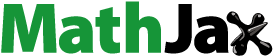
Abstract
In this work, two bidentate 2-pyridyl-1,2,3-triazole ligands (3a and 3b) containing a 4-substituted benzenesulfonamide pharmacophore prepared by classical click chemistry procedures, as well as their corresponding rhenium complexes, 4a and 4b of general formula [ReCl(CO)3(L)] (L = 3a or 3b) were prepared and fully characterised by spectroscopic methods (IR, NMR, MS, UV-Vis), elemental analysis, X-ray diffraction, and theoretical studies using DFT and TD-DFT methods. In particular, we showed that, in the solid state, the pyridine and the triazole rings of 3b adopted an uncommon cis configuration which stems from intermolecular hydrogen bonds. Preliminary assays demonstrated a promising nanomolar inhibitory activity against carbonic anhydrase isoform IX for both ligands and complexes with a strong affinity Ki of 2.8 nM for ligand 3a. More interestingly, complex 4b exhibited a pronounced selectivity against hCA IX over the off-targets hCA I and hCA II which makes this compound a promising potential anticancer drug candidate.
1. Introduction
Over recent decades, rhenium(I) tricarbonyl complexes have been intensively studied by the inorganic chemist community, due to their significant photophysical and photochemical properties. These features make them interesting tools for numerous potential practical applications, such as photo sensitisers in solar cellsCitation1, CO2 reduction catalystsCitation2, organic light-emitting devices (OLEDs)Citation3, luminescence sensorsCitation4, and CO-releasing moieties (CORMs)Citation5. Additionally, radioactive fac-[188Re(CO)3]+ complexes have recently drawn the attention of several research groups for their use as therapeutic radiopharmaceuticalsCitation6.
In this context, the efficient design and synthesis of chelating ligands represent the pivotal key to the successful application of this type of complexes. Most of the reported ligands which coordinate efficiently to the rhenium(I) tricarbonyl core are based on bidentate and tridentate chelating systems including N-heteroaromatic nitrogen, oxygen and to a lesser extend sulfur or phosphorus donor atomsCitation7. While tridentate ligands lead to the most stable rhenium(I) tricarbonyl complexes, bidentate ligands based on α,α'-diimines are currently very popular. Indeed, their corresponding rhenium complexes exhibit interesting photo-physical properties which can be tuned by small changes in the ligand scaffold or around the rhenium centre by substituting the chlorine bound to the metal by a ternary ligand (generally, cyanides or N-heterocycles)Citation8. Among efficient bidentate α,α'-diimine chelators, 2,2'-bipyridine (bpy) has been originally usedCitation9. More recently, similar chelating systems based on pyridine-triazole (pyta and tapy systems1) were developed as alternative ligands to 2,2′-bipyridinesCitation10. Obata et al. first reported that pyta moieties acted like a bipyridine mimic with strongly electron-donating substituents, the corresponding complexes of general formula fac-[Re(pyta)(CO)3Cl] exhibiting luminescence propertiesCitation11.
The growing interest on pyta (and tapy) architectures is mainly due to their easier synthetic access and functionalization. Unlike bpy systems, it is possible by using a classical copper-catalysed alkyne-azide cycloaddition (CuAAC reaction) not only to generate a large number of this important class of heterocyclic compounds but also to allow different functionalizations of the triazole ringCitation12. As an example, we previously reported the preparation of two [M(CO)3]+ complexes (M = Re and 99mTc) from a pyridyltriazole-based ligand bearing the bioactive (2-methoxyphenyl) piperazine pharmacophore, which is a central nervous system (CNS) receptors-targeted vector. Thus, we demonstrated that (i) both complexes were iso-structural, as expected, (ii) the rhenium complex exhibited fluorescence at room temperature and the radioactive 99mTc-complex presented a suitable lipophilic character for its use as CNS imaging agentCitation12a. Given these first results, we decided to explore similar systems as potential human carbonic anhydrase inhibitors (CAIs) by adding a heterocyclic sulfonamide moiety to a pyta scaffold.
While sulfonamides (and bioisosteres sulfamates and sulfamides) are known to possess very efficient carbonic anhydrase inhibitory propertiesCitation13, only a few examples of benzenesulfonamide-based compounds incorporating a [M(CO)3]+ complex (M = Re and 99mTc) have been reportedCitation14. Among these examples, Alberto and coworkers showed that simple piano-stool-type rhenium complexes with a pendent arylsulfonamide arm inhibited hCA IX and hCA XII with nanomolar affinitiesCitation14c. Using a similar strategy, i.e. coupling a simple and compact rhenium complex to a 4-substituted benzenesulfonamide moiety, we report here the synthesis of two tricarbonyl rhenium(I) conjugates based on a pyta ligand as well as their full characterisation by spectroscopic methods, X-ray crystallography study and DFT calculations. Preliminary biological assays against cytosolic/membrane-associated carbonic anhydrase isoforms I, II and IX (hCAs I, II and IX) were performed with both ligands and complexes and first results are promising.
2. Experimental section
2.1. Materials and equipment
All purchased chemicals were of the highest purity commercially available and used without further purification. Analytical grade solvents were used and were not further purified unless specified. Starting materials 1a and 1b were purchased from Aldrich Chem. Co. [Re(CO)5Cl] was purchased from Acros Organics.
1H and 13 C nuclear magnetic resonance (NMR) spectra were recorded with a Bruker Avance 300 (75.5) MHz; chemical shifts are reported in parts per million (ppm) relative to a residual solvent peak and coupling constants (J) are given in Hertz (Hz). Multiplicities were recorded as s (singlet), br s (broad singlet), d (doublet), t (triplet), q (quadruplet) … and m (multiplet). Infra-red (IR) spectra were recorded with Perkin–Elmer FTIR 1725 spectrophotometer in the range 4000–400 cm−1. Electrospray (ESI) mass spectra were obtained on a Q TRAP Applied Biosystems spectrometer and High-Resolution Mass Spectra (HRMS) on an LCT Premier Waters spectrometer. Microanalysis was performed by the microanalytical department of the Laboratoire de Chimie de Coordination de Toulouse (LCC, Toulouse, France). Electronic spectrum was measured on a Hewlett Packard 8453 temperature-controlled spectrometer in the range 1000–200 nm in methanol solution. Melting points were measured in capillaries using Mettler Toledo and are reported as uncorrected.
Literature methods were used to prepare intermediates 2a and 2bCitation15 (if azido compounds are potentially explosive intermediates, in our hands, we never observed hazardous reactions for both azido intermediates).
