Abstract
There is significant interest in increasing the microalgal efficiency for producing high-quality products that are commonly used as food additives in nutraceuticals. Some natural substances that can be extracted from algae include lipids, carbohydrates, proteins, carotenoids, long-chain polyunsaturated fatty acids, and vitamins. Generally, microalgal photoautotrophic growth can be maximised by optimising CO2 biofixation, and by adding sodium bicarbonate and specific bacteria to the microalgal culture. Recently, to enhance CO2 biofixation, a thermostable carbonic anhydrase (SspCA) encoded by the genome of the bacterium Sulfurihydrogenibium yellowstonense has been heterologously expressed and immobilised on the surfaces of bacteria. Carbonic anhydrases (CAs, EC 4.2.1.1) are ubiquitous metalloenzymes, which catalyse the physiologically reversible reaction of carbon dioxide hydration to bicarbonate and protons: CO2 + H2O ⇄ HCO3− + H+. Herein, we demonstrate for the first time that the fragments of bacterial membranes containing immobilised SspCA (M-SspCA) on their surfaces can be doped into the microalgal culture of the green unicellular alga, Chlorella sorokiniana, to significantly enhance the biomass, photosynthetic activity, carotenoids production, and CA activity by this alga. These results are of biotechnological interest because C. sorokiniana is widely used in many different areas, including photosynthesis research, human pharmaceutical production, aquaculture-based food production, and wastewater treatment.
1. Introduction
Photosynthesis employs sunlight and the reaction between CO2 and H2O to generate carbohydrates and oxygen as a side product. This gas is necessary for the aerobic respiration but also promotes the formation of the ozone layer in the upper atmosphere. During the photosynthetic reactions, the energy of sunlight is converted into chemical energy, i.e., ATP and NADPH, which are thereafter involved in the biosynthesis of carbohydrates from CO2 as a unique carbon sourceCitation1,Citation2. The aerobic respiration (glucose + O2 → H2O + CO2), on the contrary, is the process of energy production, which converts sugars into carbon dioxide and water. These two opposite reactions influence the global carbon cycle, being fundamental for most life forms on earthCitation2. The light-dependent reactions to form glucose and other carbohydrates are known as the Calvin-Benson cycle. There are three photosynthetic pathways, C3, C4, and CAM (Crassulacean Acid Metabolism) that exist among terrestrial plantsCitation3,Citation4. In the C3 photosynthesis, which is the most ancestral form, the enzyme ribulose bisphosphate carboxylase-oxygenase (RuBisCO)Citation5, which is present in the chloroplast stroma of C3 plants, combines the ribulose-1,5-bisphosphate (RuBP), a molecule containing five carbon atoms, with CO2 to form two molecules of phosphoglycerate (PGA, a 3-carbon molecule)Citation6,Citation7. In the C4 pathway, the CO2 is converted into bicarbonate, which is subsequently reacted with phosphoenolpyruvic acid (PEP), a 3-carbon molecule, in the presence of phosphoenolpyruvate carboxylase (PEPC)Citation8. The product of this reaction is a 4-carbon molecule, oxaloacetic acid (OAA), which is thereafter reduced to malate, another four-carbon acidCitation8. The CAM pathway was documented for the first time in plant families that are adapted to very arid regions, such as many epiphytes and succulentsCitation9. These plants have a dual pathway of carboxylation temporally separated into the same tissue. In the night with the stomata opening, the CO2 is fixed as an organic acid form of the anion malate by PEPC. In contrast, during the day, with the stomatal closure, the malic acid undergoes decarboxylation, determining an increase of CO2 around the enzyme RuBisCO of about 60 times the ambient levels, allowing the photosynthetic reaction typical of the C3 cycle mentioned aboveCitation9. The RuBisCO enzyme also uses O2 as substrate, not only CO2Citation10. The rate of the oxygenation and carboxylation by RuBisCO is controlled by the levels of O2 and CO2 and is the primary factor in determining the efficiency of the photosynthetic processCitation11. CAM is one example of a carbon-concentrating mechanism (CCM) in higher order plants, in which, as mentioned above, decarboxylation of malic acids affords supplementary amounts of CO2.
Microalgal growth is driven by the same photosynthetic process present in higher plantsCitation12–15. Both freshwater and marine microalgae, also developed a CCM to increase CO2 concentration close to that for RuBisCO that is up to 1000-fold compared to the low CO2 concentrations found in aquatic environmentsCitation16. In the single-cell green alga, Chlamydomonas reinhardtii, the microalgal inorganic carbon uptake has been well describedCitation17,Citation18. It involves the diffusion of CO2 and transport of HCO3− across the microalgal membranes and the interconversion of CO2 and HCO3− by the algal carbonic anhydrases (CAs, EC 4.2.1.1), with the final result of concentrating the CO2 in the proximity of RuBisCOCitation17, which is localised mostly within the pyrenoids, the chloroplast microcompartments found in algaeCitation19–23. In cyanobacteria, carboxysomes are the equivalent of the pyrenoidsCitation24–27.
Indeed, CAs are a superfamily of metalloenzymes, which catalyse the simple but physiologically reversible and crucial reaction of carbon dioxide hydration to bicarbonate and protons: CO2 + H2O ⇄ HCO3− + H+Citation28–35. To date, CAs are categorised into eight genetically distinct families (or classes), named with the Greek letters: α, β, γ, δ, ζ, η, θ, and ιCitation36. The last three classes were only recently discoveredCitation37–41. The distribution of CA-classes is very variegated in most living organisms investigated so far. CAs present in animals belong to α-classCitation21,Citation42, plants and algae have α, β, γ, δ, ζ, θ and ι-classes; fungi encode for α and β-CAs; protozoa for α, β and/or η-CAs; bacteria for α, β, γ, and, as recently reported, for ι-CA classesCitation34,Citation37,Citation40,Citation43–47. The proposed physiological role of CAs in all these organisms is to regulate pH and to assist the transport of carbon dioxide and bicarbonate, making possible their balance inside the cells, which will not be ensured by the very low kcat (0.15 s−1) of the uncatalyzed CO2 hydration/dehydration reactionCitation43,Citation48–52. All these roles of CAs have in the end crucial physiological functions for the metabolism of the organisms in which they are foundCitation43,Citation48–52.
