liposomes or exosomes, can encapsulate therapeutic molecules, nucleic acids, or imaging agents within their lipid bilayers or luminal compartments. Through another process involving specific biogenesis and secretion pathways, animal cells can produce exosomes. This process begins with endosomal membranes budding inwards, resulting in the formation of multivesicular bodies that release exosomes upon fusion with the plasma membrane.
The underlying biochemical and cellular processes involved in nanoparticle synthesis within animal cells are complex and highly regulated. Enzymatic reactions, biomolecule interactions, cellular uptake, intracellular processing, membrane remodeling, and biogenesis pathways play crucial roles in the formation and assembly of NPs. Understanding these processes allows for the optimization of nanoparticle production, surface modification, cargo loading, and the development of targeted delivery systems for various biomedical applications.
2.3. Importance of vasculatures and endothelial cells interaction with nanoparticles
Most NPs will eventually enter the bloodstream in three common ways. First by ingestion through food and water we consume, which eventually enter the gastrointestinal (GI) tract and are absorbed into the bloodstream [Citation53]. Second, when injected as drugs directly into the bloodstream. Third, inhaled through the respiratory system which then cleared from the respiratory tract via the mucociliary escalator, which then enters the GI tract. When NPs are within the bloodstream, they will circulate the body through the vasculature, interact with endothelial cells, circulate, and migrate to other tissues and organs. The endothelial lining of blood vasculatures results in a high surface area for substances such as naturally occurring nutrients, cytokines, and NPs to exchange between the vasculature content and the surrounding tissues [Citation54,Citation55]. Therefore, the vasculature is considered a dynamic cellular ‘organ’ as the endothelium also regulates the blood flow and the trafficking of leukocytes during inflammation. As such, endothelial cells play a crucial role in nanoarchitectonics as they are involved in disease detection through endothelium imaging, tumor diagnosis, cancer treatment, and gene therapy which are further enhanced with NPs. The contributions of nanoarchitectonics, when coupled with, will enhance non-invasive disease detection methods for diseases such as cancer and Parkinson’s [Citation56].
To achieve malignant proliferation, solid tumors are able to hijack the nutrients in blood by stimulating the local angiogenesis, producing abnormal vessels with leaky characteristics known as the enhanced permeability and retention (EPR) effect [Citation57]. This results in selective extravasation and retention of macromolecular drugs, thus making EPR the ‘“gold standard”’ in drug designing to target tumors [Citation58]. However, the EPR effect faces several limitations such as its dependence on mature tumors and its heterogeneous nature [Citation59]. Therefore, the EPR effect should be further built on to extend the targeting to young tumors which would not present any EPR effect. Nevertheless, it is still paramount to target the tumor-related endothelium since microvascular endothelial cells are spread all over the body, allowing the closest contact interface for NPs circulating in the blood to achieve deep tissue targeting [Citation60]. For instance, molecular markers of disease that exist on the surface of the endothelium can be targeted by NPs decorated with corresponding antibodies. NPs engineered can have the ability to do magnetic resonance imaging or X-ray, computed tomography, and high sensitivity of positron emission tomography. Cancer mass was reported to produce pro-inflammatory factors which subsequently stimulate endothelial cells expressing VCAM, ICAM, PECAM, and E- and P-selectin on their luminal side [Citation60]. Therefore, it is possible to modify NPs to have suitable antibodies of tumor markers on the surface of endothelium through nanoarchitectonics, which can target and image the microvasculature of tumor mass [Citation61].
2.4. Interactions of nanoparticles with vasculatures in animal cells
In recent years, synthetic NPs have been found to give antagonistic and protagonistic effects in the vasculature of normal and diseased blood vessels, and this study was called the nanomaterial-induced endothelial leakiness (NanoEL) effect [Citation59,Citation62]. Leakiness of endothelial cells could be an outcome of various diseases such as inflammation, cancer, atherosclerosis and acute respiratory distress syndrome [Citation63]. Based on the nanoparticle design, it has the ability to induce direct pathological effects such as disruption of cell-cell junctions resulting in the promotion of cancer metastasis or onset of various invasive infections. It could also induce secondary pathological effects such as perturbed intracellular cell signaling, oxidative stress, and ultimately cell death, resulting in changes in molecular transport across the vascular walls and edema. On the other hand, this can also result in therapeutic effects with proper designing of NPs. This is because NPs can be designed to have desired physicochemical properties to make the leakiness predictable and tunable, promoting leakiness only where it is needed, and also increasing target specificity, showing great potential in potential drug delivery for cancer treatment. Gold NPs were able to tune the leakiness of tumoral vascular leakiness by disrupting the endothelial adherens junction [Citation64]. Remarkably, the endothelial cells did not display any perceivable toxicity in this process.
By coupling gold NPs with liposomal doxorubicin (Lipo-Dox) therapy, the efficacy of the therapy increased as compared to treatment with free Dox, as the implanted mice tumor had decreased in size and even became undetectable after a period of treatment. To further understand the phenomenon behind cancer treatment through NanoEL, Wang et. al discovered that besides interacting with the endothelial vessels, the interaction with tumor vascular basement membrane (BM) plays an important role as this membrane is another physiological barrier for NPs to overcome before accessing the tumoral environment [Citation65]. After crossing the endothelial vessels, NPs would form NP pools in the subendothelial space, followed by a pool eruption which promotes extravasation of NPs into the tumoral environment. To control and enhance NP extravasation into tumors, local hyperthermia therapy (LHT) could promote the formation of NP pools on the subendothelial region, by disrupting the vascular endothelial (VE)-cadherin. The opened BM barriers encourage neutrophil infiltration, which leads to the aggressive explosion of trapped NPs from the NP pools, thus resulting in effective and deep penetration into the tumors. In summary, there has been an ongoing growth in the potential of applying endothelial leakiness in therapeutic applications. In fact, this leakiness can be induced in a myriad of modalities, including synthetic and nature-derived NPs, heat, ultrasound, photodynamic therapy, and radiotherapy [Citation66]. By purposefully inducing endothelial leakiness coupled with a better understanding of the mechanisms governing it, strategy for nanomedicine design could become safer, with lower potential side effects.
2.5. Tuning endothelial leakiness with nature-derived nanoparticles
Besides lipid-based NPs, animal cells have a great capacity to produce proteins when a particular sequence of DNA is transcribed to mRNA, and then the mRNA is translated into a protein chain with the help of ribosomes. Employing this mechanism to create desired proteins has been incorporated in mRNA-based vaccines, which were widely used to combat severe acute respiratory syndrome coronavirus 2 (SARS-CoV-2). The desired spike protein found on the virus’s outer membrane corresponding to SARS-Cov-2 was created by the cells when the person was inoculated with the corresponding mRNA vaccine. In contrast, human cells also created proteins that are of interest in studying their advantages and disadvantages physiologically. The aggregation of amyloid beta (Aβ) has been identified as the potential cause of Alzheimer’s disease (AD), a major neurodegeneration disease that is impacting the aging population worldwide. AD patients are observed to have increased deposition of Aβ in the brain, even though the protein exists naturally throughout the body and has hormone storage as one of its biological functions [Citation67]. In a recent on-going investigation, our team investigated the pathological functions of Aβ and found similarities to the nanomaterials-induced endothelial leakiness (NanoEL). Together with collaborators, we found that even non-synthetic NPs such as Aβ protein were able to interact with VE-cadherin located in the blood vessels in the blood-brain barrier (BBB), leading to transient gaps being formed thus making the BBB leaky thus resulting in the accumulation of Aβ protein in the brain [Citation68]. Although Aβ in the brain is not healthy, due to its inherent NanoEL behavior, it could be incorporated into treatment where naturally-occurring materials is favored for NanoEL effect than synthetic NPs made from gold or titanium.
Liu et. al. discovered a strategy to potentially treat pulmonary arterial hypertension, which is associated with endothelial leakiness [Citation69]. Her team proposed a strategy that would attenuate endothelial leakiness in the pulmonary vasculatures to slow down the progression of pulmonary vascular remodeling. The main cause of the disease was the upregulation of protein Atg101, which is pro-autophagic and leads to apoptosis of endothelial cells causing leakiness. Therefore, the strategy was to use functionalized DNA nanostructures that can knock out Atg101. Remarkably, the DNA nanostructures that were conjugated with siAtg101 and aptamer were found to have high selectivity to diseased pulmonary endothelial cells. This resulted in high efficacy for the treatment of pulmonary arterial hypertension. This demonstrates that nucleic acids can have synergistic characteristics when forming complexes to support the delivery of drugs and other chemical cargo [Citation4]. This particular study provided a glimpse into how nanomaterials such as DNA nanostructures have enhanced the targeting efficacy, and incorporating the knowledge of endothelial leakiness opens a window into the future of nature-derived nanomaterials that target diseased endothelial cells of various causes.
3. Animal cell membrane
As an essential component of all living cells, the cell membrane serves many functions such as protecting components of the cell from outside, regulating the transport of materials between the extracellular matrix and the cell, and interacting with the surrounding cells and organisms. To achieve these functions, the cell membrane contains a plethora of biomolecules such as proteins, lipids, and carbohydrates [Citation70]. The composition and specific functions of the cell membrane differ depending on the cell type. Some functions of the cell membrane can be transferred to NPs by combining the NPs with the cell membrane. The diversity of cell membranes provides a rich and versatile toolbox for nanoarchitectonics. A brief summary of the different cell types used as cell membrane source and their functions are given in .
Table 1. Different cell types as a cell membrane source and their functions.
One of the early explored functions of the cell membrane was its ability to cloak inorganic NPs, which would otherwise have been eliminated quickly by the immune system. For this purpose, the red blood cell (RBC) membrane was first tested [Citation71]. Shielding inorganic NPs from the immune system by enveloping them with RBC membranes proved successful in prolonging the circulation time of these NPs in the bloodstream [Citation71,Citation72]. Longer circulation in the bloodstream and stronger immune evasion capability of the RBC-cloaked NPs were demonstrated in the study with macrophage cell culture [Citation73]. Significant decreases in the number of RBC membrane-coated NPs taken up by the macrophage cells were reported. Following this initial discovery, other cell membranes, such as platelets and leukocytes, were shown to have similar immune evasion capabilities with the added function of targeting [Citation74,Citation75].
Cell membrane facilitates interactions with surrounding cells including recognition, signaling, targeting, attachment, and exchange of cargo, by expressing some cell-specific biomolecules such as intrinsic and extrinsic proteins and glycolipids [Citation79,Citation80]. The targeting function has been widely studied for drug delivery and targeted therapy applications, such as the homotypic targeting ability of the cancer cell membrane. Zhu et al. demonstrated that even in the presence of two different tumors, NPs accumulated at the tumor that matched the cell membrane coating on the nanoparticle [Citation78]. By changing the cell membrane coating on the NPs, authors were able to dictate which treatment to deliver to which tumor. This demonstration endorsed the versatility of the cell membrane coating technology. Other cell membrane types have also been shown to target disease sites via heterotypic targeting. For example, the cell membrane of the mesenchymal stem cells was used to construct a nanostructure to treat prostate cancer and the macrophage cell membrane was employed for targeting the lung metastasis of breast cancer [Citation52,Citation77].