2.2. Syntheses
2.2.1. 4-Azidobenzenesulfonamide (2a).
Yield: 267 mg (93%). 1H NMR (300 MHz, DMSO-d6): δ/ppm = 7.29 (d, J = 8.7 Hz, 2H, HAr), 7.36 (s, 2H, NH2), 7.82 (d, J = 8.6 Hz, 2H, HAr). Spectroscopic analysis is in agreement with reported dataCitation15.
2.2.2. 4-(Azidomethyl)-benzenesulfonamide (2b).
Yield: 391 mg (85%). 1H NMR (300 MHz, DMSO-d6): δ/ppm = 4.56 (s, 2H, NCH2), 7.37 (s, 2H, NH2), 7.55 (d, J = 8.7 Hz, 2H, HAr), 7.83 (d, J = 8.6 Hz, 2H, HAr). Spectroscopic analysis is in agreement with reported dataCitation15.
General procedure for CuAAC reaction: Azide compound (2a or 2b, 1.1 equiv.) and 2-ethynylpyridine (1 equiv.) were suspended in acetonitrile (10 ml). Copper(II) acetate monohydrate (0.2 equiv) and sodium ascorbate (0.4 equiv) were added and the mixture is stirred in the dark at 45 °C overnight (16–18 h). The solvent was then removed under reduced pressure and remaining residues purified by column chromatography on silica gel using CH2Cl2/MeOH (95:5) as eluent.
2.2.3. 4-(4–(2-pyridyl)-1H-1,2,3-triazol-1-yl)-benzenesulfonamide (3a)
Two fifty milligams (1.26 mmol) of 2a and 116 μL (1.15 mmol) of 2-ethynylpyridine with 46 mg (0.23 mmol) of Cu(OAc)2.H2O and 91.4 mg (0.46 mmol) of sodium ascorbate yielded the desired compound 3a as a yellow solid. Suitable crystals of 3a for X-ray crystal structure determination were grown by slow evaporation of methanol solution.
Yield: 236 mg (69%). mp 244–248 °C. 1H NMR (300 MHz, DMSO-d6): δ/ppm = 7.43 (ddd, J = 7.6, 4.8, 1.2 Hz, 1H, Hpyr), 7.54 (s, 2H, NH2), 7.97 (td, J = 7.7, 1.8 Hz, 1H, Hpyr), 8.04 (d, J = 8.7 Hz, 2H, HAr), 8.14 (dt, J = 7.9, 1.1 Hz, 1H, Hpyr), 8.26 (d, J = 8.7 Hz, 2H, HAr), 8.63-8.71 (m, 1H, Hpyr), 9.46 (s, 1H, Hta). 13 C NMR (75 MHz, DMSO-d6): δ/ppm = 119.9, 123.5, 137.4, 149.2 (CHpyr), 120.5, 127.5 (CHAr), 121.6 (CHta) 138.6, 144.0 (CAr), 148.5 (Cpyr), 149.8 (Cta). ESI+-MS: m/z = 302.1 [M + H]+, 324.1 [M + Na]+. HRMS calculated for C13H12N5O2S ([M + H]+) 302.0712; found 302.0716. Elemental analysis for C13H11N5O2S: calculated (found) C, 51.82 (51.64); H, 3.68 (3.63); N, 23.24 (22.26).
2.2.4. 4–(4-(2-pyridyl)-1H-1,2,3-triazol-1-ylmethyl)-benzenesulfonamide (3b)
Two twenty milligrams (1.04 mmol) of 2b and 95 μL (0.94 mmol) of 2-ethynylpyridine with 38 mg (0.19 mmol) of Cu(OAc)2.H2O and 75.5 mg (0.38 mmol) of sodium ascorbate yielded the desired compound 3b as a yellow solid. Suitable crystals of 3b for X-ray crystal structure determination were grown by slow evaporation of methanol solution.
Yield: 180 mg (61%). mp 192–195 °C. 1H NMR (300 MHz, DMSO-d6): δ/ppm = 5.78 (br s, 2H, NCH2), 7.29–7.41 (m, 3H, Hpyr, NH2), 7.53 (d, J = 8.7 Hz, 2H, HAr), 7.83 (d, J = 8.7 Hz, 2H, HAr),7.90 (ddd, J = 7.9, 7.5, 1.8 Hz, 1H, Hpyr), 8.04 (dt, J = 7.9, 1.1 Hz, 1H, Hpyr), 8.60 (ddd, J = 4.8, 1.8, 1.0 Hz, 1H, Hpyr), 8.73 (s,1H, Hta). 13 C NMR (75 MHz, DMSO-d6): δ/ppm = 52.4 (CH2), 119.4, 123.7, 137.2, 149.6 (CHpyr), 123.1 (CHta), 126.2, 128.4 (CHAr), 139.7, 143.9 (CAr), 147.6 (Cpyr), 149.8 (Cta). ESI+-MS: m/z = 316.1 [M + H]+. HRMS calculated for C14H14N5O2S ([M + H]+) 316.0868, found 316.0868. Elemental analysis for C14H13N5O2S: calculated (found) C, 53.32 (53.19); H, 4.16 (3.94); N, 22.21 (21.49).
General procedure for complexation reaction: A solution of 3a/3b (1 equiv) and commercial [Re(CO)5Cl] (1.1 equiv.) in methanol (8 ml) was stirred 12 h at 65 °C. After cooling to room temperature, the solution was concentrated until 3 ml and then a precipitate was obtained. Five millilitres of methanol was added to the precipitate. The mixture was heated, then cooled and stored at 4 °C for 3 h and then a supernatant was carefully removed. This process was repeated three times before the precipitate was dried under vacuum.
2.2.5. [(3a)Re(CO)3Cl], (4a)
3a (70 mg, 0.23 mmol) and [Re(CO)5Cl] (92.5 mg, 0.25 mmol) yielded the desired complex 4a as a white solid. Suitable crystals of 4a for X-ray crystal structure determination were grown by slow evaporation of methanol solution.