Recently, considerable and diverse efforts have been made to improve the efficiency of microalgal cultures, as they provide biomass abundant in high-value products, such as lipids, carbohydrates, and proteinsCitation53,Citation54. Moreover, they are also a biological factory of carotenoids, long-chain polyunsaturated fatty acids, and vitamins, which are commonly used as food additives in nutraceuticalsCitation53,Citation55,Citation56. Generally, for maximising the microalgal biomass during the photoautotrophic growth, the microalgal cultures are usually optimised improving the CO2-fixation or, by adding sodium bicarbonateCitation57,Citation58 or specific bacteriaCitation59. Recently, our groups heterologously expressed and immobilised on the surface of bacterial hosts a thermostable α-CA (SspCA is the acronym) from the bacterium Sulfurihydrogenibium yellowstonenseCitation60. This approach, entitled in vivo immobilisation, was achieved by transforming the E. coli cells with a plasmid containing a chimeric gene resulted by the fusion of a signal peptide (pelB gene), which directs the neosynthesized protein to the bacterial periplasmic space; the gene (INPN gene) encoding for the Pseudomonas syringae INP domain, which anchors the neosynthesized protein to the bacterial outer membrane (external side); and the gene encoding for the thermostable enzyme SspCACitation60. The anchored SspCA was thus efficiently overexpressed on the external bacterial surface of E. coli and was stable and active for 15 h at 70 °C and for many days at 25 °CCitation60. Assuming that the CA activity facilitates the rapid conversion of the aqueous CO2 to HCO3−, we hypothesised that the addition of an exogenous and thermostable CA into the microalgal culture might enhance the algal bicarbonate uptake ameliorating the microalgal growth. Thus, in the present paper, this concept was investigated for the first time and used to enhance the biomass, photosynthetic activity, carotenoids production, and CA activity of Chlorella sorokiniana. This was achieved by supplementing the microalgal culture with fragments of bacterial membranes containing the immobilised SspCA on their surface and biocarbonate. Furthermore, the results were compared with those obtained by adding only sodium bicarbonate at the concentration of 1.0 g/L.
2. Materials and methods
2.1. Chemicals and instruments
All the chemicals used in this study were of reagent grade and purchased from Sigma and GE Healthcare. SDS–PAGE apparatus was procured by BioRAD.
2.2. Protein determination
The protein quantification was carried out by Bradford method (BioRAD)Citation61.
2.3. Preparation of the bacterial membrane with the immobilised SspCA
Competent E. coli BL21 (DE3) cells were transformed with construct indicated with the acronym pET-22b/INPN-SspCA and prepared as describe by Del Prete et al.Citation60. Bacterial cells were grown at 37 °C, and when cells reached an OD600 of 0.6–0.7, the protein surface expression was induced with 0.5 mM isopropyl-thio-b-D-galactoside (IPTG) and 0.5 mM ZnSO4. After additional growth for 6 h, the cells were harvested by centrifugation and washed three times with PBS. Aliquots of cells were resuspended in 25 mM Tris-HCl, pH 8.0. Membrane fragments containing the immobilised SspCA (M-SspCA) were prepared disrupting the cells by sonication (10 s, for 10 cycles). 0.5 g of M-SspCA were added at time 0 and 48 h to the algal medium containing the bicarbonate.
2.4. Assay for carbonic anhydrase using CO2 as substrate
CA activity assay was performed as described by Capasso et al.Citation62. Briefly, the assay was based on the monitoring of pH variation due to the catalysed conversion of CO2 to bicarbonate. Bromothymol blue was used as the indicator of pH variation and the assay was performed at 0 °C. The CO2-satured solution was used as substrate. To test the activity of carbonic anhydrase, 1.0 mL of 25 mM Tris, pH 8.3, containing bromothymol blue as a dye (to give a distinct and visible blue colour) was added to two test tubes chilled in an ice bath. An appropriate amount of the enzyme solution (e.g. microalgal cell extract) were added to one tube, and an equivalent amount of buffer was added to the second tube as control. One millilitre of CO2 solution was added, and the time required for the solution to change from blue to yellow was recorded (transition point of bromothymol blue is pH 6.0–7.6). The time required for the colour change is inversely related to the quantity of enzyme present in the sample. Wilbur-Anderson units were calculated according to the following definition: One Wilbur-Anderson unit (WAU) of activity is defined as (T0−T)/T, where T0 (uncatalyzed reaction) and T (catalysed reaction) are recorded as the time (in seconds) required for the pH to drop from 8.3 to the transition point of the dye in a control buffer and in the presence of enzyme, respectively.