In addition to targeting specific cell types, cell membrane coating can be utilized for its ability to interact with biomolecules that are found in the extracellular matrix. This function of the cell membrane might be useful for the treatment of diseases that are strongly associated with inflammatory conditions. One such example was the use of neutrophil cell membrane-coated PLGA NPs (neutrophil-NPs) for the treatment of rheumatoid arthritis [Citation9]. Neutrophil-NPs were able to infiltrate deeper into the inflamed cartilage in comparison to those coated with RBC membrane. Neutrophil-NPs could neutralize the inflammatory factors and provide effective chondroprotection against joint damage. The effect of specific cell membrane coating was demonstrated in another study in which the authors compared differently polarized macrophage cell membranes from the same macrophage source for their ability to absorb inflammatory cytokines and facilitate the recovery of osteoarthritis [Citation76]. It was found that the cell membrane of the M2 macrophage with anti-inflammatory properties could sponge up the TNF-α and IL1-β cytokines much more effectively than the cell membranes of native M0 macrophages and pro-inflammatory M1 macrophages. Effective removal of these cytokines alleviated osteoarthritis inflammation and facilitated matrix regeneration and recovery.
The functionality of cell membrane coating can be further enhanced by the strategic engineering of the membrane. One way of engineering cell membranes is by inserting selected biomolecules into the membrane. For example, the insertion of folate or the nucleolin-targeting aptamer AS1411 into the RBC membrane enabled the targeting of different cancer cells by the RBC-coated NPs [Citation81]. Another method of engineering cell membranes is by combining selected cell membranes into one to create a hybrid membrane. By doing so, unique functions of different cell membranes can be collected in a single membrane. In a study done by Dehaini et al. the successful construction of a hybrid membrane was demonstrated using RBC and platelet (PL) membranes () [Citation82]. In a follow-up study, this hybrid membrane was used as a surface coating to endow a nanorobot with the capability of neutralizing various toxins and removing pathogens [Citation83]. In another study, the above-mentioned cell membrane engineering methods were combined to create magnetic nanobeads for the capture of circulating tumor cells (CTCs) [Citation84]. For this construct, the platelet membrane was first fused with the leukocyte membrane. Then the hybrid membrane was further functionalized with CTC-targeting antibodies. While the hybrid membrane coating provided reduced interaction with white blood cells, incorporated antibodies enabled enhanced capture of CTCs, increasing captured cell purity from 66% to 96%.
Figure 3. Schematic of membrane fusion of red blood cell (RBC) and platelet membranes to produce RBC-platelet hybrid membrane-coated NPs. Reproduced from [Citation82]. With permission from John Wiley and Sons.
![Figure 3. Schematic of membrane fusion of red blood cell (RBC) and platelet membranes to produce RBC-platelet hybrid membrane-coated NPs. Reproduced from [Citation82]. With permission from John Wiley and Sons.](/cms/asset/87daa84e-7cae-49b5-9486-eff1b7ed9da3/tsta_a_2315013_f0003_oc.jpg)
Besides biological considerations such as which cell membrane to choose, from a nanoarchitectonics perspective, other factors may affect the success of the nanoconstruct. Physical properties such as size, shape, and degree of cell membrane coverage are factors explored in the literature. The size of the RBC-coated NPs did not have a significant effect on the in vitro macrophage uptake and in vivo immune response [Citation85]. However, it was found that with increasing size, the circulation time of the NPs was decreased, and liver accumulation was increased. The effect of shape on the targeting efficiency of the cancer cell membrane NPs was demonstrated using spherical and flattened constructs [Citation86]. It was shown that the flattened shape can target the tumor faster and achieve better treatment efficacy due to increased contact area between the NPs and the target cells (). Another physical factor that was explored was the cell membrane coverage efficacy. NPs with various degrees of cell membrane coating coverage were prepared and the cell uptake mechanisms were investigated [Citation87]. The NPs with a cell membrane coating degree of 50% or more were considered high coating degree, while a coating degree of 20–50% was considered a low coating degree. The NPs with a cell membrane coating degree of less than 20% were labelled as very low coating degree. Through comprehensive experimentation and theoretical modeling, the authors concluded that NPs with high cell membrane coating degrees could be uptaken individually (). On the other hand, NPs with low coating degree first need to aggregate into groups and these small chunks of NPs are uptaken into cells all at once. The NPs with very low coating degrees are thought to have been uptaken by the cells only if they aggregate together with the higher coating degree NPs.
Figure 4. (a) Illustration of the difference of surface interaction between differently shaped cell membrane nanoparticles. Reproduced from Ref [Citation86], with permission from the American Chemical Society. b) The possible endocytic entry mechanism for nanoparticles with different degrees of coating. Reproduced from Ref [Citation87], with permission from Springer Nature.
![Figure 4. (a) Illustration of the difference of surface interaction between differently shaped cell membrane nanoparticles. Reproduced from Ref [Citation86], with permission from the American Chemical Society. b) The possible endocytic entry mechanism for nanoparticles with different degrees of coating. Reproduced from Ref [Citation87], with permission from Springer Nature.](/cms/asset/e510163b-60f3-4321-ae2a-1431aa272e0e/tsta_a_2315013_f0004_oc.jpg)
4. Plant cell-derived nanomaterials
4.1. Plant cell cultures for nanoparticle production
Plant cell cultures, such as hairy root cultures and suspension cultures, have emerged as promising platforms for nanoparticle production. These cultures involve the growth of plant cells in a controlled environment, allowing for the synthesis of various types of NPs. Here is an overview of the use of plant cell cultures for nanoparticle production and their advantages in terms of scalability, sustainability, and cost-effectiveness.
Hairy root cultures involve the induction of adventitious roots in plants using Agrobacterium rhizogenes. These cultures offer unique advantages for nanoparticle production such as the green synthesis of silver nanoparticles [Citation10]. (i) Secondary Metabolite Production: Hairy root cultures can produce a wide range of secondary metabolites, including polyphenols, alkaloids, and flavonoids. These metabolites can act as precursors or stabilizers for nanoparticle synthesis. (ii) Genetic Stability: Hairy root cultures exhibit genetic stability, allowing for consistent nanoparticle production over time. This stability is advantageous for long-term and large-scale nanoparticle synthesis. (iii) Enhanced Nutrient Uptake: Hairy roots have a high surface area-to-volume ratio, enabling efficient nutrient uptake and utilization. This promotes cell growth and enhances nanoparticle production. (iv) Sustainability and Cost-Effectiveness: Hairy root cultures offer sustainability benefits similar to suspension cultures, as they can be maintained in bioreactors without the need for extensive land or resources. Moreover, the scalability and cost-effectiveness of hairy root cultures make them attractive for large-scale nanoparticle production [Citation10].
Suspension cultures involve the cultivation of plant cells in a liquid medium which is typically performed in bioreactors [Citation88]. They offer several advantages for nanoparticle production. (i) Scalability: Suspension cultures can be easily scaled up to large volumes, making them suitable for industrial-scale nanoparticle production. Bioreactors can be employed to provide controlled growth conditions, nutrient supply, and continuous monitoring. (ii) High Biomass Yield: Plant suspension cultures can achieve high biomass yields, resulting in a substantial number of cells available for nanoparticle synthesis. This enables efficient production of NPs at a larger scale. (iii) Genetic Manipulation: Plant cells in suspension cultures can be genetically modified to enhance nanoparticle synthesis or introduce specific functionalities [Citation89]. This flexibility allows for tailoring NPs to meet specific requirements. (iv) Sustainable and Cost-Effective: Plant cell cultures are sustainable as they provide a continuous supply of cells without the need for land, water, or other resources required for traditional agriculture. Additionally, plant cell cultures can be more cost-effective compared to other cell culture systems.
The advantages of plant cell cultures lie in their scalability, sustainability, and cost-effectiveness. These systems provide a continuous supply of cells, offer genetic manipulation possibilities, and can achieve high biomass yields. Moreover, plant cell cultures can be cultivated in controlled environments, reducing the dependence on external factors, and ensuring consistent nanoparticle production. The sustainable and cost-effective nature of plant cell cultures makes them attractive for industrial applications, where large quantities of NPs are required for various fields, including medicine, agriculture, and environmental remediation.
4.2. Types of nanoparticles synthesized by plant cells
Plant cells have the remarkable ability to synthesize various types of NPs, including metal NPs, carbon-based NPs, and polymer NPs. These NPs are produced through complex biochemical and metabolic pathways within plant cells. Here is an overview of the different types of NPs synthesized by plant cells and the underlying mechanisms involved.
Plant cells can synthesize metal NPs through a process called bioreduction [Citation90,Citation91]. This involves the conversion of metal ions present in the environment into their metallic form by plant metabolites. For instance, plant cells produce enzymes, such as reductases, that can catalyze the reduction of metal ions into metal NPs. These enzymes utilize cofactors, such as NADPH, to facilitate the reduction reaction. Moreover, phytochemicals, such as flavonoids, terpenes, and phenolic compounds, along with various biomolecules like proteins and polysaccharides, act as reducing and stabilizing agents. They interact with metal ions, facilitating their reduction and subsequent stabilization as NPs [Citation92]. In addition, metal NPs can be synthesized both intracellularly and extracellularly within plant cells. Intracellular synthesis occurs within specific compartments, such as vacuoles or endoplasmic reticulum, while extracellular synthesis takes place in the apoplast or on the cell surface.
Plant cells can also produce carbon-based NPs, including carbon dots and carbon nanotubes [Citation93,Citation94]. The mechanisms of carbon-based nanoparticle synthesis involve pyrolysis, carbonization, and biomolecule functionalization. Plant cells possess carbon-rich compounds, such as sugars, cellulose, lignin, and proteins, which can undergo pyrolysis or carbonization processes under controlled conditions. These processes result in the formation of carbon-based NPs. In addition, plant-derived biomolecules, such as proteins, polysaccharides, and phenolic compounds, can serve as precursors or templates for the synthesis of carbon-based NPs. Functionalization of these biomolecules leads to the formation of carbon NPs with unique properties for bioimaging, and antimicrobial and antioxidant capabilities [Citation93]. Furthermore, nanoporous carbon materials, utilized in supercapacitor applications, can be derived from plants like lotus seed and Terminalia chebula seed, contributing to the advancement of clean energy storage [Citation95,Citation96].
Plant cells can synthesize polymer NPs through the metabolism and polymerization of specific monomers [Citation97]. For instance, plant cells possess metabolic pathways that produce monomers, such as phenolic compounds, flavonoids, and terpenes. These monomers can undergo enzymatic or non-enzymatic polymerization reactions within plant cells, resulting in the formation of polymer NPs. Furthermore, some plant-derived polymers have the ability to self-assemble or undergo coacervation, forming NPs. This process involves the spontaneous aggregation of polymer chains driven by factors like pH, temperature, or ionic strength.