Yield: 95 mg (67%). mp >300 °C. 1H NMR (300 MHz, DMSO-d6): δ/ppm = 7.64 (s, 2H, NH2), 7.71 (ddd, J = 7.3, 5.5, 1.6, 1H, Hpyr), 8.14 (d, J = 8.8 Hz, 2H, HAr), 8.25 (d, J = 8.8 Hz, 2H, HAr), 8.31 (ddd, J = 7.9, 1.6, 0.8 Hz, 1H, Hpyr), 8.38 (td, J = 7.7, 1.5 Hz, 1H, Hpyr), 9.04 (dd, J = 5.6, 1.2 Hz, 1H, Hpyr), 10.08 (s, 1H, Hta). 13 C NMR (75 MHz, DMSO-d6): δ/ppm = 121.4, 127.8 (CHAr), 122.7, 126.9, 137.4, 149.2 (CHpyr), 124.6 (CHta), 141.1, 145.5 (CAr), 148.3 (Cpyr), 153.3 (Cta), 189.4, 196.5, 197.4 (CO). IR: νCO=2027, 1931, 1905 cm−1, ESI+-MS: m/z = 570.0 [M-Cl]+, 627.9 [M + Na]+. HRMS calculated for C16H11N5O5SClRe ([M + Na]+) 627.9597, found 627.9616. Elemental analysis for C16H11N5O5SClRe: calculated (found) C, 31.66 (31.37); H, 1.83 (1.44); N, 11.54 (11.10).
2.2.6. [(3b)Re(CO)3Cl], (4b)
3b (75 mg, 0.238 mmol) and [Re(CO)5Cl] (95 mg, 0.26 mmol) yielded the desired complex 4b as a white solid. Suitable crystals of 4b for X-ray crystal structure determination were grown by slow evaporation of methanol solution.
Yield: 98 mg (66%). mp 268–271 °C. 1H NMR (300 MHz, DMSO-d6): δ/ppm = 6.00 (s, 2H, NCH2), 7.41 (s, 2H, NH2), 7.60–7.68 (m, 3H, 1Hpyr, 2HAr), 7.90 (d, J = 8.3 Hz, 2H, HAr), 8.19–8.37 (m, 2H, 2Hpyr), 8.96 (dd J = 5.4, 1.3 Hz, 1H, Hpyr), 9.31 (s, 1H, Hta). 13 C NMR (75 MHz, DMSO-d6): δ/ppm = 54.1 (CH2), 122.8, 126.6, 137.8, 148.6 (CHpyr), 126.2 (CHta), 126.4, 129.2 (CHAr), 140.7, 144.5 (CAr), 148.6 (Cpyr), 153.0 (Cta), 189.5, 196.7, 197.5 (CO). IR (KBr): νCO=2029, 1920, 1902 cm−1. ESI+-MS: m/z = 584.0 [M-Cl]+, 642 [M + Na]+. HRMS calculated for C17H13N5O5NaSClRe ([M + Na]+) 641.9753, found 641.9750. Elemental analysis for C17H13N5O5SClRe: calculated (found) C, 32.88 (32.79); H, 2.11 (1.84); N, 11.28 (10.97).
2.3. Crystal structure determination
X-ray intensity data of ligands 3a, 3b and corresponding rhenium complexes 4a, 4b were collected on a Bruker D8 VENTURE diffractometer (MA, USA) and using graphite monochromated Mo Kα radiation (λ = 0.71073 Å) for 3b, 4a and 4b and Cu Kα radiation (λ = 1.54178 Å) for 3a at 193 K. The semi-empirical absorption corrections were employed [SADABS, Programme for data correction, Bruker-AXS]. Crystallographic data and refinement details are given in . The structures were solved using an intrinsic phasing methodCitation16, and refined by full matrix least squares procedures on F2. All non-hydrogen atoms were refined with anisotropic displacement coefficients. The hydrogen atoms bound to carbon atoms were placed in calculated positions and treated as riding on their parent atoms with d(C–H)=0.93 Å, Uiso(H)=1.2 Ueq(C) (aromatic); and d(C–H)=0.96 Å, Uiso(H)=1.5 Ueq(C) (methyl). The methyl groups were allowed to rotate about their local threefold axis. The ShelX software packageCitation16 was used for the calculations.
Table 1. Crystal data and structure refinement for 3a, 3b, 4a, and 4b.
Table 2. Inhibition data of human CA I, II and IX isoforms with compounds 3a, 3b, 4a, and 4b in comparison with the standard sulfonamide inhibitor acetazolamide (AAZ) by a stopped-flow CO2 hydrase assay.
CCDC 1871452 (3a), CCDC 1871453 (3b), CCDC 1871454 (4a) and CCDC 1871455 (4b) contain the supplementary crystallographic data. These data can be obtained free of charge from http://www.ccdc.cam.ac.uk/conts/retrieving.html, or from the Cambridge Crystallographic Data Centre, 12 Union Road, Cambridge CB2 1EZ, UK; tel: +44 (0)1223 336408; fax: +44 (0)1223 336033; or e-mail: [email protected].
2.4. Computational details
The reported calculations for the studied tricarbonyl rhenium(I) complexes 4a and 4b were carried out using the GAUSSIAN 09 programme packageCitation17 with the aid of the GaussView visualisation programCitation18. Full geometries optimizations were performed in gas-phase and methanol solvent without any symmetry restrictions with the DFT method using the hybrid B3LYP (Becke, three-parameter, Lee-Yang-Parr) functionalCitation19. The pseudo-potential (ECP) LANL2DZCitation20 was employed to describe the electrons of Re atom while the other atoms (H, C, O, N and S) were assigned by the standard 6–31 G (d) basis set. The optimised geometries were evaluated using vibrational frequencies calculations to ensure that the true local minimum was attained and all eigenvalues are positive. Natural bond orbital analysis was carried out using NBO code included in GAUSSIAN 09Citation21. At the optimised structures, time-dependent density functional theory (TD-DFT) methodCitation22 using B3LYP functional was applied to calculate the vertical excitation energies and corresponding oscillator strengths. In addition, the solvent effect (methanol) was modelled using the polarisable continuum model with the integral equation formalism (IEF–PCM)Citation23. The initial geometries were taken from X-ray structures, and all calculations were based on the optimised geometries.