2.5. Protonography
To prepare Chlorella crude extract for protonography, aliquots of 200 mL of algal culture were harvested by centrifugation at 4000 g for 7 min; the pellets were re-suspended in 4.0 mL of cold extraction buffer (50 mM Tris-HCl, pH 8.3) and the cells were lysed by two passage at 1100 psi through French pressure cell (Aminco). The lysate was cleared by centrifugation at 12,000 g for 30 min at 4 °C and the obtained supernatant represented the crude extract. To perform the protonography, wells of 12% SDS-PAGE gel prepared as described by LaemmliCitation63, were loaded with an appropriate amount of the microalgal crude extract mixed with loading buffer without 2-mercaptoethanol and without boiling the samples, in order to avoid protein denaturation. The gel was run at 150 V until the dye front ran off the gel. Following the electrophoresis, the 12% SDS-PAGE gel was treated as described by Capasso et al.Citation64–67 to detect the yellows bands due to the hydratase activity.
2.6. Algal strains and growth conditions
Chlorella sorokiniana Shihira and Krauss, strain 211/8k (CCAP of Cambridge University) was grown in Erlenmeyer flask at 30 ± 2.5 °C, under continuously light (led panel, 70 µmol photons m−2 s−1) and flushed with air. The composition of the basal medium was previously reported by Salbitani et al.Citation68, and the pH of the medium was 7.5 at T0. Cultures of C. sorokiniana were grown with a supplementation of 0 (control) and 1.0 g L−1 of NaHCO3. The bicarbonate was added, in a single administration at T0, when cultures were into lag phase (∼2.0 × 106 cell mL−1). To some cultures supplemented with bicarbonate 1.0 g L−1, at T0 or T2 fragments of bacterial membranes (0.5 g) with the immobilised thermostable SspCA were added. The number and cells size of Chlorella were determined by Countess II FL Automated Cell Counter (Thermo Fisher Scientific) equipped with a fluorescence filter (Ex 628/40, Em 692/40; EVOS Light Cube for Cy5; Termo Fisher Scientifc Inc.).
2.7. Pigment contents
Total chlorophyll and carotenoids contents were estimated spectrophotometrically after extraction into N,N-dimethylformamide according to Inskeep and BloomCitation69 and WellburnCitation70, respectively.
2.8. Photosynthetic efficiency
The maximum PSII photochemical efficiency (Fv/Fm) has been determined using a Phyto_PAM II compact unit (Walz). All samples were acclimated at the dark for 30 min before the analysis to minimise the non-photochemical dissipation of excitation, and measurements were blank corrected filtering the sample through 0.2 μm filterCitation71. As regard Fv/Fm, samples were illuminated with a saturating pulse following as reported in Maxwell and JonsonCitation72, and values derived from the formula Fv/Fm = (Fm−F0)/Fm.
3. Results and discussion
3.1. Basal hydratase activity of the endogenous microalgal CAs
The analysis of the genome belonging to different microalgal species evidences a very variegated pattern of CA classes. It is possible to identify seven of the eight CA-classes discovered up to now in these organisms. The different classes can coexist or have different localizations inside the cells, such as the cell wall, plasma membrane, cytosol, mitochondria, chloroplast stroma, and chloroplast thylakoid lumenCitation73–75. Besides, for each enzyme class, many isoforms were reported to existCitation74. In the present manuscript, the interest was focussed on the freshwater green microalga Chlorella sorokiniana as it can be useful in many fields, such as photosynthesis research, pharmaceuticals for humans, aquaculture foods, and wastewater treatment. In 1998, a soluble form of CA belonging to the α-classCitation76 was purified and characterised from C. sorokiniana. Other CA-classes appear to be encoded by this green microalga genome although they have not yet been characterisedCitation76.
The C. sorokiniana extract was subjected to the Wilbur-Anderson (WA) assay and protonography, to investigate the microalgal endogenous CA hydrates activity. Using CO2 as a substrate, the CA specific activities of the microalgal extract resulted to be 20 ± 0.7 WAU/mg. The protonography analysis, which is specific for the detection of the CO2 hydratase activity on the polyacrylamide gel, was performed treating the SDS-PAGE gel with blue bromothymol, which is blue in its deprotonated form. The production of H+ ions, due to the CA hydratase activity, lowers the pH of the solution to pH 6.8, the colour transition point of the dye, developing a yellow band in correspondence of the hydratase activity. As a positive control, the commercial bovine α-CA (bCA) has been used. shows the protonogram obtained using the C. sorokiniana crude extract. Intriguing, the protonogram evidenced three yellow bands corresponding to the CO2 hydratase activity (). One band is at the gel position corresponding to 29 kDa, the molecular weight of the C. sorokiniana α-CA, whereas the other two bands (very close to each other) are visible at a molecular weight above 50 kDa. The latter two bands could be a different oligomeric state of the α-CA (not wholly dissociated in the monomer form with MW of about 29 kDa) or a different class of the microalgal CAs not yet characterised (). It is interesting to note that, these microalgal CAs, such as the CAs identified in other speciesCitation64, can refold and generate their active form correctly after the removal of the SDS by the gel to accomplish the protonography analysis.
Figure 1. Microalgal endogenous activity revealed by the protonography analysis. Legend: Lane 1, molecular markers; Lane 2, C. sorokiniana cellular extract; Lane 3, commercial bovine CA used as positive controls. The arrows identified the yellow bands corresponding to CO2 hydratase activity due to the microalgal CAs.