The specific metabolic pathways and mechanisms involved in nanoparticle synthesis within plant cells are still being explored and vary depending on the nanoparticle type. Plant cells utilize their enzymatic machinery, phytochemicals, biomolecules, and metabolic pathways to facilitate the reduction, carbonization, or polymerization of precursor materials, resulting in the formation of NPs. Understanding these underlying mechanisms is crucial for harnessing plant cell-based nanoparticle synthesis for various applications, including medicine, agriculture, and environmental remediation. It allows researchers to optimize nanoparticle production, tailor nanoparticle properties, and explore sustainable and eco-friendly approaches to nanoparticle synthesis.
5. Photosynthetic system
Plants not only have the ability to produce NPs through plant cultures or synthesizing NPs, but the components within the plant cell also have the ability to become functional NPs, in the photosynthetic system [Citation11]. The photosynthetic system, where light energy is converted to chemical energy for the plant’s essential functions, is of great interest and will be further explored in this review through the roles and potential of thylakoids and chlorophyll.
5.1. Thylakoid and light reaction
Thylakoids are specialized membrane-bound compartments found within chloroplasts, playing a crucial role in the light reactions of photosynthesis. These structures contain pigments, particularly chlorophyll, that efficiently absorb light energy. The absorption of light triggers the excitation of electrons in chlorophyll molecules, initiating a sequence of electron transfer events within an embedded electron transport chain [Citation98,Citation99]. This electron transport process generates a proton gradient across the thylakoid membrane, enabling the synthesis of ATP through ATP synthase complexes (). Moreover, the light reactions involve the photolysis of water, resulting in the release of molecular oxygen as a byproduct. Furthermore, the excited electrons are utilized to reduce nicotinamide adenine dinucleotide phosphate (NADP+) to NADPH, a high-energy electron carrier [Citation100]. NADPH, along with ATP, provides the chemical power necessary for the subsequent reactions of the Calvin cycle, facilitating carbon dioxide assimilation and carbohydrate synthesis. Thus, thylakoids play a pivotal role in ATP synthesis, NADPH production, and oxygen evolution. Collectively, these processes enable the conversion of light energy into chemical energy and provide the essential components for carbon fixation during photosynthesis. Consequently, thylakoids can serve as versatile structures capable of supplying general therapeutic molecules, namely ATP, NADPH, and oxygen, to diseased cells.
Figure 5. Photosynthetic electron transport on the thylakoid membrane which synthesizes ATP through the ATP synthase complexes.
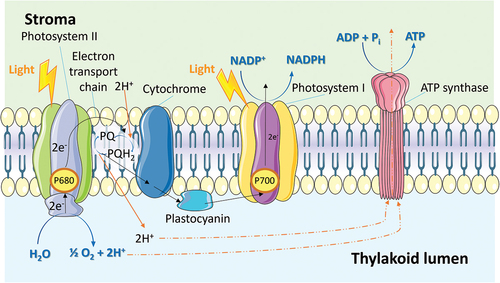
However, under conditions of excessive light intensity, the efficiency of the electron transfer chain in converting all excited electrons into stable chemical energy, such as ATP, NADPH, or O2, becomes limited [Citation101]. As a result, reactive oxygen species (ROS) are generated. Although thylakoids possess an antioxidant system on their membranes, primarily catalase, the overproduction of ROS can overwhelm the antioxidant system and impair the functioning of the light reactions themselves. This further reduces electron conversion efficiency and leads to the accumulation of ROS. Consequently, thylakoids can also serve as light-driven ROS generation structures with destructive implications.
5.1.1. Nano-thylakoid for cancer treatments
Fragmented thylakoids in nano-size, referred to as nano-thylakoids (NT), retain several original functions such as oxygen evolution, enzymatic reactivity, and ROS overproduction, making them suitable for cancer elimination and normalization of the tumor microenvironment. The first reported NT was in 2018 by Jiang et al. They isolated thylakoids from spinach and fragmented them into NT using ultrasonication and membrane filtration techniques () [Citation102]. The produced NT particles had an average size of approximately 50 nm with a polydispersity index (PDI) of around 0.11. Remarkably, the catalase enzyme originally located on the thylakoid membrane was preserved within the NT and exhibited enzymatic activity in converting H2O2 to O2. Moreover, when coupled with chlorophyll electron excitation, the oxygen produced by the catalase enzyme reaction transfers into singlet1O2, a powerful ROS. Consequently, NT was applied as a photodynamic therapy (PDT) agent to convert H2O2 within the hypoxic tumor microenvironment into toxic ROS under external light irradiation. Near-infrared radiation (NIR) was chosen as the light source in this case, as tumors are commonly located deep within tissues, beyond the penetration capacity of visible light. The efficacy of NT was evaluated in vitro using a stage IV human breast cancer cell line, 4T1, as well as in vivo by BALB/c mice bearing 4T1-derived tumors. The NT combined with light irradiation successfully increased the hypoxia levels both in vitro and in vivo. Additionally, the tumor sections from mice demonstrated up-regulation of the hypoxia-related protein HIF-alpha. Amazingly, the tumor size was significantly reduced to its original size within 15 days of treatment, which was markedly lower compared to mice receiving liposomes containing only chlorophyll with NIR irradiation. While this research reveals the potential therapeutic effect of NT, the authors did not include the photosynthetic function in their therapeutic strategy, leading to limited differences between NT and a simple liposome carrying chlorophyll and catalase.
Figure 6. (a) Illustration of the production of nano-thylakoids (NT) and the mode of action of PDT-based cancer treatment. (b) Chemical structure of chlorophyll. Reproduced from Ref [Citation102]. With permission from the Royal Society of Chemistry.
![Figure 6. (a) Illustration of the production of nano-thylakoids (NT) and the mode of action of PDT-based cancer treatment. (b) Chemical structure of chlorophyll. Reproduced from Ref [Citation102]. With permission from the Royal Society of Chemistry.](/cms/asset/05bc4ba0-9aa8-4587-aaa2-2edaddd45dfe/tsta_a_2315013_f0006_oc.jpg)
A similar design was reported by Liu et al. in 2021. They incorporated the thylakoid membrane with extracellular vehicles (EV) derived from pro-inflammatory M1 macrophages, creating TK@M1. TK@M1 was utilized to re-polarize pro-tumoral M2 macrophages into an anti-tumoral M1 phenotype [Citation103]. This activation of the tumor microenvironment led to continuous H2O2 production, which was then decomposed into O2 by the catalase on the thylakoid membrane. This hybrid bio-nanovesicle facilitated immune regulation for PDT treatment. The incorporation of M1 EV significantly increased the specific tumor accumulation of TK@M1 and enhanced the O2 production in tumor tissues. This was possible due to the M2 macrophage re-education and the native tumor-targeting capabilities. Consequently, the in vivo PDT performance of TK@M1 was significantly improved compared to bare thylakoid nanovesicles, providing a practical application of the thylakoid membrane in tumor therapy.
Similarly, Lin et al. also utilized NT primarily for its catalase activity. They constructed a mannose-decorated hollow mesoporous Prussian blue (HMPB) nanoplatform (Man-HMPB) with the encapsulation of an autophagy inhibitor hydroxychloroquine (HCQ) to target and polarize tumor-associated macrophages (TAMs) [Citation104]. In vitro studies shown that Man-HMPB/HCQ could significantly enhance cellular internalization of TAMs and induced the polarization of M2-TAMs into M1 phenotypes. To reduce uptake by the reticuloendothelial system, alleviate hypoxia, and induce membrane rupture within the tumor, the researchers prepared a hybrid membrane by combining macrophage membrane and thylakoid membrane, resulting in TK-M@Man-HMPB/HCQ. The macrophage membrane facilitated exceptional tumor targeting distribution, localized membrane rupture, controlled nanoparticle release, and hypoxia alleviation within the tumor microenvironment. In vivo studies demonstrated that TK-M@Man-HMPB/HCQ could suppress tumor growth through TAM polarization, hypoxia alleviation, infiltration of cytotoxic T lymphocytes, and a decrement of regulatory T cells. Although the strategies for tumor targeting and killing were improved and became more intricate in these three consecutive yet independent studies, the role of NT remained primarily as a provider of catalase and chlorophyll, while the delicate photosynthetic functions were overlooked.
On the other hand, Zheng et al. recognized the oxygen evolution capacity of thylakoids and created a photosynthetic leaf-inspired abiotic/biotic NT (PLANT) system. This was done by combining synthetic NPs with the O2-generating thylakoid membrane to achieve efficient in vivo O2 production () [Citation105]. Comparative analysis revealed that PLANT significantly exhibited higher O2 production upon irradiation by 660 nm laser as compared to traditional O2-generating materials such as MnO2 or CaO2. In a multicellular tumor spheroid model, PLANT alleviated apparent tumor hypoxia while concurrently restraining cell migration and inhibited anaerobic respiration. Metabolic analysis further showed that PLANT could restore oxygen supply and could in vivo readjust the abnormal tumor microenvironment. Additionally, this optically controlled tumor oxygenation material was combined with O2-related therapies, including phototherapy and anti-angiogenesis therapy, for enhanced tumor treatment. Although the mechanism behind the improved oxygen evolution with nanoparticle assistance was not fully explained, this pioneering research represents the first attempt to utilize the photosynthetic functions of NT, namely oxygen evolution, offering a novel strategy for cancer treatments.
Figure 7. Schematic diagram of the normalization of tumor induced by the PLANT system. When irradiated by light, photosynthetic enzymes in PLANT catalyzed the water-splitting reaction and produced O2 in situ. Reproduced from Ref [Citation105]. With permission from the American Chemical Society.