2.5. Carbonic anhydrase inhibition assays
An Applied Photophysics stopped-flow instrument was used for assaying the CA catalysed CO2 hydration activity. Phenol red (at a concentration of 0.2 mM) was used as indicator, working at the absorbance maximum of 557 nm, with 20 mM Hepes (pH 7.5) as buffer, and 20 mM Na2SO4 (for maintaining the constant ionic strength), following the initial rates of the CA-catalysed CO2 hydration reaction for a period of 10–100 s. The CO2 concentrations ranged from 1.7–17 mM for the determination of the kinetic parameters and inhibition constants. In particular, CO2 was bubbled in distilled deionised water for 30 min so that the water was saturated (the concentration at a specific temperature is known from literature). In addition, a CO2 assay kit (from Sigma) was used to measure the concentration in variously diluted solutions obtained from the saturated one (which was kept at the same temperature and a constant bubbling during the experiments). For each inhibitor at least six traces of the initial 5–10% of the reaction was used for determining the initial velocityCitation24. The uncatalysed rates were determined in the same manner and subtracted from the total observed rates. Stock solutions of inhibitor (0.1 mM) were prepared in distilled-deionised water and dilutions up to 0.01 nM were done thereafter with distilled-deionised water. Inhibitor and enzyme solutions were pre-incubated together for 15 min–2 h (or longer, i.e. 4–6 h) at room temperature (at 4 °C for the incubation periods longer than 15 min) prior to assay, in order to allow for the formation of the E-I complex. The inhibition constants were obtained by non-linear least-squares methods using PRISM 3 and the Cheng-Prusoff equationCitation25,Citation26, as reported earlier, and represent the mean from at least three different determinations. hCA I was purchased by Sigma-Aldrich and used without further purification, whereas all the other hCA isoforms were recombinant ones obtained in-house as reported earlierCitation27,Citation28.
3. Results and discussion
3.1. Synthesis and structural characterisation
The synthesis of rhenium tricarbonyl complexes 4a and 4b were readily synthesised following a three-step procedure as depicted in Scheme 1. The ligands 3a and 3b were prepared by classical copper-catalysed Huisgen cycloaddition (CuAAC reaction) using the corresponding azido intermediates and commercial 2-ethynylpyridine derivatives. Both azido compounds 2a and 2b were obtained in good yields (>80%) by following a procedure reported earlierCitation15. By applying classical CuAAC conditions we previously reported for pyta ligands preparationCitation10b (i.e. catalytic system: Cu(OAc)2.H2O/sodium ascorbate in tert-butanol/water mixture or in acetonitrile at room temperature), 3a and 3b were obtained in low yield or at trace level. In turn, the yield of the cycloaddition was noticeably improved by performing the reaction at 45 °C in acetonitrile (average yield of 65%). Pyta-based rhenium complexes 4a and 4b were finally obtained in modest yields (c.a. 66%) by the reaction of commercial rhenium pentacarbonyl chloride with chelating ligands in refluxing methanol during 12 h. All our compounds are stable in the solid state as well as in organic solvents and their stability is not affected by the presence of air or moisture. They were fully characterised by NMR, IR, MS and elemental analysis and exhibited classical spectroscopic features for pyta-based compounds (see experimental section and ESI part, Tables S1–2 for NMR data). Briefly, upon complexation with the tricarbonyl rhenium core, a significant down-field shift (∼0.6 ppm) of the singlet due to the triazole proton and very minor shifts of the hydrogens of the phenyl ring compared to those of the free ligand were observed in proton NMR for each complex, confirming the coordination of the rhenium by the triazolyl unit and the pendent nature of the 4-substituted arylsulfonamide moiety. The pattern of the CO stretching frequencies in the IR spectra (three intense bands in the 2030–1900 cm−1 region) confirms the facial arrangement of the CO ligands in the complexes. More interestingly, as reported recently by Sarkar et al., the average value of the CO stretching frequencies gave information on the overall donor strength of the chelating pyta pincerCitation29. In our case, both complexes exhibited an average value of 1950 cm−1, this value being consistent with those reported for other pyta ligandsCitation29 and indicating an overall donor strength similar to bpy ligands. Fortunately, we obtained crystals of ligands 3a/3b and complexes 4a/4b suitable for single crystal X-ray diffraction from the slow evaporation of a concentrated sample in methanol solution. Selected crystallographic parameters are listed in and selected bond lengths and angles values are gathered in the ESI part (Tables S3–S5).
Scheme 1. Synthesis of ligands 3a/3b and their corresponding benzenesulfonamide rhenium tricarbonyl complexes 4a/4b. Conditions and reagents: (i) 1a, NaNO2, NaN3, HCl/THF/DMF (v/v/v: 1:1:1), 0–25 °C, 1 night (93%) or 1b, NaN3, Tf2O, CuSO4.5H2O, K2CO3, CH2Cl2, 0–25 °C, 1 night (85%); (ii) Cu(OAc)2.H2O, sodium ascorbate, CH3CN, 45 °C, 1 night, (3a: 69%, 3b: 61%); (iii) [Re(CO)3Cl], 65 °C, MeOH, 12h, (4a: 67%, 4b: 66%).
![Scheme 1. Synthesis of ligands 3a/3b and their corresponding benzenesulfonamide rhenium tricarbonyl complexes 4a/4b. Conditions and reagents: (i) 1a, NaNO2, NaN3, HCl/THF/DMF (v/v/v: 1:1:1), 0–25 °C, 1 night (93%) or 1b, NaN3, Tf2O, CuSO4.5H2O, K2CO3, CH2Cl2, 0–25 °C, 1 night (85%); (ii) Cu(OAc)2.H2O, sodium ascorbate, CH3CN, 45 °C, 1 night, (3a: 69%, 3b: 61%); (iii) [Re(CO)3Cl], 65 °C, MeOH, 12h, (4a: 67%, 4b: 66%).](/cms/asset/af5f74af-b9a7-4bd0-ba84-3de95511b905/ienz_a_1585835_sch0001_b.jpg)
Both ligands (3a and 3b) were crystallized in triclinic and monoclinic system and their crystals were solved in space groups P and P21/c, respectively (see ORTEP diagrams in ESI part, Figure S1). As expected, the structural characteristics of 3a/3b are similar to those reported for related pyta ligandsCitation10a,b,Citation11,Citation12,Citation30. The azo character of the triazolyl ring is confirmed by the N(2)–N(3) distance of the 1,2,3-triazole unit which is shorter than the N(3)–N(4) and N(2)–C(6) bonds (1.314(3) Å vs. 1.357(3) Å and 1.366(4) Å for 3a with similar bond length values for both molecules of the asymmetric unit, and 1.313(2) Å vs. 1.343(2) Å and 1.363(2) Å for 3b.
