ABSTRACT
The tumor microenvironment (TME) plays an essential role in tumor cell survival by profoundly influencing their proliferation, metastasis, immune evasion, and resistance to treatment. Extracellular vesicles (EVs) are small particles released by all cell types and often reflect the state of their parental cells and modulate other cells’ functions through the various cargo they transport. Tumor-derived small EVs (TDSEVs) can transport specific proteins, nucleic acids and lipids tailored to propagate tumor signals and establish a favorable TME. Thus, the TME’s biological characteristics can affect TDSEV heterogeneity, and this interplay can amplify tumor growth, dissemination, and resistance to therapy. This review discusses the interplay between TME and TDSEVs based on their biological characteristics and summarizes strategies for targeting cancer cells. Additionally, it reviews the current issues and challenges in this field to offer fresh insights into comprehending tumor development mechanisms and exploring innovative clinical applications.
1. Introduction
The tumor microenvironment (TME) comprises a complex interplay of physiological and biochemical elements, including tumor cells, tumor-associated fibroblasts, innate and adaptive immune cells, the vascular system, cytokines, and the extracellular matrix (ECM) networkCitation1 (). Changes within the TME significantly influence tumor growth, invasion, metastasis and the effectiveness of therapeutic interventions and often arise due to factors such as primary organ location, tumor characteristics, stage and patient status, which can then lead to consequential impact on drug resistance and treatment outcomes.Citation2 Recent studies have also confirmed the interaction between tumor cells and tumor-associated immune cells in TME, reporting the effects of tumor progression and metastasis through different signal transduction or receptor activation pathways.Citation3 The TME can be characterized by hypoxia,Citation4 acidity,Citation5 nutrient deprivationCitation6 and immunosuppressionCitation7 due to the rapid tumor growth, increased swelling, and inadequate vascularization. These unique features provide a favorable microenvironment for tumor development and dissemination.
Figure 1. Schematic representation illustrating the components and interactions within the tumor microenvironment.
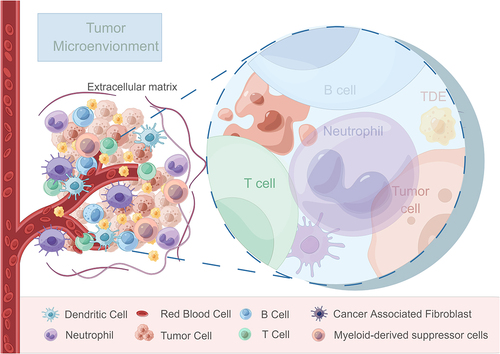
Extracellular vesicles (EVs) are membrane-bound structures present in various bodily fluids, including blood, urine, and ascites. Among these, small extracellular vesicles (sEVs), typically measuring less than 200 nm in diameter, fulfill diverse physiological and pathological functions.Citation8
Tumor-derived small extracellular vesicles (TDSEVs) are continuously released by tumor cells and serve as specialized signaling carriers within theTME.Citation9 As an important form of communication between tumor cells and non-tumor cells in the microenvironment, TDSEVs play essential roles in various stages of tumor development.Citation10 Recent findings indicate that TDSEVs harbor a diverse array of molecular constituents, including lipids, membrane-associated proteins, long noncoding RNAs (lncRNAs), and miRNAs,Citation10 which contribute to key processes in tumor initiation and progression, such as angiogenesis, proliferation, metastasis, and immune evasion.Citation11 Moreover, the prominent biological features of TME, such as hypoxia, extracellular acidosis and low nutrients, can induce heterogeneous changes in TDSEVs release, cargo composition and transport, which are also key determinants for malignancy, proliferation, and metastasis.Citation9,Citation12,Citation13 Therefore, it is imperative not to overlook the role of TDSEVs in maintaining the survival environment of tumor cells and promoting their invasion and metastasis. Investigating the mechanisms by which TDSEVs mediate tumor occurrence and development through their interaction with the TME could offer valuable guidance for devising more effective tumor prognosis and treatment strategies based on TDSEVs and TME interactions.