![Figure 7. Schematic diagram of the normalization of tumor induced by the PLANT system. When irradiated by light, photosynthetic enzymes in PLANT catalyzed the water-splitting reaction and produced O2 in situ. Reproduced from Ref [Citation105]. With permission from the American Chemical Society.](/cms/asset/f090338f-7192-4837-afcf-c94087e6f447/tsta_a_2315013_f0007_oc.jpg)
5.1.2. Nano-thylakoid for other diseases
In 2018, Zhang et al. [Citation106] reported a novel application of photosynthetic NT aimed to target energy-deficient diseases by supplying ATP, the “molecular unit of currency. To achieve this, they focused on the key photosynthetic reaction in the thylakoid membrane, which involves a linear electron transfer to produce ATP upon light irradiation. They constructed a highly efficient intracellular opto-driven system called HELIOS in short, which could generate cytosolic electrons to significantly enhance intracellular ATP levels upon light irradiation. A proteo-liposome-like structure was synthesized by combining thylakoid fragments from spinach with phosphocholines and cholesterol. The thylakoid enzymes in HELIOS were speculated to use optical energy and transform generated electrons into electron acceptors (NADPH) within the system. These electrons were then transported through the malate-aspartate shuttle system into mammalian mitochondria to generate ATP (). In various cell lines, the addition of HELIOS was observed to significantly increase the intracellular ATP generation when exposed to light irradiation. This treatment could also enhance cellular functions such as fluorescent protein synthesis, cell movement, and insulin secretion. Besides providing ATP during ischemia, HELIOS was able to perform organ powering in rats. The behavioral experiments performed on zebrafish also demonstrated the potential of this strategy to be translated to other living animals. In plants, the primary purpose of photosynthesis is to absorb light energy and store abundant solar energy into chemical energy as fuel for cell metabolism, namely ATP and NADPH in light reactions which are further converted into more stable chemical energy in the form of glucose or starch. This work was indeed the pioneering work that applied plant-origin NT as a photosynthetic platform to convert light energy into chemical energy that can be utilized by mammalian cells, proving NT as a promising candidate in treating energy-deficient diseases. The author claimed that such energy supply was enabled by the photosynthetic generation of NADPH and this NADPH diffused into mitochondria and participated in the tricarboxylic acid cycle (TCA) cycle in generating ATP, which was, however, problematic. First, only the reduced nicotinamide adenine dinucleotide (NADH) can be directly utilized by the TCA cycle to power ATP synthesis. Mitochondrial conversion of NADPH into NADH was slow and only accounted for a minimal amount of ATP synthesis. Second, NADH cannot be produced in light reaction as the author claimed, hence the NADPH-powered ATP synthesis would not be possible. Instead, ATP itself in light reaction will be generated in photosystem II (PSII). Therefore, the energy-supplying effect in vitro or in vivo in this research might mainly come from ATP produced by NT rather than from mitochondria in the targeted cell.
Figure 8. Schematic of HELIOS. (a) Isolating the plant photosystem. (b) The assembly into a nanoparticle, through the conversion of optical energy to ATP chemical energy to power cells. Reproduced from Ref [Citation106]. With permission from John Wiley and Sons.
![Figure 8. Schematic of HELIOS. (a) Isolating the plant photosystem. (b) The assembly into a nanoparticle, through the conversion of optical energy to ATP chemical energy to power cells. Reproduced from Ref [Citation106]. With permission from John Wiley and Sons.](/cms/asset/fdcfef03-b831-42a0-93ab-f251ac1fa360/tsta_a_2315013_f0008_oc.jpg)
Building upon this research, Chen et al. further investigated the photosynthetic ATP production of NT and applied it to power cellular anabolism and promote cellular metabolic reprogramming [Citation107]. They developed a nanosized plant-derived photosynthetic system based on NT that was independent and controllable. To enable applications on other species, they used chondrocyte membrane, a specific mature cell membrane, for camouflage encapsulation. The study demonstrated that these membrane-coated NT entered chondrocytes through membrane fusion, avoiding lysosome degradation and achieving rapid penetration. Exposure to light increased intracellular ATP levels in situ, leading to improved anabolism in degenerated chondrocytes. The NT corrected energy imbalance systemically, improved cartilage homeostasis, restored cellular metabolism, and protected against the pathological progression of osteoarthritis. This therapeutic strategy successfully utilized a natural photosynthetic system to enhance cell anabolism by providing key metabolic and energy carriers. Thus, demonstrating the potential and feasibility of the transplantation of a plant-derived photosynthetic system across the other species. This approach has potential applications in degenerative diseases related to abnormal anabolism or metabolism.
5.2. Chlorophyll
Diving into the pivotal molecule and pigment found inside the thylakoid, crucial for the photosynthesis process, chlorophyll is abundantly present in plant species, especially those that exhibit green pigmentation. With approximately a quarter of the world’s land area covered by forests, the potential reservoirs of chlorophyll are vast. This essential pigment can be extracted from a diverse array of plant sources. Chlorophyll a and chlorophyll b usually exist in plant species, while chlorophyll c, chlorophyll d, and chlorophyll f usually exist in various algae and microalgae () [Citation108,Citation109]. The abundant reserves of chlorophyll in nature and its inherent fluorescence have spurred numerous investigations into its various possible applications.
Figure 9. Molecular structures of various chlorophyll molecules. (a) Molecular structure of chlorophyll a.(b) Molecular structure of chlorophyll b. (c) Molecular structure of chlorophyll c. (d) Molecular structure of chlorophyll d. (e) Molecular structure of chlorophyll f.
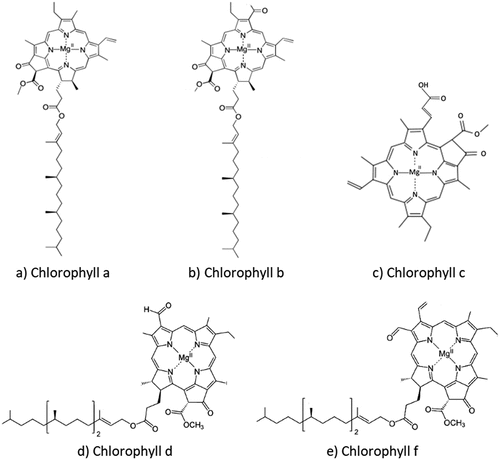
The fluorescence intensity of chlorophyll serves as an indicator of its content, enabling a rapid assessment of photosynthetic performance. This innovative approach has allowed researchers to enhance crop development by efficiently gauging photosynthetic efficiency through chlorophyll fluorescence measurements [Citation110]. Moreover, chlorophyll’s versatile properties extend beyond its role in plants. Through strategic blending with other materials, chlorophyll has contributed to the development of artificial photosynthesis systems. This innovative application capitalizes on chlorophyll’s light-capturing capabilities, mirroring the natural photosynthetic process, and holds potential for advancements in various fields [Citation13,Citation111]. In addition, Pheophorbide-Appending Cellulose was used to make nanorods to work as light absorbers [Citation112]. The constant exploration of chlorophyll’s attributes underscores its significance as a fundamental natural resource that continues to inspire new scientific inquiries and applications. In addition to the application in crops, this review will focus more on the application of chlorophyll and its derivatives in biomedicine.
Chlorophyll is characterized by a cyclic tetrapyrrole structure, prominently featuring a homocyclic five-membered ring [Citation113]. This unique structural aspect, referred to as a macrocycle, serves as a focal point of chlorophyll research. Investigations predominantly center around the macrocycle, leading to the creation of numerous chlorophyll derivatives through modifications. The chemical attributes of these derivatives are significantly altered by factors such as the extent of macrocycle reduction, metal ion coordination, and the presence of substituents. These modified derivatives offer a range of valuable functionalities and traits. Notably, they exhibit potent antioxidant capabilities, effectively serving as scavengers for free radicals. Additionally, these derivatives possess light absorption properties, rendering them valuable photosensitizers for inducing light-triggered cell damage, a trait pivotal in applications like PDT [Citation114]. The diverse outcomes achieved by manipulating the macrocycle of chlorophyll highlight the potential for multifaceted applications, spanning from antioxidant interventions to advanced therapeutic strategies involving light-mediated cellular responses, which will be discussed further [Citation115].
5.2.1. Antioxidant capability
Substantial research has demonstrated that both chlorophyll and its derivatives play a pivotal role in mitigating oxidative stress by activating cellular antioxidant pathways. These compounds exhibit considerable efficacy in neutralizing diverse free radical species, including hydrogen peroxide (H2O2), hydroxyl radical (·OH), and singlet oxygen (1O2) [Citation116]. Hence, chlorophyll and its derivatives are effective as potent antioxidants capable of diminishing ROS levels. To further explore the naturally occurring chlorophyll as an antioxidant, researchers studied the reactions and enzymes responsible for the production and dissemination of ROS as shown in [Citation117]. The antioxidant reactions were catalysed by antioxidant enzymes such as superoxide dismutase (SOD), catalase (CAT), glutathione peroxidase (GPx), glutathione reductase (GR), the redox state of glutathione of reduced glutathione (GSH) and oxidized glutathione (GSSG). However, when compared with other antioxidants like carotenoids and tocopherols, chlorophyll and its derivatives exhibit a relatively lower antioxidant potency at equivalent concentrations [Citation108,Citation117]. To attain antioxidant effects on par with those of carotenoids and tocopherols, it is necessary to extract higher concentrations of chlorophyll and its derivatives. The mechanisms of antioxidant effects of carotenoids are illustrated in . Electrons are conveyed from the carotenoid to the photosystem reaction center, resulting in the generation of an excited state. demonstrates its proficiency in quenching singlet oxygen [Citation117].
Figure 10. The mechanisms delineating the antioxidant action of chlorophyll and carotenoid in diverse processes. (a) The reactive oxygen species (ROS) generation and propagation of chlorophyll antioxidant and the participating reactions and enzymes. (b) Depicts the electron transfer from the characteristic conjugated polyenic chain of carotenoids (Car) to chlorophylls (Chl). (c) This entails the physical quenching of singlet oxygen [Citation117].
![Figure 10. The mechanisms delineating the antioxidant action of chlorophyll and carotenoid in diverse processes. (a) The reactive oxygen species (ROS) generation and propagation of chlorophyll antioxidant and the participating reactions and enzymes. (b) Depicts the electron transfer from the characteristic conjugated polyenic chain of carotenoids (Car) to chlorophylls (Chl). (c) This entails the physical quenching of singlet oxygen [Citation117].](/cms/asset/c28ec6f3-e6cc-4e7a-bd87-d96ee7221f8a/tsta_a_2315013_f0010_oc.jpg)
5.2.2. Photodynamic therapy (PDT) effect
Tetrapyrrole macrocycles exhibit distinct absorption spectra in the visible and near-infrared regions, characterized by two prominent bands: the B-band or Soret-band around 400 nm and the Q-band occurring within the range of 600–800 nm. This spectral behavior denotes that longer wavelengths hold greater penetration capabilities, while shorter wavelengths offer higher energy levels. The inherent cyclic tetrapyrrole structure with a five-membered ring within chlorophyll contributes to its remarkable light-absorption proficiency. Notably, research has demonstrated that derivatives of chlorophyll possess robust light-absorption capabilities. When subjected to light of specific wavelengths, both chlorophyll and its derivatives undergo excitation, transitioning from a ground state to a transient excited state, thus engendering a PDT effect seen in [Citation118–120].
Figure 11. Type 1 and type 2 reactions in photodynamic therapy (PDT) [Citation119]. Upon excitation, the photosensitizer undergoes a transition from its ground state to a transient excited state, thereby initiating a PDT effect. This process involves two pathways: Type I reactions generate radicals and radical anion species (such as O2• – and HO•), whereas Type II reactions produce singlet oxygen (1O2) from molecular oxygen (O2).