While 3b is essentially planar with an angle between the least-square planes of the 2-pyridyl and triazole rings of 8.1(1)°, 3a displays an exceptional deviation between the pyridyl part and the triazolyl entity. The values of the dihedral angle (33.3(1)° and 27.9(1)° for both molecules of the asymmetric unit) are the largest observed for the pyta scaffold compared to the previously reported values (range from 1.56°–15°)Citation10a,Citation11,Citation30. In turn, one of the two molecules of 3a exhibits a singular planar geometry with respect to the triazolyl moiety and the phenyl group of the benzenesulfonamide unit (dihedral angle value of 3.2(1)° vs. 30.3(2)° for the second molecule of the asymmetric unit). Generally, in pyta scaffold bearing a phenyl group directly connected to the triazole ring, the dihedral angle between the pyridyl and triazole rings is shorter than that between the triazole and phenyl rings (dihedral angle value: c.a. 20–30°)Citation11,Citation30a. A network of inter-molecular hydrogen interactions (see ESI part, Table S6) and π–π interactions could explain these unexpected structural features for 3a (see ESI part, Table S7).
More interestingly, while the nitrogen atoms N(1)/N(2) and N(6)/N(7) of 3a exhibits a classical anti configurationCitation11,Citation12,Citation30 with minimal electronic repulsions between both nitrogens, an unprecedented cis arrangement is clearly adopted by the nitrogen atoms of the pyridine and triazole rings of ligand 3b, as illustrated in . Stabilisation through a network of non-covalent interactions has been invoked to explain thus unexpected arrangement. The single-crystal X-ray structure of 3b shows two types of hydrogen-bonding interactions (see ESI part, Table S6): stronger intermolecular hydrogen-bonding interactions of the type N–H···N [2.939(2) Å] between the N(5)–H(5b) proton of the sulfonamide moiety and the N(1) atom of the pyridine ring, as well as C–H···O [3.260(2) Å] between the C(7)–H(7) proton of the 1,2,3-triazolyl group and O(1) atom of the sulfonamide moiety. π–π interactions have also been detected in the crystallographic structure for 3b (see ESI part, Table S7).
Figure 1. Unprecedented cis-configuration of the 2-pyridyl-1,2,3-triazole unit of ligand 3b; a partial view of the crystal packing of 3b with N–H•••N and C–H•••O hydrogen bonds and π–π contacts shown as dashed lines. The purple spheres represent the centroids of the rings involved in π–π interactions.
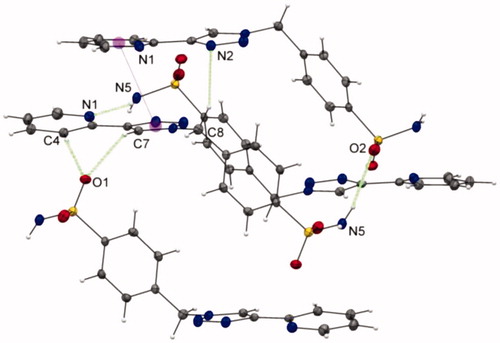
Rhenium complexes 4a and 4b shown in (molecular views), crystallise as neutral molecular complexes in the orthorhombic space groups Pbcn and triclinic space groups P respectively. As suggested by the spectroscopic data, each rhenium centre adopts a distorted octahedral coordination geometry. The rhenium atoms are coordinated to two nitrogens of the pyta pincer, three carbonyl donors in a fac-orientation and one chlorine atom. The bond lengths and angles for both complexes 4a and 4b are unexceptional and similar to those previously reported for other rhenium tricarbonyl complexes based on bidentate ligandsCitation10–12,Citation30. As an example, the average Re-carbonyl bond length is 1.92 Å, and the OC–Re–CO angles range from 87.4(2)° to 90.3(2)° and 88.8(1)° to 90.3(1)° for 4a and 4b, respectively. The trans bond angles exhibit a moderate distortion from an idealised octahedral geometry (range of 170.3–179.0°), as expected. The Re–Cl bond lengths [2.490(1) Å for 4a, 2.485(1) Å for 4b] lie in the upper side of the range (2.454–2.505 Å) found for other rhenium tricarbonyl complexes based on pyta derivativesCitation10g,Citation11,Citation12a. For both complexes 4a and 4b, the chelate part is essentially planar with a very slight deviation of 2.5(1)° and 8.2(2)° between the mean planes through the triazolyl and pyridyl rings, respectively. As observed previously, the co-planarity was extended to the benzenesulfonamide pendent arm in 4a. The 4-substituted aromatic arm shows a dihedral angle of 2.1(1)° with the mean plane of the triazolyl ring, as a result of conjugationCitation10b,Citation31. In both complexes, a network of π–π interactions and inter-molecular hydrogen bonds between two adjacent complex molecules for 4a and between two adjacent complex molecules plus the lattice methanol molecule for 4b are involved in the crystal cohesion (see ESI part, Table S6, and Figure S2).
3.2. Computational study
The density functional theory (DFT) and time-dependent density functional theory (TDDFT) calculations were performed in order to assess the impact of the methylene linker (−CH2−) between the benzenesulfonamide moiety and the chelate unit of 3b on the electronic structures of its rhenium complexes (See Tables S8–S10 and Figure S3 in ESI part for more details). While HOMOs energy levels exhibit small changes, the introduction of the methylene spacer in the complex 4b has an effect on LUMOs energy, as illustrated in . Compared to 4a, the introduction of this methylene linker increased the energy level of LUMO (−2.29 eV) causing wider energy gap (3.22 eV for 4b vs. 3.09 eV for 4a) and a slight blue-shift of the lowest energy absorption band (331 nm for 4b vs. 333 nm for 4a). The lowest virtual orbitals of complexes are mainly concentrated on the π-anti-bonding orbitals of the chelate and CO ligands. The lowest virtual molecular orbital (LUMO) is centred on π-anti-bonding orbitals of the 2-pyridyl-1,2,3-triazole part and CO ligands. The LUMO + 1 and LUMO + 4 are mainly localised on the full pyta ligand for both complexes. The LUMO + 2 is located on the 2-pyridyl-1,2,3-triazole unit in 4a, and with a slight contribution from the phenyl ring in the case of 4b. The LUMO + 3 is wholly centred on phenyl ring for the complex 4a, whereas for 4b, it is localised on the full connected ligand.
For 4a and 4b, the low-energy absorption bands at 333 and 331 nm have mixed metal-to-ligand [MLCT] and ligand-to-ligand [LLCT] charge transfer character. Accordingly, these electronic transitions originate mainly from the mixed orbitals of the rhenium centre and chlorine to the π-antibonding orbitals of the chelating ligand and carbonyl group, which can be described as {d/π(Cl) → π*(L)/π*(CO) or π*(L)}. The band assignments were presented like those previously reported by other researchers, for related Re(CO)3-complexes with similar bidentate ligandsCitation32.