This review examines the interaction between TMEs and TDSEVs, with a primary focus on the biological characteristics of TMEs. We focus on the reciprocal regulatory mechanisms of TDSEVs within tumor hypoxic, acidic, nutrient-deprived and immunosuppressive microenvironments and discuss targeted therapeutic approaches involving TDSEVs or TMEs, along with the predominant challenges currently faced in this field. Additionally, we provide a comprehensive review of the potential of targeted strategies directed toward TMEs and TDSEVs in tumor therapy to provide insights into potential clinical treatment methods and present innovative concepts for combating tumors.
2. Generation and characteristics of EVs
2.1. EVs and exosomes
EVs are membranous particles released from cells, bounded by lipid bilayers, and lacking a functional nucleus, thus incapable of self-replication.Citation8 Exosomes are a subtype of EVs secreted by cells. They can be broadly categorized into ectosomes and exosomes.Citation14 Exosomes typically range from 30 to 150 nm (with a mean of 100 nm) in size and are formed via the endocytic pathway through several sequential steps.Citation14 Initially, plasma membrane invagination leads to the formation of early endosomes.Citation15,Citation16 Subsequently, intracellular multivesicular bodies (MVBs) arise through inward budding, generating intraluminal vesicles (ILVs) enveloped within endosomes.Citation16 Ultimately, ILVs fuse with the cell membrane, facilitating exosome releaseCitation16 (). The size of MVBs ranges between 100 and 250 nm, and they harbor multiple ILVs, which themselves vary in diameter from 30 to 150 nm.Citation17 Various proteins participate in ILV and MVB formation, as well as cargo selection. Notably, endosomal sorting complexes required for transport (ESCRT) proteins, such as ESCRT-0, -I, -II and -III,Citation16,Citation18 are important in exosome biogenesis and possess distinct roles in membrane shaping and cargo sorting, wherein ESCRT-0 and ESCRT-I facilitate the association of ubiquitinated cargo with lipid microdomains, while ESCRT-II and -III mediate invagination and MVB/ILV formation.Citation9 Additionally, an ESCRT-independent pathway exists for exosome biogenesis.Citation19
Figure 2. Schematic representation depicting the process of exosome biogenesis and release. A. The steps involved in exosome biogenesis: ① Invagination of the plasma membrane. ② Formation of early endosomes. ③ Maturation of early endosomes into late endosomes, which develop into multivesicular bodies (MVBs). The membrane invagination of MVBs results in the formation of intraluminal vesicles (ILVs), which then fuse with the plasma membrane and release ILVs as exosomes. B. Schematic representation illustrating the structure of exosomes.
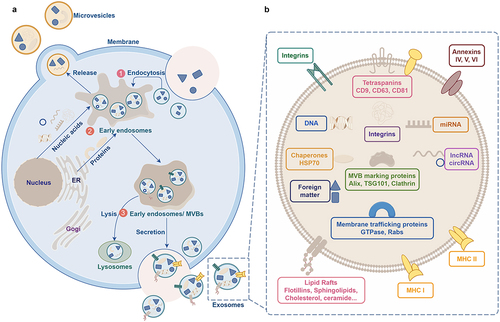
Ectosomes are vesicles that bud off from the plasma membrane via outward budding and encompass microvesicles, microparticles, and larger vesicles ranging from 50 to 1000 nm in diameter.Citation14 Despite the considerable knowledge regarding exosomes, our understanding on the biosynthesis of ectosomes remains largely unclear. The process of ectosome formation requires the accumulation of cargo on specific microdomains of the cytosolic surface of the plasma membrane. Concurrent membrane dynamics involve outward budding and fission within these microdomains. It is believed that the rearrangement of asymmetric membrane phospholipid layers, mediated by Ca2+-dependent enzymes, flippases and floppases may contribute to this process.Citation20 Notably, the activation of at least two ESCRT complexes is similar to the mechanism observed during intraluminal vesicle (ILV) formation.Citation21 Other mechanisms that regulate ectosome shedding from the plasma membrane include Arf6, a small GTPase implicated in vesicular transport, and members of the Rho family of GTPases, such as RhoA, Cdc42, and Rac1, which facilitate cortical actin contraction beneath the plasma membrane.Citation22–25 Furthermore, ectosome production typically occurs across a large, extensive portion of the plasma membrane in response to various stimuli. Similar to exosomes, ectosomes also exhibit high levels of cholesterol, sphingomyelin, and ceramides.Citation26
However, there is currently no consensus on specific biomarkers for EV subtypes, making it challenging to categorize EVs into distinct biogenesis pathways. Consequently, much of the existing literature on “exosomes” and “ectosomes/microvesicles” addresses heterogeneous populations of EVs rather than those released via specific biogenesis routes.Citation8 To address this ambiguity, the International Society for Extracellular Vesicles (ISEV) emphasizes careful characterization, often relying on particle diameter to define EV subtypes.Citation8 Specifically, sEVs are typically described as <200 nm in diameter, while large EVs exceed 200 nm in diameter.Citation8 Exosomes constitute a subset of sEVs, with endosomes typically containing intracellular vesicles smaller than 200 nm in diameter. Ectosomes can vary in size, including those resembling exosomes. The sEV population encompasses both exosomes and small ectosomes. Henceforth, this review will consistently use sEVs to denote the term “exosome” in the literature.