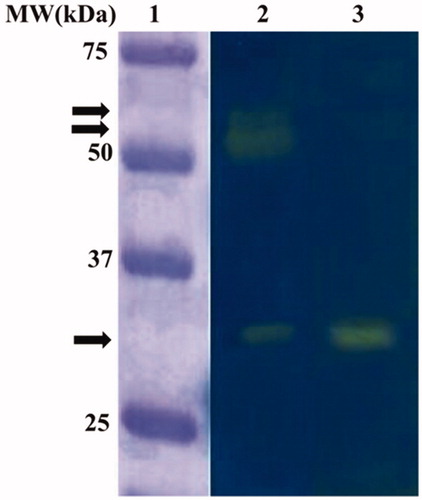
3.2. Microalgal growth
C. sorokiniana cells were cultivated under four different conditions: (1) Control: culture growth in the basal medium; (2) Bic: culture supplemented at T0 (0 h) with bicarbonate at 1.0 g L−1; (3) M-SspCA-0: culture supplemented at T0 with bicarbonate (1.0 g L−1) and fragments of bacterial membranes (0.5 g) with the immobilised thermostable SspCA; (4) M-SspCA-2: culture supplemented at T0 with bicarbonate (1.0 g L−1) and at T2 (48 h) with fragments of bacterial membranes (0.5 g) having the immobilised thermostable SspCA.
indicates the growth of C. sorokiniana under the four different conditions (specified above). The growth was monitored by determining the number of microalgal cells and the optical density (OD800 nm) of the cultures. The algae biomass growth based on the optical density was not shown because it displayed a profile very similar to that of the number of cells mL−1. From is readily apparent that when the microalgal medium was supplemented with bicarbonate or bicarbonate and M-SspCA at the two growing times (0 and 48 h), there was an increase, already at 24 h, in the number of cells with respect to the control (). Interestingly, after 48 h, bicarbonate supplemented medium provoked an increase of the number of cells (9.97 × 106 ± 0.015 cell mL−1) that was similar to that for M-SspCA-2, and 1.5 times higher compared to the number of cells (6.85 × 106 ± 0.212 cell mL−1) obtained using bicarbonate plus M-SspCA added at the initial time. Obviously, up to 48 h the Bic and M-SspCA-0 cultures proceed in a completely similar manner. Intriguing, the addition of M-SspCA at 48 h (M-SspCA-2), resulted in better microalgal growth, generating, at 72 h, an evident increase in the number of the cells, which was of about 2.2 times (16.86 × 106 ± 1.95 cell mL−1) than the control (7.8 × 106 ± 0.26 cell mL−1), 1.7 time than M-SspCA-0 (9.7 × 106 ± 0.5 cell mL−1) and 1.3 times than Bic (12.75 × 106 ± 0.14 cell mL−1). We can speculate that the addition of the M-SspCA at the initial time transforms the bicarbonate in CO2, reducing the availability of bicarbonate and the uptake from the microalgal cells. On the contrary, the addition of M-SspCA at 48 h increased the number of cells mL−1. These can be explained considering that during the first 48 h, the microalgal growth in M-SspCA-2 cultures is supported only by bicarbonate; in fact, in absence of exogenous carbon dioxide, in M-SspCA-2 cultures, the bicarbonate is only in a very small part spontaneously converted in CO2. In addition, the bicarbonate is consumed by the algal growth and there is an accumulation of CO2; the addition of M-SspCA at 48 h immediately converts the CO2, from air and cellular metabolism, into bicarbonate, giving a further boost to microalgal growth. Thus, the bicarbonate coming from the catalysed reaction of the thermostable SspCA ameliorate the microalgal growth, as demonstrated by the fact that the number of cells is increased up to 17 × 106 cell mL−1 (see ). This is corroborated by the fact that, as described in the literature, microalgal growth is improved by the addition of bicarbonate, reducing the microalgal oxidative stress induced by the macronutrient deficient conditionsCitation77,Citation78.
Figure 2. Cellular density (cells mL−1) in control and experimental cultures (Bic, M-SspCA-0, M-SspCA-2) of Chlorella sorokiniana. Error bars represent SD (n = 3).
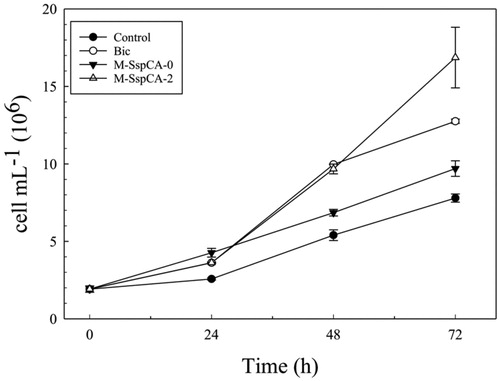
Another exciting aspect is the difference in the C. sorokiniana cell size when the alga is grown in the four different conditions aforementioned. shows the microalgal cell size monitored at different times. As a result, at 24 h the cell size increased in the cultures supplemented with bicarbonate or bicarbonate plus the M-SspCA (added at 0 or 48 h) (). It is fascinating to note that the addition of M-SspCA at 0 and 48 h determines at 72 h an increase of about 1.7 times of the microalgal cell size compared to the control. On the contrary, the medium supplemented with bicarbonate alone determined at 24 h an increase of the microalgal cell size of 1.3 times respect to the control, which slightly started to decrease after the 24 h (). The increase of average cell diameter could be related to intracellular carbon storage; in fact, according to previous studies, bicarbonate supplementation to microalgae cultures promotes lipid accumulationCitation79,Citation80.
3.3. Microalgal photosynthetic efficiency (Fv/Fm)
shows the photosynthetic efficiency (Fv/Fm) of C. sorokiniana in the cultures supplemented with bicarbonate or bicarbonate containing M-SspCA. An increase of Fv/Fm was observed growing C. sorokiniana in the presence of bicarbonate and M-SspCA. The microalgal photosynthesis efficiency reached its maximum value at 72 h in the cultures supplemented with bicarbonate plus the M-SspCA at time 0 and 48 h compared to the control. In contrast, the photosynthetic behaviour of the cells grown in the presence of only bicarbonate showed a maximum of Fv/Fm at 24 h (about 0.42 ± 0.03) and, after that time, started to decrease at 0.32 ± 0.02 Fv/Fm. It can be hypothesised that the reduction of Fv/Fm after 24 h is due to the low availability of bicarbonate in the medium caused by microalgal utilisation. This is supported by the fact that the presence of M-SspCA guarantees bicarbonate in the microalgal culture medium through the reaction catalysed by the thermostable CA, which converts CO2 of the medium into bicarbonate. Moreover, as shown in , the addition of M-SspCA at 48 h determined an increment of the microalgal photosynthetic activity.