3.3. Carbonic anhydrase inhibitory activity
As mentioned in the introduction, the group of Alberto demonstrated that compact tricarbonyl rhenium complexes acted as excellent carbonic anhydrase IX and XII inhibitors, with nanomolar affinitiesCitation14c. More recently, Tim Storr et al., reported that tricarbonyl rhenium complexes based on a dipyridylamine (DPA) or an iminodiacetate (IDA) ligands with a pendent benzenesulfonamide pharmacophore exhibited interesting CA-IX inhibition (KI of 37 nM for the best candidate)Citation14d.
In our case, both ligands 3a and 3b and their corresponding complexes 4a and 4b were tested for their efficacy to inhibit physiologically relevant hCA I, II and the tumour-associated hCA IX isoforms, as a preliminary study. The synthesised compounds were screened for their inhibition potential by means of stopped-flow carbon dioxide hydration assay and compared with the clinically used reference drug acetazolamide (AAZ) ().
Both complexes 4a and 4b and the reference drug acetazolamide (AAZ) exhibited similar nanomolar affinities (ca. 25 nM) against hCA IX isozyme. Compared to reported affinity values with different tricarbonyl rhenium systems bearing an arylsulfonamide moiety, our values are better than those recently reported by the group of Storr (37–220 nM), in the lower range than those obtained by Babich et al. (3–116 nM) but higher than those obtained by Alberto et al. (3–7 nM)Citation14b–d. The introduction of a methylene group between the metallic part and the sulfonamide unit has a slight negative effect on the inhibition values (18.7 nM for 4a vs. 27.3 nM for 4b), as previously reportedCitation14c. Additionally, a better inhibition is observed with ligands 3a and 3b compared to their corresponding metallic analogs. In particular, a very strong inhibition is found for ligand 3a, which displayed 7-fold higher affinity than its rhenium complex 4a (Ki(3a)=2.8 nM vs. Ki(4a)=18.7 nM). This unexpected result should be confirmed on other hCA isoforms, the non-metaled free ligands exhibiting generally weaker affinity than that of the corresponding rhenium complexesCitation14b. In turn, this first study showed that both rhenium complexes were highly inactive against hCA I and exhibited a low affinity against hCA II but a nanomolar affinity against hCA IX. In terms of selectivity, complex 4b was our best candidate with promising hCA I/hCA IX and hCA II/hCA IX ratios of 261.75 and 30.64, respectively.
4. Conclusion
In short, two new bidentate 2-pyridyl-1,2,3-triazole ligands with a pendent 4-substituted benzenesulfonamide arm and their corresponding Re(CO)3-complexes were successfully synthesised. All the prepared compounds were fully characterised by classical spectroscopic methods and X-ray, as well as theoretical studies for both complexes. While in the solid state, rhenium complexes showed classical distorted octahedral geometry, ligand 3b adopted an uncommon cis-configuration arising from a network of intermolecular hydrogen-bonding interactions. DFT calculations revealed that the presence of methylene (−CH2−) linker between the benzenesulfonamide moiety and the chelate unit has a slight effect on the absorption bands position of 4b as well as the HOMO-LUMO gap which is slightly blue-shifted compared to 4a.
Preliminary investigation on the inhibitory activity of these compounds against the cytosolic human carbonic anhydrase I, II and the membrane-associated isoforms IX (hCAs I, II and IX) revealed an activity in the nanomolar range for hCA IX isozyme. Surprisingly, better inhibition was found with ligands compared to rhenium complexes. In particular, this preliminary study showed that ligand 3a exhibited a strong affinity Ki of 2.8 nM for hCA IX. Additionally, complex 4b exhibited a pronounced selectivity against hCA IX over the off-targets isoforms hCA I and hCA II which makes this compound a promising potential anticancer drug candidate. Further in vitro studies against other hCA isoforms with both ligands and rhenium complexes and other derivatives based on the same scaffold are under progress in order to rationalise these first biological results.
Supplemental Material
Download PDF (966.4 KB)Disclosure statement
No potential conflict of interest was reported by the authors.
Note
Additional information
Funding
Notes
1 Pyta and tapy mean 4-(2-pyridyl)-1,2,3-triazole and 1-(2-pyridyl)-1,2,3-triazole, respectively.
References
- Chen Y, Liu W, Jin JS. Rhenium(I) tricarbonyl complexes with bispyridine ligands attached to sulfur-rich core: syntheses, structures and properties. J Organomet Chem 2009;694:763–70.
- Chabolla SA, Machan CW, Yin J, et al. Bio-inspired CO2 reduction by a rhenium tricarbonyl bipyridine-based catalyst appended to amino acids and peptidic platforms: incorporating proton relays and hydrogen-bonding functional groups. Faraday Discuss 2017;198:279–300.
- (a) Chu WK, Ko CC, Chan KC, et al. A Simple design for strongly emissive sky-blue phosphorescent neutral rhenium complexes: synthesis, photophysics, and electroluminescent devices. Chem Mater 2014;26:2544–50. (b) Velmurugan G, Ramamoorthi BK, Venuvanalingam P. Are Re(I) phenanthroline complexes suitable candidates for OLEDs? Answers from DFT and TD-DFT investigations. Phys Chem Chem Phys 2014;16:21157–71. (c) Li X, Zhang D, Lu G, et al. Synthesis and characterization of novel rhenium (I) complexes with large Stokes shift for applications in organic electroluminescent devices. J Photochem Photobiol A Chem 2012;241:1–7.
- (a) Patrocinio AOT, Iha NYM. Photoswitches and luminescent rigidity sensors based on fac-[Re(CO)3(Me4phen)(L)]+. Inorg Chem 2008;47:10851–7. (b) Sun SS, Lees AJ. Transition metal based supramolecular systems: synthesis, photophysics, photochemistry and their potential applications as luminescent anion chemosensors. Coord Chem Rev 2002;230:171–92. (c) Bullock S, Hallett AJ, Harding LP, et al. Luminescent rhenium fac-tricarbonyl-containing complexes of androgenic oxo-steroids. Dalton Trans 2012;41:14690–6.