![Figure 11. Type 1 and type 2 reactions in photodynamic therapy (PDT) [Citation119]. Upon excitation, the photosensitizer undergoes a transition from its ground state to a transient excited state, thereby initiating a PDT effect. This process involves two pathways: Type I reactions generate radicals and radical anion species (such as O2• – and HO•), whereas Type II reactions produce singlet oxygen (1O2) from molecular oxygen (O2).](/cms/asset/2b9a9dcc-6c60-4270-ae81-b048a4e32cfc/tsta_a_2315013_f0011_oc.jpg)
Pheophorbide, a derivative of chlorophyll, holds substantial potential for biomedical applications. Research has illuminated that pheophorbide exhibits an intriguing capability: tumor cells display a higher uptake of pheophorbide compared to normal cells. This differential absorption provides a remarkable opportunity to harness pheophorbide’s therapeutic potential in a targeted manner. A noteworthy study conducted on myeloma cells exhibited a distinct preference for pheophorbide uptake over normal spleen cells. This observation demonstrated that pheophorbide indeed has enhanced affinity for tumor cells, effectively enabling selective absorption by these cells as opposed to normal cells [Citation121]. Subsequently, this selective uptake of pheophorbide by tumor cells is harnessed to leverage the PDT effect of pheophorbide, thereby instigating tumor cell destruction and, consequently, achieving therapeutic objectives in tumor treatment [Citation122].
In addition to the field of tumor treatment, chlorophyll and its derivatives play a significant role in the food industry. When chlorophyll was incorporated into a food packaging film development, it was able to generate active oxygen species after being irradiated by light to sterilize and protect food [Citation123,Citation124].
Researchers have harnessed the unique structure and properties of chlorophyll and its derivatives to induce antibacterial effects through PDT. This strategy capitalizes on the ability of chlorophyll and its derivatives to absorb light and generate ROS upon activation. When exposed to specific wavelengths of light, these compounds transition from their ground state to an excited state, triggering the generation of ROS that can induce oxidative stress and cellular damage in bacteria. This targeted mechanism of action makes PDT a promising avenue for combating bacterial infections while minimizing the risk of antibiotic resistance. By ingeniously utilizing the inherent properties of chlorophyll and its derivatives, researchers are exploring novel ways to address the challenge of bacterial infections in both medical and environmental contexts [Citation125].
5.2.3. Antimutagenic capability
Chlorophyll possesses a range of potential benefits that extend beyond its well-established photodynamic and antioxidant properties. Studies have shed light on its remarkable capacity to counteract mutagenesis, particularly its role in preventing liver cancer, especially in individuals exposed to aflatoxins, which is a potent mutagen that is often present in certain foods. The antimutagenic capability of chlorophyll is attributed to its unique ability to selectively bind with mutagens in the digestive tract. This binding action not only reduces the availability of mutagens but also hinders the body’s absorption of aflatoxins. Consequently, this process results in a reduction in DNA damage, effectively mitigating the risk of mutagen-induced harm. Additionally, the impact of chlorophyll extends to the modulation of enzyme activity, further contributing to its protective effects against mutagenesis [Citation126]. This multifaceted approach strengthens the potential of chlorophyll in combating mutagenic processes, ultimately playing a pivotal role in the prevention of liver cancer and promoting overall health.
6. Current gaps and future perspectives of nature-derived nanomaterials
The synthesis efficiency and scalability of nanomaterials derived from animal and plant cells can vary depending on several factors. In this section, we compare the factors and strategies for optimizing production yield. The synthesis of nanomaterials derived from animal cells can involve complex cellular processes, which may require specialized culture conditions, extended incubation times, and specific enzymatic reactions. Factors such as cell viability, enzyme activity, and the specific biochemical pathways involved can influence the efficiency of synthesis. In contrast, the synthesis of nanomaterials from plant cells often involves simpler processes, such as the extraction and purification of natural plant components or enzymatic reactions. Plant cells are amenable to large-scale cultivation, producing abundant biomass, which contributes to higher synthesis efficiency.
Scaling up the production of nanomaterials from animal cells can be challenging due to factors like limited cell availability, complex culture systems, and the need for stringent quality control. Optimization of cell culture parameters, media composition, and bioreactor design may be required. Furthermore, nanomaterials derived from plant cells offer better scalability due to the abundance and rapid growth of plant biomass. Large-scale cultivation using conventional agricultural practices makes it easier to meet the demand for nanomaterial production. Plant-based systems can also be engineered for enhanced production of target nanomaterials.
The production yields of both animal and plant cell-derived nanomaterials can be influenced by various factors, including the choice of specific cells or plant tissues, cell viability, growth conditions, and genetic variability. Moreover, the efficiency of extraction methods employed to isolate nanomaterials from cells or tissues can influence production yields. Optimization of extraction techniques, such as selection of solvents, pH conditions, and mechanical disruption, can enhance yields. Understanding and manipulating the cellular metabolism and pathways involved in nanomaterial synthesis can impact production yields. Modulating enzyme activity, precursor availability, and cofactors can optimize yields.
As many of these nature-derived nanomaterials have biomedical endpoints, maintaining consistency in the quality across batches can be a significant challenge, even after addressing the scaling-up issues. Compartmentalization into multiple streams in parallel batch processing through microfluidics techniques might be able to solve these consistency issues. When multiple streams are deployed, individualized level sampling of the parallel streams can be carried out to ‘reject’ streams that do not meet the standards from entering the final merging of the product stream, ensuring the fidelity of the overall final product.
Regarding nano-photosynthesis systems derived from either from plant or cyanobacterial, their stability under room temperature as well as storage conditions remains a significant area for optimization. Meanwhile, the isolation procedure could be optimized to maximally preserve the delicate light-harvesting system in the intact thylakoid membrane. Additionally, preventing the formation of ROS, a by-product of light-chemical energy conversion, is crucial to avoid potential damage to the bioactivity of NPs and the target cell itself. If not addressed, this could pose a threat even without causing cell death. Therefore, addressing the prevention of ROS formation is an essential task.
While animal cell-derived nanomaterials may have more complex synthesis processes and scalability challenges, plant cell-derived nanomaterials offer advantages in terms of scalability, ease of cultivation, and availability of biomass. Strategies for optimization should focus on genetic engineering, bioprocess optimization, enzyme engineering, downstream processing, and scale-up approaches to enhance production yields for both types of nanomaterials.
Last but not least, while all of these nanomaterials are derived from nature and in fact some of these materials are food in normal circumstance, they may or may not be safe. Safety needs, therefore, need to be ascertained since these are meant for biomedical applications. As the possibilities of cell derived nanomaterials are tremendous, advanced testing methodologies may need to be developed to catch up with the possible tsunami of these novel candidates. Advanced methodologies of using induced pluripotent stem cells (iPSCs) to better represent the target organ of interest and artificial intelligence driven data pattern recognition may be deployed to cope with the possibly large influx of data.
7. Conclusion
Nature has much to offer to the materials scientists. Materials syntheses that have taken eons to perfect can be employed to create new materials at an accelerated pace. Nature has a ready-to-use toolbox and treasure trove of novel and unique structures for our imaginative use and applications. Through this, we may be able to better expand the possibilities far beyond our current horizons.
Acknowledgments
The authors would like to acknowledge the generous funding from the Singapore Ministry of Education and NUS Reimagine Grant (A-0009179-02-00; A-0009179-03-00), Singapore Food Story Grant (A-8000575-00-00; Grant no: W22W3D0007), The Japan Society for the Promotion of Science (JSPS 2023 Fellowship; D.L.), and The China Scholarship Council [financial support for the postdoctoral fellow study provided by J.W.].
Disclosure statement
No potential conflict of interest was reported by the author(s).
Additional information
Funding
Notes on contributors
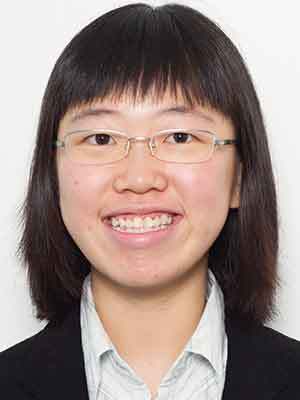
Li Xian Yip
Li Xian Yip is currently a Ph.D. student in the Department of Chemical and Biomolecular Engineering, National University of Singapore (NUS). She received her Master of Science and Bachelor of Engineering degrees in Chemical Engineering from NUS. Her research interests include nanotoxicology, nanomaterials-cell interactions, nanotherapeutics and computational fluid dynamics.
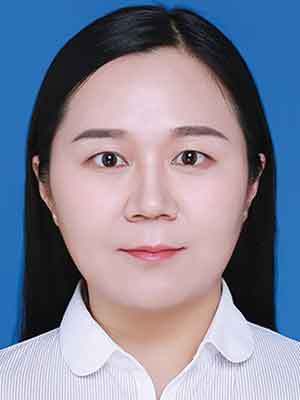
Jinping Wang
Jinping Wang is an Assistant Professor at the School of Biological Science and Technology, University of Jinan. She obtained her Ph.D. in Chemical and Biomolecular Engineering from National University of Singapore (NUS). Her research interests include nanomaterials-cell interactions, drug delivery, and electrochemical sensing.
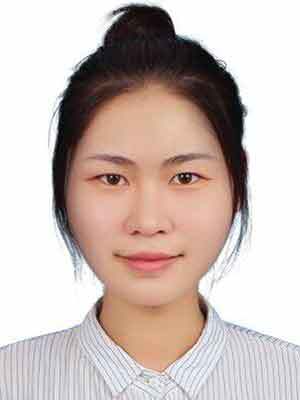
Yuling Xue
Yuling Xue is a research engineer in the Department of Chemical and Biomolecular Engineering, National University of Singapore (NUS). She received her Master of Engineering degree in Chemical and Biomolecular Engineering from NUS. Her research interests include chemical synthesis, antibacterial and antiviral compounds, and bacteriophage.
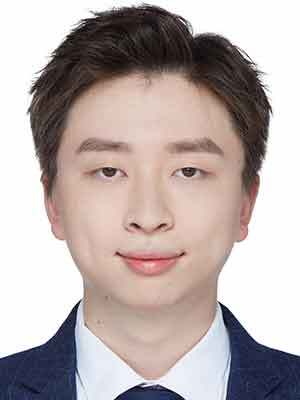
Kuoran Xing
Kuoran Xing is currently a Ph.D. student in NUS Graduate School for Integrative Sciences & Engineering Programme hosted by the Department of Chemical and Biomolecular Engineering, National University of Singapore (NUS). He received B.Eng degree in Polymer Materials & Engineering from Zhejiang University. His research interests include organellar nanotechnology, plant-derived nanomedicine and drug delivery system.