Figure 4. Maximum quantum yield (Fv/Fm) in Chlorella sorokiniana control and experimental cultures (Bic, M-SspCA-0, M-SspCA-2). Error bars represent SD (n = 3).
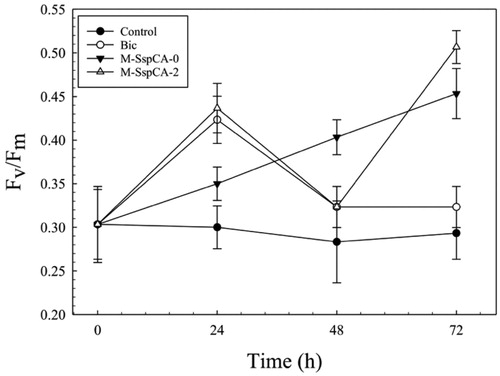
Furthermore, since the shortage of bicarbonate in the culture determines a condition of stress for the microalgal culture, slowing down the photosynthetic carbon fixation, the total content of the chlorophylls had been also monitored. As expected, considering the data previously shown, the photosynthetic pigment had a maximum in the microalgal culture grown in the medium containing bicarbonate and M-SspCA (). In fact, the addition of M-SspCA, with its hydratase activity and using the CO2 present in the medium solution, regenerates the bicarbonate, which is necessary for increasing the microalgal metabolism and, thus, the photosynthetic pigment. The addition of M-SspCA at T0 is only responsible of an increase of the total chlorophyll contents at 24 h ().
3.4. Carotenoids production
Microalgae are considered to be one of the best commercial sources of natural carotenoids, such as β-carotene, astaxanthin, canthaxanthin, lutein, etc. These molecules are produced in a variable amount depending on microalgal growth conditionsCitation69. Besides, carotenoids are of great interest to humans since they are used as antioxidants, anti-inflammatories, antidiabetics, anti-obesities, antitumoral, and it has been reported that they possess a cardiovascular and neuronal protective roleCitation69. For this reason, we explored the carotenoids production by C. sorokiniana in the medium supplemented with bicarbonate and bicarbonate with M-SspCA. evidences the content of the total carotenoids in the Chlorella cells. The carotenoids content remained almost constant up to 24 h in the four conditions. At 48 h, the microalgal carotenoid production was slightly enhanced by the presence of bicarbonate or bicarbonate with M-SspCA, however, at 72 h, its amount in M-SspCA-2 became three times higher (4.8 mg L−1 ± 0.09) compared to the control (1.62 mg L−1 ± 0.17). These results are fascinating for a biotechnological application because the membrane-bound SspCA can be used in the microalgal culture for obtaining a very high production of the carotenoids.
3.5. Determination of the microalgal endogenous hydratase activity at 72 h
As described above, the basal specific activities of the microalgal endogenous CAs resulted to be of 20 ± 0.7 WAU/mg. Since the CA is a crucial enzyme in the carbon concentration mechanism by enhancing the conversion between CO2 and bicarbonate ions, the microalgal CA activity of the microalgal cells coming from the control medium has been measured at 72 h, as well as in the medium supplemented with bicarbonate and the culture medium containing bicarbonate and M-SspCA added at the two different times (0 and 48 h). The cells were extensively washed and recovered by centrifugation. To avoid the interference of the external M-SspCA added to the culture medium, a control containing M-SspCA-0 or M-SspCA-2 without microalgal cells was prepared. As a result, the CA specific activity at 72 h of the microalgal culture was of 60 ± 0.8 WAU/mg when the M-SspCA was added at 48 h to the medium culture supplemented with bicarbonate. At the same time, it resulted to be 40 ± 0.7 WAU/mg when added at time 0 and in the medium containing only bicarbonate. The addition of M-SspCA at 48 h to the microalgal medium drastically influences the metabolic efficiency of the microalgal culture, determining an increase of the photosynthetic efficiency, microalgal cell size, total chlorophyll, as well as a higher production of carotenoids (see ). It can be speculated that the extra-CA activity provided by SspCA may enhance the conversion of bicarbonate to CO2 in the proximity of the RuBisCO enzyme affecting thus the metabolic efficiency of C. sorokiniana.
4. Conclusions
In the present paper, fragments of the bacterial membranes containing a thermostable SspCA (M-SspCA) immobilised on their surface were used for exploring the effect on growth and photosynthetic efficiency of the freshwater green microalga Chlorella sorokiniana. M-SspCA was added to the microalgal culture medium supplemented with bicarbonate at two different times (0 and 48 h). The microalgal metabolic efficiency was investigated following the variation in the number of cells, photosynthetic activity, carotenoids production, and CA activity. The results presented in the present paper evidenced that the maximum algal growth was reached when M-SspCA is added to the culture at 48 h. The addition of M-SspCA at T0 mainly affects the microalgal growth and the photosynthetic efficiency at 48 h. In conclusion, it can be speculated that when the bicarbonate is consumed by the algal uptake and there is an accumulation of CO2 into the microalgal medium, the addition of M-SspCA at T2, immediately converts the CO2 into bicarbonate. Mainly, its effect is to increase the microalgal metabolic efficiency significantly with respect to the control medium or the medium supplemented only with bicarbonate. This is of a great interest from a biotechnological viewpoint because the freshwater green microalga Chlorella sorokiniana can be useful in many fields, such as photosynthesis research, human pharmaceutical production, aquaculture foods, and wastewater treatment as well as third-generation biofuels feedstockCitation81.