- (a) Pierri AE, Pallaoro A, Wu G, et al. A luminescent and biocompatible photoCORM. J Am Chem Soc 2012;134:18197–200. (b) Carrington SJ, Chakraborty I, Bernard JML, et al. A theranostic two-tone luminescent photocorm derived from Re(I) and (2-pyridyl)-benzothiazole: trackable CO delivery to malignant cells. Inorg Chem 2016;55:7852–8. (c) Prieto L, Rossier J, Derszniak K, et al. Modified biovectors for the tuneable activation of anti-platelet carbon monoxide release. Chem Commun 2017;53:6840–3. (d) Kianfar E, Schafer C, Lornejad-Schafer MR, et al. New photo-CORMs: deeply-coloured biocompatible rhenium complexes for the controlled release of carbon monoxide. Inorg Chim Acta 2015;435:174–7.
- (a) Müller C, Schubiger PA, Schibli R. Isostructural folate conjugates radiolabeled with the matched pair 99mTc/188Re: a potential strategy for diagnosis and therapy of folate receptor-positive tumors. Nucl Med Biol 2007;34:595–601. (b) Kluba CA, Mindt TL. Click-to-Chelate: development of technetium and rhenium-tricarbonyl labeled radiopharmaceuticals. Molecules 2013;18:3206–26. (c) Brink A, Helliwell JR. New leads for fragment-based design of rhenium/technetium radiopharmaceutical agents. IUCrJ 2017;4:283–90. (d)Eychenne R, Guizani S, Wang J-H, et al. Rhenium complexes based on an N2O tridentate click scaffold: from synthesis, structural and theoretical characterization to a radiolabelling study. Eur J Inorg Chem 2017; 2017:69–81.
- Schibli R, Schubiger PA. Current use and future potential of organometallic radiopharmaceuticals. Eur J Nucl Med 2002;29:1529–42.
- (a) Wright PJ, Muzzioli S, Werrett MV, et al. Synthesis, photophysical and electrochemical investigation of dinuclear tetrazolato-bridged rhenium complexes. Organometallics 2012;31:7566–78. (b) Werrett MV, Huff GS, Muzzioli S, et al. Methylated Re(I) tetrazolato complexes: photophysical properties and light emitting devices. Dalton Trans 2015;44:8379–93.
- (a) Coleman A, Brennan C, Vos JG, et al. Photophysical properties and applications of Re(I) and Re(I)–Ru(II) carbonyl polypyridyl complexes. Coord Chem Rev 2008;252:2585–95. (b) Lo KKW, Louie MW, Zhang KY. Design of luminescent iridium(III) and rhenium(I) polypyridine complexes as in vitro and in vivo ion, molecular and biological probes. Coord Chem Rev 2010;254:2603–22.
- (a) Boulay A, Seridi A, Zedde C, et al. Tricarbonyl Re(I) complexes from functionalised pyridine–triazole derivatives: from mononuclear to unexpected dimeric complexes. Eur J Inorg Chem 2010;2010:5058–62. (b) Wolff M, Munoz L, François A, et al. Tricarbonylrhenium complexes from 2-pyridyl-1,2,3-triazole ligands bearing a 4-substituted phenyl arm: a combined experimental and theoretical study. Dalton Trans 2013;42:7019–31. (c) François A, Auzanneau C, Le Morvan V, et al. A functionalized heterobimetallic 99mTc/Re complex as a potential dual-modality imaging probe: synthesis, photophysical properties, cytotoxicity and cellular imaging investigations. Dalton Trans 2014;43:439–50. (d) Clède S, Lambert F, Sandt C, et al. A rhenium tris-carbonyl derivative as a single core multimodal probe for imaging (SCoMPI) combining infrared and luminescent properties. Chem Commun 2012;48:7729–31. (e) Bertrand HC, Clède S, Guillot R, et al. Luminescence modulations of rhenium tricarbonyl complexes induced by structural variations. Inorg Chem 2014;53:6204–23. (f) Ching HYV, Wang X, He M, et al. Rhenium complexes based on 2-pyridyl-1,2,3-triazole ligands: a new class of CO2 reduction catalysts. Inorg Chem 2017;56:2966–76. (g) Kim TY, Elliott ABS, Shaffer KJ, et al. Rhenium(I) complexes of readily functionalized bidentate pyridyl-1,2,3-triazole “click” ligands: a systematic synthetic, spectroscopic and computational study. Polyhedron 2013;52:1391–8. (h) Lo WKC, Huff GS, Cubanski JR, et al. Comparison of inverse and regular 2-pyridyl-1,2,3-triazole “click” complexes: structures, stability, electrochemical, and photophysical properties. Inorg Chem 2015;54:1572–87.
- Obata M, Kitamura A, Mori A, et al. Syntheses, structural characterization and photophysical properties of 4-(2-pyridyl)-1,2,3-triazole rhenium(I) complexes. Dalton Trans 2008;3292–300.
- (a) Seridi A, Wolff M, Boulay A, et al. Rhenium(I) and technetium(I) complexes of a novel pyridyltriazole-based ligand containing an arylpiperazine pharmacophore: synthesis, crystal structures, computational studies and radiochemistry. Inorg Chem Commun 2011;14:238–42. (b) Kilpin KJ, Gavey EL, McAdam CJ, et al. Palladium(II) Complexes of readily functionalized bidentate 2-pyridyl-1,2,3-triazole “click” ligands: a synthetic, structural, spectroscopic, and computational study. Inorg Chem 2011;50:6334–46.
- (a) Güzel-Akdemir Ö, Akdemir A, Karal N, et al. Discovery of novel isatin-based sulfonamides with potent and selective inhibition of the tumor-associated carbonic anhydrase isoforms IX and XII. Org Biomol Chem 2015;13:6493–9. (b) Carta F, Ferraroni M, Scozzafava A, et al. Fluorescent sulfonamide carbonic anhydrase inhibitors incorporating 1,2,3-triazole moieties: kinetic and X-ray crystallographic studies. Bioorg Med Chem 2016;24:104–12. (c) Pala N, Micheletto L, Sechi M, et al. Carbonic anhydrase inhibition with benzenesulfonamides and tetrafluorobenzenesulfonamides obtained via click chemistry. ACS Med Chem Lett 2014;5:927–30. (d) Alterio V, Di Fiore A, D’Ambrosio K, et al. Multiple binding modes of inhibitors to carbonic anhydrases: how to design specific drugs targeting 15 different isoforms? Chem Rev 2012;112:4421–68. (e) Winum JY, Supuran CT. Recent advances in the discovery of zinc-binding motifs for the development of carbonic anhydrase inhibitors. J Enzyme Inhib Med Chem 2015;30:321–4.