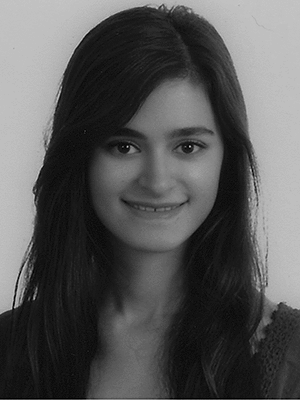
Cansu Sevencan
Cansu Sevencan is a research fellow at the Department of Chemical and Biomolecular Engineering, National University of Singapore (NUS). She received her Ph.D. degree from NUS under the guidance of Associate Professor David Tai Leong in 2020. Her research interests include cellular nanomaterials, cell membrane nanotechnologies, and biological effects and applications of nanomaterials.
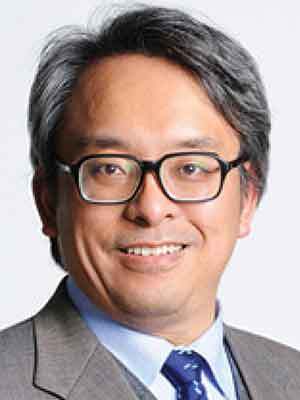
Katsuhiko Ariga
Katsuhiko Ariga received his Ph.D. degree from Tokyo Institute of Technology in 1990. He joined the National Institute for Materials Science (NIMS) in 2004 and is currently the leader of the Supermolecules Group and principal investigator of the World Premier International (WPI) Research Centre for Materials Nanoarchitectonics (MANA), NIMS. He is also appointed as a professor of The University of Tokyo and an advisory board member of Angew. Chem. Int. Ed., Adv. Mater., Chem. Asian J., and ChemNanoMat.
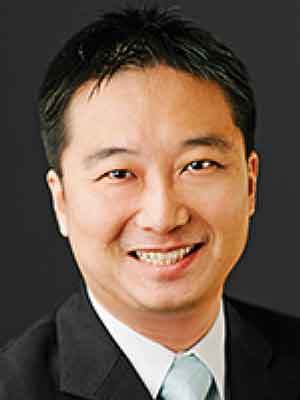
David Tai Leong
David Tai Leong is an Associate Professor at the Department of Chemical and Biomolecular Engineering, National University of Singapore (NUS). He obtained his Ph.D. in Biology and Bachelor in Chemical Engineering from NUS, and received his post-doctoral training at the Howard Hughes Medical Institute at the University of Pennsylvania. He is also an elected Fellow of the Royal Society of Chemistry. His research interests span across the fundamental understanding of biological effects of nanomaterials to their applications in nanomedicine, biosensing, and nanotoxicology.
References
- Mohammadinejad R, Karimi S, Iravani S, et al. Plant-derived nanostructures: types and applications. Green Chem. 2016;18(1):20–25. doi: 10.1039/C5GC01403D
- Lovley DR, Stolz JF, Nord GL Jr, et al. Anaerobic production of magnetite by a dissimilatory iron-reducing microorganism. Nature. 1987;330(6145):252–254. doi: 10.1038/330252a0
- Govindasamy R, Gayathiri E, Sankar S, et al. Emerging trends of nanotechnology and genetic engineering in cyanobacteria to optimize production for future applications. Life. 2022;12(12):2013. doi: 10.3390/life12122013
- Li BL, Zhang H, Li NB, et al. Materialistic interfaces with nucleic acids: principles and their impact. Adv Funct Mater. 2022;32(37):1–29. doi: 10.1002/adfm.202201172
- Li Y, Cui R, Zhang P, et al. Mechanism-oriented controllability of intracellular quantum dots formation: the role of glutathione metabolic pathway. ACS Nano. 2013;7(3):2240–2248. doi: 10.1021/nn305346a
- Lo Presti M, Vona D, Ragni R, et al. Perspectives on applications of nanomaterials from shelled plankton. MRS Commun. 2021;11(3):213–225. doi: 10.1557/s43579-021-00032-0
- Nawaz MA, Zakharenko AM, Zemchenko IV, et al. Phytolith formation in plants: from soil to cell. Plants (Basel). 2019;8(8):249. doi: 10.3390/plants8080249
- Akhtar MS, Panwar J, Yun Y-S. Biogenic synthesis of metallic nanoparticles by plant extracts. ACS Sustainable Chem Eng. 2013;1(6):591–602. doi: 10.1021/sc300118u
- Zhang Q, Dehaini D, Zhang Y, et al. Neutrophil membrane-coated nanoparticles inhibit synovial inflammation and alleviate joint damage in inflammatory arthritis. Nat Nanotech. 2018;13(12):1182–1190. doi: 10.1038/s41565-018-0254-4
- Kobylinska N, Shakhovsky A, Khainakova OK, et al. ‘Hairy’ root extracts as source for ‘green’ synthesis of silver nanoparticles and medical applications. RSC Adv. 2020;10:39434–39446. doi: 10.1039/D0RA07784D
- Kong Q, Zhu Z, Xu Q, et al. Nature-inspired thylakoid-based photosynthetic nanoarchitectures for biomedical applications. Small Methods. 2023:2301143–2301162. doi: 10.1002/smtd.202301143
- Gao W, Fang RH, Thamphiwatana S, et al. Modulating antibacterial immunity via bacterial membrane-coated nanoparticles. Nano Lett. 2015;15(2):1403–1409. doi: 10.1021/nl504798g
- Ruiz-Hitzky E, Ariga K, Lvov YM. Bio-inorganic hybrid nanomaterials: strategies, synthesis, characterization and applications. Germany: John Wiley & Sons; 2008. https://books.google.ie/books?id=yN9XlxJazTUC&printsec=frontcover&dq=Bio-inorganic+Hybrid+Nanomaterials:+Strategies,+Synthesis,+Characterization+and+Applications&hl=&cd=1&source=gbs_api#v=onepage&q=Bio-inorganic%20Hybrid%20Nanomaterials%3A%20Strategies%2C%20Synthesis%2C%20Characterization%20and%20Applications&f=false
- Elfick A, Rischitor G, Mouras R, et al. Biosynthesis of magnetic nanoparticles by human mesenchymal stem cells following transfection with the magnetotactic bacterial gene mms6. Sci Rep. 2017;7(1):39755. doi: 10.1038/srep39755
- Tian L-J, Li W-W, Zhu T-T, et al. Directed biofabrication of nanoparticles through regulating extracellular electron transfer. J Am Chem Soc. 2017;139(35):12149–12152. doi: 10.1021/jacs.7b07460
- Cohen S, Valm AM, Lippincott-Schwartz J. Interacting organelles. Curr Opinion Cell Biol. 2018;53:84–91. doi: 10.1016/j.ceb.2018.06.003
- Boeynaems S, Alberti S, Fawzi NL, et al. Protein phase separation: a new phase in cell biology. Trends Cell Biol. 2018;28(6):420–435. doi: 10.1016/j.tcb.2018.02.004
- Woodruff JB, Hyman AA, Boke E. Organization and function of non-dynamic biomolecular condensates. Trends Biochem Sci. 2018;43(2):81–94. doi: 10.1016/j.tibs.2017.11.005
- Wang C, Yang J, Lu Y. Modularize and unite: toward creating a functional artificial cell. Front Mol Biosci. 2021;8. doi: 10.3389/fmolb.2021.781986
- O’Connor C, Adams JU. Essentials of cell biology. Cambridge, MA: NPG Education; 2010.
- Khoshmanesh K, Kouzani AZ, Nahavandi S, et al. At a glance: cellular biology for engineers. Comput Biol Chem. 2008;32(5):315–331. doi: 10.1016/j.compbiolchem.2008.07.010
- Lin J, Yang K, New EJ. Strategies for organelle targeting of fluorescent probes. Org Biomol Chem. 2021;19(43):9339–9357. doi: 10.1039/D1OB01447A
- Rolfe DFS, Brown GC. Cellular energy utilization and molecular origin of standard metabolic rate in mammals. Physiol Rev. 1997;77(3):731–758. doi: 10.1152/physrev.1997.77.3.731
- Starnes DL, Jain A, Sahi SV. In planta engineering of gold nanoparticles of desirable geometries by modulating growth conditions: an environment-friendly approach. Environ Sci Technol. 2010;44(18):7110–7115. doi: 10.1021/es101136q
- Elahian F, Reiisi S, Shahidi A, et al. High-throughput bioaccumulation, biotransformation, and production of silver and selenium nanoparticles using genetically engineered Pichia pastoris. Nanomedicine. 2017;13(3):853–861. doi: 10.1016/j.nano.2016.10.009
- Zhang D, Wu T, Qin X, et al. Intracellularly generated immunological gold nanoparticles for combinatorial photothermal therapy and immunotherapy against tumor. Nano Lett. 2019;19(9):6635–6646. doi: 10.1021/acs.nanolett.9b02903
- Gan PP, Li SFY. Potential of plant as a biological factory to synthesize gold and silver nanoparticles and their applications. Rev Environ Sci Bio/Technol. 2012;11(2):169–206. doi: 10.1007/s11157-012-9278-7
- Barwal I, Ranjan P, Kateriya S, et al. Cellular oxido-reductive proteins of chlamydomonas reinhardtii control the biosynthesis of silver nanoparticles. J Nanobiotechnol. 2011;9(1):56. doi: 10.1186/1477-3155-9-56
- Mann S, Frankel RB, Blakemore RP. Structure, morphology and crystal growth of bacterial magnetite. Nature. 1984;310(5976):405–407. doi: 10.1038/310405a0
- Smith PR, Holmes JD, Richardson DJ, et al. Photophysical and photochemical characterisation of bacterial semiconductor cadmium sulfide particles. J Chem Soc Faraday Trans. 1998;94(9):1235–1241. doi: 10.1039/a708742j
- Kröger N, Deutzmann R, Sumper M. Polycationic peptides from diatom biosilica that direct silica nanosphere formation. Science. 1999;286(5442):1129–1132. doi: 10.1126/science.286.5442.1129
- Rosenfeldt S, Riese CN, Mickoleit F, et al. Probing the nanostructure and arrangement of bacterial magnetosomes by small-angle X-Ray scattering. Appl environ microbiol. 2019;85(24):e01513–19. doi: 10.1128/AEM.01513-19
- Abdelmoneim HM, Taha TH, Elnouby MS, et al. Extracellular biosynthesis, OVAT/statistical optimization, and characterization of silver nanoparticles (AgNPs) using Leclercia adecarboxylata THHM and its antimicrobial activity. Microb Cell Factories. 2022;21(1):277. doi: 10.1186/s12934-022-01998-9
- Saifuddin N, Wong CW, Yasumira AAN. Rapid biosynthesis of silver nanoparticles using culture supernatant of bacteria with microwave irradiation. E-J Chem. 2009;6(1):61–70. doi: 10.1155/2009/734264
- Anandan M, Poorani G, Boomi P, et al. Green synthesis of anisotropic silver nanoparticles from the aqueous leaf extract of Dodonaea viscosa with their antibacterial and anticancer activities. Process Biochem. 2019;80:80–88. doi: 10.1016/j.procbio.2019.02.014