Acknowledgements
We are grateful to Giovanni Del Monaco for technical assistance.
Disclosure statement
No potential conflict of interest was reported by the author(s).
Additional information
Funding
References
- Miner GL, Bauerle WL, Baldocchi DD. Estimating the sensitivity of stomatal conductance to photosynthesis: a review. Plant Cell Environ 2017;40:1214–38.
- Niyogi KK. Editorial overview: physiology and metabolism: light responses from photoreceptors to photosynthesis and photoprotection. Curr Opin Plant Biol 2017;37:iv–vi.
- Kumpers BM, Burgess SJ, Reyna-Llorens I, et al. Shared characteristics underpinning C4 leaf maturation derived from analysis of multiple C3 and C4 species of Flaveria. J Exp Bot 2017;68:177–89.
- Reeves G, Grange-Guermente MJ, Hibberd JM. Regulatory gateways for cell-specific gene expression in C4 leaves with Kranz anatomy. J Exp Bot 2017;68:107–16.
- Tcherkez G, Gauthier P, Buckley TN, et al. Leaf day respiration: low CO2 flux but high significance for metabolism and carbon balance. New Phytol 2017;216:986–1001.
- Galmes J, Andralojc PJ, Kapralov MV, et al. Environmentally driven evolution of Rubisco and improved photosynthesis and growth within the C3 genus Limonium (Plumbaginaceae). New Phytol 2014;203:989–99.
- Sage TL, Busch FA, Johnson DC, et al. Initial events during the evolution of C4 photosynthesis in C3 species of Flaveria. Plant Physiol 2013;163:1266–76.
- Niklaus M, Kelly S. The molecular evolution of C4 photosynthesis: opportunities for understanding and improving the world’s most productive plants. J Exp Bot 2019;70:795–804.
- Yamori W, Hikosaka K, Way DA. Temperature response of photosynthesis in C3, C4, and CAM plants: temperature acclimation and temperature adaptation. Photosyn Res 2014;119:101–17.
- Ślesak I, Ślesak H, Kruk J. RubisCO early oxygenase activity: a kinetic and evolutionary perspective. Bioessays 2017;39:1700071.
- Allakhverdiev SI, Kreslavski VD, Klimov VV, et al. Heat stress: an overview of molecular responses in photosynthesis. Photosyn Res 2008;98:541–50.
- Giordano M, Beardall J, Raven JA. CO2 concentrating mechanisms in algae: mechanisms, environmental modulation, and evolution. Annu Rev Plant Biol 2005;56:99–131.
- Kaplan A, Reinhold L. CO2 concentrating mechanisms in photosynthetic microorganisms. Annu Rev Plant Physiol Plant Mol Biol 1999;50:539–70.
- Moroney JV, Jungnick N, Dimario RJ, Longstreth DJ. Photorespiration and carbon concentrating mechanisms: two adaptations to high O2, low CO2 conditions. Photosyn Res 2013;117:121–31.
- Raven JA, Beardall J, Sanchez-Baracaldo P. The possible evolution and future of CO2-concentrating mechanisms. J Exp Bot 2017;68:3701–16.
- Atkinson N, Feike D, Mackinder LC, et al. Introducing an algal carbon-concentrating mechanism into higher plants: location and incorporation of key components. Plant Biotechnol J 2016;14:1302–15.
- Mackinder L. The Chlamydomonas CO2-concentrating mechanism and its potential for engineering photosynthesis in plants. New Phytol 2018;217:54–61.
- Wang Y, Duanmu D, Spalding MH. Carbon dioxide concentrating mechanism in Chlamydomonas reinhardtii: inorganic carbon transport and CO2 recapture. Photosyn Res 2011;109:115–22.
- Alterio V, Langella E, Viparelli F, et al. Structural and inhibition insights into carbonic anhydrase CDCA1 from the marine diatom Thalassiosira weissflogii. Biochimie 2012;94:1232–41.
- De Simone G, Supuran CT. (In)organic anions as carbonic anhydrase inhibitors. J Inorg Biochem 2012;111:117–29.
- Supuran CT. Carbonic anhydrases as drug targets-an overview. Curr Top Med Chem 2007;7:825–33.
- Supuran CT. Carbonic anhydrases-an overview. Curr Pharm Des 2008;14:603–14.
- Supuran CT, Scozzafava A. Carbonic anhydrases as targets for medicinal chemistry. Bioorg Med Chem 2007;15:4336–50.
- Kinney JN, Axen SD, Kerfeld CA. Comparative analysis of carboxysome shell proteins. Photosyn Res 2011;109:21–32.
- Turmo A, Gonzalez-Esquer CR, Kerfeld CA. Carboxysomes: metabolic modules for CO2 fixation. FEMS Microbiol Lett 2017;364: 364–70.
- Kimber MS. Carboxysomal carbonic anhydrases. Subcell Biochem 2014;75:89–103.
- Price GD, Pengelly JJ, Forster B, et al. The cyanobacterial CCM as a source of genes for improving photosynthetic CO2 fixation in crop species. J Exp Bot 2013;64:753–68.
- Del Prete S, De Luca V, De Simone G, et al. Cloning, expression and purification of the complete domain of the eta-carbonic anhydrase from Plasmodium falciparum. J Enzyme Inhib Med Chem 2016;31:54–9.