- (a) Akurathi V, Dubois L, Lieuwes NG, et al. Synthesis and biological evaluation of a 99mTc-labelled sulfonamide conjugate for in vivo visualization of carbonic anhydrase IX expression in tumor hypoxia. Nucl Med Biol 2010;37:557–64. (b) Lu G, Hillier SM, Maresca KP, et al. Synthesis and SAR of novel Re/99mTc-labeled benzenesulfonamide carbonic anhydrase IX inhibitors for molecular imaging of tumor hypoxia. J Med Chem 2013;56:510–20. (c) Can D, Spingler B, Schmutz P, et al. (Cp-R)M(CO)3 (M = Re or 99mTc) Arylsulfonamide, arylsulfamide, and arylsulfamate conjugates for selective targeting of human carbonic anhydrase IX. Angew Chem Int Ed 2012;51:3354–7. (d) Nakai M, Pan J, Lin KS, et al. Evaluation of 99mTc-sulfonamide and sulfocoumarin derivatives for imaging carbonic anhydrase IX expression. Inorg Biochem 2018;185:63–70. (e) Huentupil Y, Peña L, Novoa N, et al. New sulfonamides containing organometallicacylhydrazones: synthesis, characterisation and biological evaluation as inhibitors of human carbonic anhydrases. J Enzyme Inhib Med Chem 2019;34:451–8.
- Chambers JM, Hill PA, Aaron JA, et al. Cryptophane Xenon-129 nuclear magnetic resonance biosensors targeting human carbonic anhydrase. J Am Chem Soc 2009;131:563–9.
- Sheldrick GM. Crystal structure refinement with SHELXL. Acta Crystallogr C Struct Chem 2015;71:3–8.
- Frisch MJ, Trucks GW, Schlegel HB, et al. Gaussian 09, revision A.1. Wallingford CT: Gaussian, Inc.; 2009.
- Dennington IIR, Keith T, Milliam J, GaussView version 4.1.2. Shawnee Mission, KS: Semichem Inc.; 2007.
- (a) Lee C, Yang W, Parr RG. Development of the Colle-Salvetti correlation-energy formula into a functional of the electron density. Phys Rev B 1988;37:785–9. (b) Becke AD. Density‐functional thermochemistry. III. The role of exact exchange. J Chem Phys 1993;98:5648–52.
- Hay PJ, Wadt WR. Ab initio effective core potentials for molecular calculations. Potentials for K to Au including the outermost core orbitals. J Chem Phys 1985;82:299–310.
- Glendening ED, Badenhoop JK, Reed AE, et al. NBO 6.0. Theoretical chemistry institute. Madison: University of Wisconsin; 2013.
- (a) Casida ME, Jamorski C, Casida KC, et al. Molecular excitation energies to high-lying bound states from time-dependent density-functional response theory: characterization and correction of the time-dependent local density approximation ionization threshold. J Chem Phys 1998;108:4439. (b) Stratmann RE, Scuseria GE, Frisch MJ. An efficient implementation of time-dependent density-functional theory for the calculation of excitation energies of large molecules. J Chem Phys 1998;109:8218. (c) Bauernschmitt R, Ahlrichs R. Treatment of electronic excitations within the adiabatic approximation of time dependent density functional theory. Chem Phys Lett 1996;256:454–64.
- (a) Cancès E, Mennucci B, Tomasi J. A new integral equation formalism for the polarizable continuum model: theoretical background and applications to isotropic and anisotropic dielectrics. J Chem Phys 1997;107:3032. (b) Cossi M, Barone V, Mennucci B, et al. Ab initio study of ionic solutions by a polarizable continuum dielectric model. Chem Phys Lett 1998;286:253–60. (c) Mennucci B, Tomasi J. Continuum solvation models: a new approach to the problem of solute’s charge distribution and cavity boundaries. J Chem Phys 1997;106:5151. (d) Cossi M, Scalmani G, Rega N, et al. New developments in the polarizable continuum model for quantum mechanical and classical calculations on molecules in solution. J Chem Phys 2002;117:43.
- Khalifah RG. The carbon dioxide hydration activity of carbonic anhydrase. I. Stop-flow kinetic studies on the native human isoenzymes B and C. J Biol Chem 1971;246:2561–73.
- Sharma A, Tiwari M, Supuran CT. Novel coumarins and benzocoumarins acting as isoform-selective inhibitors against the tumor-associated carbonic anhydrase IX. J Enzyme Inhib Med Chem 2014;29:292–6.
- Durdagi S, Scozzafava G, Vullo D, et al. Inhibition of mammalian carbonic anhydrases I-XIV with grayanotoxin III: solution and in silico studies. J Enzyme Inhib Med Chem 2014;29:469–75.
- Alterio V, Hilvo M, Di Fiore A, et al. Crystal structure of the catalytic domain of the tumor-associated human carbonic anhydrase IX. Proc Natl Acad Sci USA 2009;106:16233–8.
- La Regina G, Coluccia A, Famiglini V, et al. Discovery of 1,1'-biphenyl-4-sulfonamides as a new class of potent and selective carbonic anhydrase XIV inhibitors. J Med Chem 2015;58:8564–72.
- Suntrup L, Klenk S, Klein J, et al. Gauging donor/acceptor properties and redox stability of chelating click-derived triazoles and triazolylidenes: a case study with rhenium(I) complexes. Inorg Chem 2017;56:5771–83.
- (a) Schweinfurth D, Pattacini R, Strobel S, et al. New 1,2,3-triazole ligands through click reactions and their palladium and platinum complexes. Dalton Trans 2009;9291–7. (b) Crowley JD, Bandeen PH, Hanton LR. A one pot multi-component CuAAC “click” approach to bidentate and tridentate pyridyl-1,2,3-triazole ligands: synthesis, X-ray structures and copper(II) and silver(I) complexes. Polyhedron 2010;29:70–83. (c) Crowley JD, Bandeen PH. A multicomponent CuAAC “click” approach to a library of hybrid polydentate 2-pyridyl-1,2,3-triazole ligands: new building blocks for the generation of metallosupramolecular architectures. Dalton Trans 2010;39:612–23.
- Kilpin KJ, Crowley JD. Palladium(II) and platinum(II) complexes of bidentate 2-pyridyl-1,2,3-triazole “click” ligands: synthesis, properties and X-ray structures. Polyhedron 2010;29:3111–17.
- Scattergood PA, Sinopoli A, Elliott PIP. Photophysics and photochemistry of 1,2,3-triazole-based complexes. Coord Chem Rev 2017;350:136–54.