- Prakash P, Gnanaprakasam P, Emmanuel R, et al. Green synthesis of silver nanoparticles from leaf extract of Mimusops elengi, Linn. For enhanced antibacterial activity against multi drug resistant clinical isolates. Colloids Surf B Biointerfaces. 2013;108:255–259. doi: 10.1016/j.colsurfb.2013.03.017
- Iravani S. Green synthesis of metal nanoparticles using plants. Green Chem. 2011;13(10):2638–2650. doi: 10.1039/C1GC15386B
- Devi TB, Ahmaruzzaman M. Bio-inspired facile and green fabrication of Au@Ag@AgCl core–double shells nanoparticles and their potential applications for elimination of toxic emerging pollutants: a green and efficient approach for wastewater treatment. Chem Eng J. 2017;317:726–741. doi: 10.1016/j.cej.2017.02.082
- Ishigami T, Suga K, Umakoshi H. Chiral recognition of L-Amino acids on liposomes prepared with L-Phospholipid. ACS Appl Mater Interfaces. 2015;7(38):21065–21072. doi: 10.1021/acsami.5b07198
- Li BL, Luo JJ, Zou HL, et al. Chiral nanocrystals grown from MoS(2) nanosheets enable photothermally modulated enantioselective release of antimicrobial drugs. Nat Commun. 2022;13(1):7289. doi: 10.1038/s41467-022-35016-8
- Simons K, Gerl MJ. Revitalizing membrane rafts: new tools and insights. Nat Rev Mol Cell Biol. 2010;11:688–699. doi: 10.1038/nrm2977
- Handa M, Beg S, Shukla R, et al. Recent advances in lipid-engineered multifunctional nanophytomedicines for cancer targeting. JCR. 2021;340:48–59. doi: 10.1016/j.jconrel.2021.10.025
- Kolašinac R, Jaksch S, Dreissen G, et al. Influence of environmental conditions on the fusion of cationic liposomes with living mammalian cells. 2019;9(7):1025. doi: 10.3390/nano9071025
- Krammer F, Schinko T, Palmberger D, et al. Trichoplusia ni cells (High FiveTM) are highly efficient for the production of influenza a virus-like particles: a comparison of two insect cell lines as production platforms for influenza vaccines. Mol Biotechnol. 2010;45(3):226–234. doi:10.1007/s12033-010-9268-3
- Adigun OO, Novikova G, Retzlaff-Roberts EL, et al. Decoupling and elucidation of surface-driven processes during inorganic mineralization on virus templates. J Colloid Interface Sci. 2016;483:165–176. doi: 10.1016/j.jcis.2016.07.028
- Hildebrand M. Biological processing of nanostructured silica in diatoms. Prog Org Coat. 2003;47(3):256–266. doi: 10.1016/S0300-9440(03)00142-5
- Dujardin E, Mann S. Bio‐inspired materials chemistry. Adv Mater. 2002;14(11):775–788. doi: 10.1002/1521-4095(20020605)14:11<775::AID-ADMA775>3.0.CO;2-0
- Xiong L-H, Tu J-W, Zhang Y-N, et al. Designer cell-self-implemented labeling of microvesicles in situ with the intracellular-synthesized quantum dots. Sci China Chem. 2020;63(4):448–453. doi: 10.1007/s11426-019-9697-2
- Das RK, Pachapur VL, Lonappan L, et al. Biological synthesis of metallic nanoparticles: plants, animals and microbial aspects. Nanotech Env Eng. 2017;2(18). doi: 10.1007/s41204-017-0029-4
- Zhu H, Zan W, Chen W, et al. Defect‐rich molybdenum sulfide quantum dots for amplified photoluminescence and photonics‐driven reactive oxygen species generation. Nanoscale Horiz. 2022;34(31). doi: 10.1002/adma.202200004
- Zhu H, Huang S, Ding M, et al. Sulfur defect-engineered biodegradable cobalt sulfide quantum dot-driven photothermal and chemodynamic anticancer therapy. ACS Appl Mater Interfaces. 2022;14(22):25183–25196. doi: 10.1021/acsami.2c05170
- Cao H, Dan Z, He X, et al. Liposomes coated with isolated macrophage membrane can target lung metastasis of breast cancer. ACS Nano. 2016;10(8):7738–7748. doi: 10.1021/acsnano.6b03148
- Bergin IL, Witzmann FA. Nanoparticle toxicity by the gastrointestinal route: evidence and knowledge gaps. J Biomed Nanosci Nanotechnol. 2013;3(1–2):163–210. doi: 10.1504/IJBNN.2013.054515
- Aird WC. Phenotypic heterogeneity of the endothelium: I. Structure, function, and mechanisms. Circ Res. 2007;100(2):158–173. doi: 10.1161/01.RES.0000255691.76142.4a
- Baldwin AL, Thurston G. Mechanics of endothelial cell architecture and vascular permeability. Crit Rev Biomed Eng. 2001;29(2):247–278. doi: 10.1615/critrevbiomedeng.v29.i2.20
- Shen X, Ariga K. Disease diagnosis with chemosensing, artificial intelligence, and prospective contributions of nanoarchitectonics. Chemosensors. 2023;11(10):528–547. doi: 10.3390/chemosensors11100528
- Hanahan D, Weinberg RA. Hallmarks of cancer: the next generation. Cell. 2011;144(5):646–674. doi: 10.1016/j.cell.2011.02.013
- Fang J, Nakamura H, Maeda H. The EPR effect: Unique features of tumor blood vessels for drug delivery, factors involved, and limitations and augmentation of the effect. Adv Drug Deliv Rev. 2011;63(3):136–51. doi: 10.1016/j.addr.2010.04.009
- Tee JK, Yip LX, Tan ES, et al. Nanoparticles’ interactions with vasculature in diseases. Chem Soc Rev. 2019;48:5381–5407. doi: 10.1039/C9CS00309F
- Setyawati MI, Tay CY, Docter D, et al. Understanding and exploiting nanoparticles’ intimacy with the blood vessel and blood. Chem Soc Rev. 2015;44(2):8174–8199. doi: 10.1038/s41565-018-0356-z
- Atukorale PU, Covarrubias G, Bauer L, et al. Vascular targeting of nanoparticles for molecular imaging of diseased endothelium. Adv Drug Deliv Rev. 2017;113:141–156. doi: 10.1016/j.addr.2016.09.006
- Peng F, Setyawati MI, Tee JK, et al. Nanoparticles promote in vivo breast cancer cell intravasation and extravasation by inducing endothelial leakiness. Nat Nanotech. 2019;14(3):279–286. doi: 10.1038/s41565-018-0356-z
- Chung BL, Toth MJ, Kamaly N, et al. Nanomedicines for endothelial disorders. Nano Today. 2015;10(6):759–776. doi: 10.1016/j.nantod.2015.11.009
- Setyawati MI, Wang Q, Ni N, et al. Engineering tumoral vascular leakiness with gold nanoparticles. Nat Commun. 2023;14(1):4269. doi: 10.1038/s41467-023-40015-4
- Wang Q, Liang Q, Dou J, et al. Breaking through the basement membrane barrier to improve nanotherapeutic delivery to tumours. Nat Nanotech. 2023. doi:10.1038/s41565-023-01498-w.
- Ni N, Wang W, Sun Y, et al. Inducible endothelial leakiness in nanotherapeutic applications. Biomaterial. 2022:287. doi: 10.1016/j.biomaterials.2022.121640
- Greenwald J, Riek R. Biology of amyloid: structure, function, and regulation. Structure. 2010;18(10):1244–1260. doi: 10.1016/j.str.2010.08.009
- Li Y, Ni N, Lee M, et al. Endothelial leakiness elicited by amyloid protein aggregation. Nature Comms. 2024;15(1): 613–629. doi: 10.1038/s41467-024-44814-1
- Liu Q, Wu D, He B, et al. Attenuating endothelial leakiness with self-assembled DNA nanostructures for pulmonary arterial hypertension. Nanoscale Horiz. 2023;8(2):270–278. doi: 10.1039/d2nh00348a
- Goñi FM. The basic structure and dynamics of cell membranes: an update of the singer–Nicolson model. Biochim Biophys Acta – Biomembr. 2014;1838(6):1467–1476. doi: 10.1016/j.bbamem.2014.01.006
- Hu CMJ, Zhang L, Aryal S, et al. Erythrocyte membrane-camouflaged polymeric nanoparticles as a biomimetic delivery platform. PNAS. 2011;108(27):10980–10985. doi: 10.1073/pnas.1106634108
- Rao L, Bu L-L, Xu J-H, et al. Red blood cell membrane as a biomimetic nanocoating for prolonged circulation time and reduced accelerated blood clearance. Small. 2015;11(46):6225–6236. doi: 10.1002/smll.201502388
- Gao W, Hu CMJ, Fang RH, et al. Surface functionalization of gold nanoparticles with red blood cell membranes. Adv Mater. 2013;25(26):3549–3553. doi: 10.1002/adma.201300638
- Hu CMJ, Fang RH, Wang KC, et al. Nanoparticle biointerfacing by platelet membrane cloaking. Nature. 2015;526(7571):118–121. doi: 10.1038/nature15373
- Parodi A, Quattrocchi N, van de Ven AL, et al. Synthetic nanoparticles functionalized with biomimetic leukocyte membranes possess cell-like functions. Nat Nanotech. 2013;8(1):61–8. doi: 10.1038/nnano.2012.212
- Teo KYW, Sevencan C, Cheow YA, et al. Macrophage polarization as a facile strategy to enhance efficacy of macrophage membrane‐coated nanoparticles in osteoarthritis. Small Sci. 2022;2(4):2100116–2100116. doi: 10.1002/smsc.202100116
- Toledano Furman NE, Lupu-Haber Y, Bronshtein T, et al. Reconstructed stem cell nanoghosts: a natural tumor targeting platform. Nano Lett. 2013;13(7):3248–3255. doi: 10.1021/nl401376w
- Zhu JY, Zheng DW, Zhang MK, et al. Preferential cancer cell self-recognition and tumor self-targeting by coating nanoparticles with homotypic cancer cell membranes. Nano Lett. 2016;16(9):5895–5901. doi: 10.1021/acs.nanolett.6b02786
- De Zorzi R, Mi W, Liao M, et al. Single-particle electron microscopy in the study of membrane protein structure. Microscopy (Tokyo). 2016;65(1):81–96. doi: 10.1093/jmicro/dfv058
- Lehninger AL, Nelson DL, Cox MM, editors. Lehninger principles of biochemistry. In Principles of biochemistry. 5th ed. New York: W.H. Freeman; 2008. p. 71–112.