- Del Prete S, Vullo D, De Luca V, et al. Cloning, expression, purification and sulfonamide inhibition profile of the complete domain of the η-carbonic anhydrase from Plasmodium falciparum. Bioorg Med Chem Lett 2016;26:4184–90.
- Del Prete S, Vullo D, De Luca V, et al. Anion inhibition profiles of the complete domain of the η-carbonic anhydrase from Plasmodium falciparum. Bioorg Med Chem 2016;24:4410–4.
- Annunziato G, Angeli A, D'Alba F, et al. Discovery of new potential anti-infective compounds based on carbonic anhydrase inhibitors by rational target-focused repurposing approaches. ChemMedChem 2016;11:1904–14.
- Del Prete S, Vullo D, De Luca V, et al. Anion inhibition profiles of α-, β- and γ-carbonic anhydrases from the pathogenic bacterium Vibrio cholerae. Bioorg Med Chem 2016;24:3413–7.
- Abdel Gawad NM, Amin NH, Elsaadi MT, et al. Synthesis of 4-(thiazol-2-ylamino)-benzenesulfonamides with carbonic anhydrase I, II and IX inhibitory activity and cytotoxic effects against breast cancer cell lines. Bioorg Med Chem 2016;24:3043–51.
- Capasso C, Supuran CT. An overview of the carbonic anhydrases from two pathogens of the oral cavity: Streptococcus mutans and Porphyromonas gingivalis. Curr Top Med Chem 2016;16:2359–68.
- Del Prete S, Vullo D, De Luca V, et al. Comparison of the sulfonamide inhibition profiles of the α-, β- and γ-carbonic anhydrases from the pathogenic bacterium Vibrio cholerae. Bioorg Med Chem Lett 2016;26:1941–6.
- Supuran CT, Capasso C. An overview of the bacterial carbonic anhydrases. Metabolites 2017;7:56.
- Jensen EL, Clement R, Kosta A, et al. A new widespread subclass of carbonic anhydrase in marine phytoplankton. Isme J 2019;13:2094–106.
- Kikutani S, Nakajima K, Nagasato C, et al. Thylakoid luminal θ-carbonic anhydrase critical for growth and photosynthesis in the marine diatom Phaeodactylum tricornutum. Proc Natl Acad Sci USA 2016;113:9828–33.
- De Simone G, Di Fiore A, Capasso C, Supuran CT. The zinc coordination pattern in the η-carbonic anhydrase from Plasmodium falciparum is different from all other carbonic anhydrase genetic families. Bioorg Med Chem Lett 2015;25:1385–9.
- Supuran CT, Capasso C. The η-class carbonic anhydrases as drug targets for antimalarial agents. Expert Opin Ther Targets 2015;19:551–63.
- Del Prete S, Vullo D, Fisher GM, et al. Discovery of a new family of carbonic anhydrases in the malaria pathogen Plasmodium falciparum-the η-carbonic anhydrases. Bioorg Med Chem Lett 2014;24:4389–96.
- Aspatwar A, Tolvanen ME, Ortutay C, Parkkila S. Carbonic anhydrase related proteins: molecular biology and evolution. Subcell Biochem 2014;75:135–56.
- Capasso C, Supuran CT. Bacterial, fungal and protozoan carbonic anhydrases as drug targets. Expert Opin Ther Targets 2015;19:1689–704.
- Capasso C, Supuran CT. An overview of the selectivity and efficiency of the bacterial carbonic anhydrase inhibitors. Curr Med Chem 2015;22:2130–9.
- Capasso C, Supuran CT. An overview of the alpha-, beta- and gamma-carbonic anhydrases from Bacteria: can bacterial carbonic anhydrases shed new light on evolution of bacteria? J Enzyme Inhib Med Chem 2015;30:325–32.
- Capasso C, Supuran CT. Sulfa and trimethoprim-like drugs - antimetabolites acting as carbonic anhydrase, dihydropteroate synthase and dihydrofolate reductase inhibitors. J Enzyme Inhib Med Chem 2014;29:379–87.
- Capasso C, Supuran CT. Anti-infective carbonic anhydrase inhibitors: a patent and literature review. Expert Opin Ther Patents 2013;23:693–704.
- Prete SD, Angeli A, Ghobril C, et al. Anion inhibition profile of the beta-carbonic anhydrase from the opportunist pathogenic Fungus Malassezia restricta involved in dandruff and seborrheic dermatitis. Metabolites 2019;9: 147–57.
- Del Prete S, Vullo D, Ghobril C, et al. Cloning, purification, and characterization of a beta-carbonic anhydrase from Malassezia restricta, an opportunistic pathogen involved in dandruff and seborrheic dermatitis. Int J Mol Sci 2019;20: 2447–59.
- Nocentini A, Bua S, Del Prete S, et al. Natural polyphenols selectively inhibit β-carbonic anhydrase from the dandruff-producing fungus Malassezia globosa: activity and modeling studies. ChemMedChem 2018;13:816–23.
- Nocentini A, Vullo D, Del Prete S, et al. Inhibition of the β-carbonic anhydrase from the dandruff-producing fungus Malassezia globosa with monothiocarbamates. J Enzyme Inhib Med Chem 2017;32:1064–70.
- Entezari Heravi Y, Bua S, Nocentini A, et al. Inhibition of Malassezia globosa carbonic anhydrase with phenols. Bioorg Med Chem 2017;25:2577–82.
- Minhas AK, Hodgson P, Barrow CJ, Adholeya A. A review on the assessment of stress conditions for simultaneous production of microalgal lipids and carotenoids. Front Microbiol 2016;7: 546–64.
- Carfagna S, Landi V, Coraggio F, et al. Different characteristics of C-phycocyanin (C-PC) in two strains of the extremophilic Galdieria phlegrea. Algal Res 2018;31:406–12.
- Bottone C, Camerlingo R, Miceli R, et al. Antioxidant and anti-proliferative properties of extracts from heterotrophic cultures of Galdieria sulphuraria. Nat Prod Res 2018;15:1–5.
- Carfagna S, Salbitani G, Bottone C, Vona V. Galdieria sulphuraria as a possible source of food colorant. J Nutr Ecol Food Res 2016;3:78–81.
- Mus F, Toussaint JP, Cooksey KE, et al. Physiological and molecular analysis of carbon source supplementation and pH stress-induced lipid accumulation in the marine diatom Phaeodactylum tricornutum. Appl Microbiol Biotechnol 2013;97:3625–42.
- YuvrajPadmanabhan P. Technical insight on the requirements for CO2-saturated growth of microalgae in photobioreactors. 3 Biotech 2017;7:119.
- Fuentes JL, Garbayo I, Cuaresma M, et al. Impact of microalgae-bacteria interactions on the production of algal biomass and associated compounds. Mar Drugs 2016;14: 100–16.
- Del Prete S, Perfetto R, Rossi M, et al. A one-step procedure for immobilising the thermostable carbonic anhydrase (SspCA) on the surface membrane of Escherichia coli. J Enzyme Inhib Med Chem 2017;32:1120–8.
- Bradford MM. A rapid and sensitive method for the quantitation of microgram quantities of protein utilizing the principle of protein-dye binding. Anal Biochem 1976;72:248–54.
- Capasso C, De Luca V, Carginale V, et al. Biochemical properties of a novel and highly thermostable bacterial α-carbonic anhydrase from Sulfurihydrogenibium yellowstonense YO3AOP1. J Enzyme Inhib Med Chem 2012;27:892–7.
- Laemmli UK. Cleavage of structural proteins during the assembly of the head of bacteriophage T4. Nature 1970;227:680–5.
- De Luca V, Del Prete S, Supuran CT, Capasso C. Protonography, a new technique for the analysis of carbonic anhydrase activity. J Enzyme Inhib Med Chem 2015;30:277–82.
- Del Prete S, De Luca V, Iandolo E, et al. Protonography, a powerful tool for analyzing the activity and the oligomeric state of the γ-carbonic anhydrase identified in the genome of Porphyromonas gingivalis. Bioorg Med Chem 2015;23:3747–50.
- Del Prete S, De Luca V, Supuran CT, Capasso C. Protonography, a technique applicable for the analysis of η-carbonic anhydrase activity . J Enzyme Inhib Med Chem 2015;30:920–4.
- Del Prete S, Vullo D, Caminiti-Segonds N, et al. Protonography and anion inhibition profile of the α-carbonic anhydrase (CruCA4) identified in the Mediterranean red coral Corallium rubrum. Bioorg Chem 2018;76:281–7.
- Salbitani G, Wirtz M, Hell R, Carfagna S. Affinity purification of O-acetylserine(thiol)lyase from Chlorella sorokiniana by recombinant proteins from Arabidopsis thaliana. Metabolites 2014;4:629–39.
- Inskeep WP, Bloom PR. Extinction coefficients of chlorophyll a and B in n,n-dimethylformamide and 80% acetone. Plant Physiol 1985;77:483–5.
- Wellburn AR. The spectral determination of chlorophylls a and b, as well as total carotenoids, using various solvents with spectrophotometers of different resolution. J Plant Physiol 1994;144:307–13.
- Cullen JJ, Davis RF. The blank can make a big difference in oceanographic measurements. Limnol Oceanogr Bull 2003;12:29–35.
- Maxwell K, Johnson GN. Chlorophyll fluorescence-a practical guide. J Exp Bot 2000;51:659–68.
- Moroney JV, Ma Y, Frey WD, et al. The carbonic anhydrase isoforms of Chlamydomonas reinhardtii: intracellular location, expression, and physiological roles. Photosyn Res 2011;109:133–49.
- DiMario RJ, Machingura MC, Waldrop GL, Moroney JV. The many types of carbonic anhydrases in photosynthetic organisms. Plant Sci 2018;268:11–7.
- Samukawa M, Shen C, Hopkinson BM, Matsuda Y. Localization of putative carbonic anhydrases in the marine diatom, Thalassiosira pseudonana. Photosyn. Res 2014;121:235–49.
- Satoh A, Iwasaki T, Odani S, Shiraiwa Y. Purification, characterization and cDNA cloning of soluble carbonic anhydrase from Chlorella sorokiniana grown under ordinary air. Planta 1998;206:657–65.
- Srinivasan R, Mageswari A, Subramanian P, et al. Bicarbonate supplementation enhances growth and biochemical composition of Dunaliella salina V-101 by reducing oxidative stress induced during macronutrient deficit conditions. Sci Rep 2018;8:6972.
- Salbitani G, Barone CMA, Carfagna S. Effect of bicarbonate on growth of the oleaginous microalga Botryococcus braunii. Int J Plant Biol 2019;10: 35–7.
- Li J, Li C, Lan CQ, Liao D. Effects of sodium bicarbonate on cell growth, lipid accumulation, and morphology of Chlorella vulgaris. Microb Cell Fact 2018;17:111
- Lohman EJ, Gardner RD, Pedersen T, et al. Optimized inorganic carbon regime for enhanced growth and lipid accumulation in Chlorella vulgaris. Biotechnol Biofuels 2015;8:82
- Chiong MC, Meng CC, Cheng TC, et al. Liquid biofuels production and emissions performance in gas turbines: a review. Energy Conversion Manage 2018;173:640–58.