- Fang RH, Hu CMJ, Chen KNH, et al. Lipid-insertion enables targeting functionalization of erythrocyte membrane-cloaked nanoparticles. Nanoscale. 2013;5(19):8884–8888. doi: 10.1039/c3nr03064d
- Dehaini D, Wei X, Fang RH, et al. Erythrocyte–platelet hybrid membrane coating for enhanced nanoparticle functionalization. Adv Mater. 2017;29(16):1–8. doi: 10.1002/adma.201606209
- de Ávila B E-F, Angsantikul P, Ramírez-Herrera DE, et al. Hybrid biomembrane–functionalized nanorobots for concurrent removal of pathogenic bacteria and toxins. Sci Rob. 2018;3(18):eaat0485. doi:10.1039/c3nr03064d
- Rao L, Meng QF, Huang Q, et al. Platelet–leukocyte hybrid membrane-coated immunomagnetic beads for highly efficient and highly specific isolation of circulating tumor cells. Adv Funct Mater. 2018;28(34):1–9. doi: 10.1002/adfm.201803531
- Li H, Jin K, Luo M, et al. Size dependency of circulation and biodistribution of biomimetic nanoparticles: red blood cell membrane-coated nanoparticles. Cells. 2019;8(8). doi: 10.3390/cells8080881
- Zhu J, Sevencan C, Zhang M, et al. Increasing the potential interacting area of nanomedicine enhances its homotypic cancer targeting efficacy. ACS Nano. 2020;14(3):3259–3271. doi: 10.1021/acsnano.9b08798
- Liu L, Bai X, Martikainen M-V, et al. Cell membrane coating integrity affects the internalization mechanism of biomimetic nanoparticles. Nat Commun. 2021;12(1):5726. doi: 10.1038/s41467-021-26052-x
- Ochoa-Villarreal M, Howat S, Hong S et al. Plant cell culture strategies for the production of natural products. BMB Rep. 2016;49(3):149–158. doi:10.5483/BMBRep.2016.49.3.264
- Kowalczyk T, Merecz-Sadowska A, Picot L, et al. Genetic manipulation and bioreactor culture of plants as a tool for industry and its applications. Molecules. 2022;27(3):795–823. doi: 10.3390/molecules27030795
- Joglekar S, Kodam K, Dhaygude M, et al. Novel route for rapid biosynthesis of lead nanoparticles using aqueous extract of Jatropha curcas L. latex. Mater Lett. 2011;65(19–20):3170–3172. doi:10.1021/acs.chemrev.6b00002
- Xie J, Lee JY, Wang DI, et al. Identification of active biomolecules in the high‐yield synthesis of single‐crystalline gold nanoplates in algal solutions. Small. 2007;3(4):672–682. doi:10.1002/smll.200600612
- Jebril S, Khanfir Ben Jenana R, Dridi C. Green synthesis of silver nanoparticles using Melia azedarach leaf extract and their antifungal activities: in vitro and in vivo. Materials Chemistry & Physics. 2020;248:122898. doi: 10.1016/j.matchemphys.2020.122898
- Gedda G, Sankaranarayanan SA, Putta CL, et al. Green synthesis of multi-functional carbon dots from medicinal plant leaves for antimicrobial, antioxidant, and bioimaging applications. Sci Rep. 2023;13. doi: 10.1038/s41598-023-33652-8
- Safdar M, Kim W, Park S, et al. Engineering plants with carbon nanotubes: a sustainable agriculture approach. J Nanobiotechnol. 2022 20;20(1). 10.1186/s12951-022-01483-w
- Gnawali CL, Shrestha LK, Hill JP, et al. Nanoporous activated carbon material from Terminalia chebula seed for supercapacitor application. C. 2023;9(4). doi: 10.3390/c9040109
- Shrestha RL, Chaudhary R, Shrestha T, et al. Nanoarchitectonics of lotus seed derived nanoporous carbon materials for supercapacitor applications. Materials. 2020;13(23):5434–5447. doi: 10.3390/ma13235434
- Shen Q, Huang Y, Bai H, et al. Polymer materials synthesized through Cell-mediated polymerization strategies for regulation of biological functions. Acc Mater Res. 2023;4(1):57–70. doi: 10.1021/accountsmr.2c00194
- Tu W, Zhou Y, Zou Z. Photocatalytic conversion of CO(2) into renewable hydrocarbon fuels: state-of-the-art accomplishment, challenges, and prospects. Adv Mater. 2014;26(27):4607–4626. doi: 10.1002/adma.201400087
- Mirkovic T, Ostroumov EE, Anna JM, et al. Light absorption and energy transfer in the antenna complexes of photosynthetic organisms. Chem Rev. 2017;117(2):249–293. doi: 10.1021/acs.chemrev.6b00002
- Wise RR. Plastids: the anabolic factories of plant cells. Encyclopedia Of Cell Biology. 2016;1:324–330.
- Khorobrykh S, Havurinne V, Mattila H, et al. Oxygen and ROS in photosynthesis. Plants (Basel). 2020;9(1). doi: 10.3390/plants9010091
- Ouyang J, Wang L, Chen W, et al. Biomimetic nanothylakoids for efficient imaging-guided photodynamic therapy for cancer. Chem Commun (Camb). 2018;54(28):3468–3471. doi: 10.1039/C8CC00674A
- Liu H, Lei Y, Nie W, et al. Immunomodulatory hybrid bio-nanovesicle for self-promoted photodynamic therapy. Nano Res. 2022. doi:10.1007/s12274-021-4050-3.
- Hou L, Gong X, Yang J, et al. Hybrid-membrane-decorated Prussian blue for effective cancer immunotherapy via tumor-associated macrophages polarization and hypoxia relief. Adv Mater. 2022;34(14):e2200389. doi: 10.1002/adma.202200389
- Zheng D, Li B, Xu L, et al. Normalizing Tumor microenvironment based on photosynthetic abiotic/biotic Nanoparticles. ACS Nano. 2018;12(6):6218–6227. doi: 10.1021/acsnano.8b02977
- Zheng DW, Xu L, Li CX, et al. Photo-powered artificial organelles for ATP generation and life-sustainment. Adv Mater. 2018;30(52):e1805038. doi: 10.1002/adma.201805038
- Chen P, Liu X, Gu C, et al. A plant-derived natural photosynthetic system for improving cell anabolism. Nature. 2022;612(7940):546–554. doi: 10.1038/s41586-022-05499-y
- Pucci C, Martinelli C, Degl’Innocenti A, et al. Light‐activated biomedical applications of chlorophyll derivatives. Macromol biosci. 2021;21(9):2100181. doi: 10.1002/mabi.202100181
- Chen M. Chlorophylls d and f: synthesis, occurrence, light-harvesting, and pigment organization in chlorophyll-binding protein complexes. In: Bernhard G, editor. Metabolism, Structure and Function of Plant Tetrapyrroles: Introduction, Microbial and Eukaryotic Chlorophyll Synthesis and Catabolism. London: Academic Press; 2019. p. 121–139.
- Murchie EH, Lawson T. Chlorophyll fluorescence analysis: a guide to good practice and understanding some new applications. J Exp Bot. 2013;64(13):3983–3998. doi: 10.1093/jxb/ert208
- Ruiz-Hitzky E, Darder M, Aranda P, et al. Advances in biomimetic and nanostructured biohybrid materials. Adv Mater. 2010;22(3):323–336. doi: 10.1002/adma.200901134
- Sakakibara K, Granström M, Kilpeläinen I, et al. Light-harvesting nanorods based on pheophorbide-appending cellulose. Biomacromolecules. 2013;14(9):3223–3230. doi: 10.1021/bm400858v
- Pucci C, Martinelli C, Degl’innocenti A, et al. Light-activated biomedical applications of chlorophyll derivatives. Macromol biosci. 2021;21(9):2100181. doi: 10.1002/mabi.202100181
- Li X, Kwon N, Guo T, et al. Innovative strategies for hypoxic-tumor photodynamic therapy. Angew Chem Int Ed. 2018;57(36):11522–11531. doi: 10.1002/anie.201805138
- Ethirajan M, Chen Y, Joshi P, et al. The role of porphyrin chemistry in tumor imaging and photodynamic therapy. Chem Soc Rev. 2011;40(1):340–362. doi: 10.1039/B915149B
- Lanfer-Marquez UM, Barros RMC, Sinnecker P. Antioxidant activity of chlorophylls and their derivatives. Food Res Int. 2005;38(8–9):885–891. doi: 10.1016/j.foodres.2005.02.012
- Perez-Galvez A, Viera I, Roca M. Carotenoids and chlorophylls as antioxidants. Antioxidants (Basel). 2020;9(6). doi: 10.3390/antiox9060505
- Calixto GM, Bernegossi J, de Freitas LM, et al. Nanotechnology-based drug delivery systems for photodynamic therapy of cancer: a review. Molecules. 2016;21(3):342. doi: 10.3390/molecules21030342
- Brandis AS, Salomon Y, Scherz A, et al. Chlorophyll sensitizers in photodynamic therapy. In: Grimm B, editor. Chlorophylls and bacteriochlorophylls. Dordrecht: Springer Netherlands; 2006. p. 461–483.
- Gerola AP, Santana A, França PB, et al. Effects of metal and the phytyl chain on chlorophyll derivatives: physicochemical evaluation for photodynamic inactivation of microorganisms. Photochem Photobiol. 2011;87(4):884–894. doi: 10.1111/j.1751-1097.2011.00935.x
- Chernomorsky S, Segelman A, Poretz RD. Effect of dietary chlorophyll derivatives on mutagenesis and tumor cell growth. Teratog Carcinog Mutagen. 1999;19(5):313–322. doi: 10.1002/(SICI)1520-6866(1999)19:5<313::AID-TCM1>3.0.CO;2-G
- Hajri A, Wack S, Meyer C, et al. In vitro and in vivo efficacy of Photofrin® and pheophorbide a, a bacteriochlorin, in photodynamic therapy of colonic cancer Cells. Photochem Photobiol. 2007;75(2):140–148. doi: 10.1562/0031-8655(2002)0750140IVAIVE2.0.CO2
- Rizzi V, Fini P, Fanelli F, et al. Molecular interactions, characterization and photoactivity of chlorophyll a/chitosan/2-HP-β-cyclodextrin composite films as functional and active surfaces for ROS production. Food Hydrocolloids. 2016;58:98–112. doi: 10.1016/j.foodhyd.2016.02.012
- Rizzi V, Fini P, Semeraro P, et al. Detailed investigation of ROS arisen from chlorophyll a/chitosan based-biofilm. Colloids Surf B. 2016;142:239–247. doi: 10.1016/j.colsurfb.2016.02.062
- Suvorov N, Pogorilyy V, Diachkova E, et al. Derivatives of natural chlorophylls as agents for antimicrobial photodynamic therapy. Int J Mol Sci. 2021;22(12):6392. doi: 10.3390/ijms22126392
- Dashwood R, Negishi T, Hayatsu H, et al. Chemopreventive properties of chlorophylls towards aflatoxin B1: a review of the antimutagenicity and anticarcinogenicity data in rainbow trout. Mutat Res. 1998;399(2):245–253. doi: 10.1016/S0027-5107(97)00259-5