2.2. TDSEVs
TDSEVs are sEVs released by tumor cells and are commonly detected in tumor tissue as well as in the body fluids of cancer patients.Citation27,Citation28 The biogenesis process of TDSEVs is similar to that of normal cells, yet tumor cells employ various strategies to modulate sEV biogenesis mechanisms, thereby facilitating tumor progression.Citation29 Notably, tumor cells produce and secrete significantly greater amounts of sEVs compared to normal proliferating cells, with elevated sEV levels frequently observed in the plasma and other bodily fluids of cancer patients.Citation30–35 Factors such as the hypoxic conditions prevalent in the TME have been reported to contribute to this robust sEV secretion by tumor cells.Citation36 Furthermore, certain enzymes or proteins play roles in regulating sEV production from tumor cells. For instance, the p53 protein, often aberrantly activated in cancer, is implicated in regulating sEV production and release by tumor cells.Citation37 Heparanase, overexpressed in many tumor cell lines, also regulates sEV secretion.Citation38 Moreover, Rap GTPase proteins, particularly Rab27a and Rab27b, which regulate secretory pathways, are strongly associated with sEV release.Citation39,Citation40 The knockdown of Rab proteins has been shown to reduce sEV secretion from tumor cells.Citation40,Citation41 Despite emerging insights into the regulation of sEV secretion by tumor cells, the precise mechanisms remain unclear. In the TME, TDSEVs are essential for intercellular communication, transferring messages from the parent tumor cell to recipient cells and modulating autocrine, juxtacrine, and paracrine signaling pathways essential for cancer cell survival.Citation42 Importantly, the paracrine activity of TDSEVs extends beyond the tumor site, as they can circulate and convey information to distant tissues and cells.Citation43 Recent studies have demonstrated that TDSEVs can promote the activation, expansion, and immunosuppressive function of immune cells.Citation44 They also participate in tumor progression and immune regulation and mediate intercellular communication within the TME.
3. Interplay between TMEs and TDSEVs
3.1. Hypoxic TME
A hypoxic TME is associated with increased intercellular communication, characterized by greater frequency and complexity of signal transduction. Emerging evidence indicates that sEVs, functioning as signaling transporters, are involved in the intricate process of hypoxia-induced signaling.Citation45,Citation46
3.1.1. Affection of TDSEVs secretion and heterogeneity
Firstly, there is a significant increase in sEV release from the hypoxic microenvironment. Cancer cells respond to hypoxia by producing more sEVs, thus fulfilling the communication requirements of cancer cells compared to the normoxic state. This phenomenon has been observed in diverse solid tumor types, including breast cancer,Citation36,Citation47 colorectal cancer (CRC),Citation48,Citation49 glioma,Citation50 gastric cancer,Citation51 hepatocellular carcinoma (HCC),Citation52 and pancreatic cancer.Citation53 However, Ramteke et al. found that prostate cancer cells (LNCaP) secreted a comparable amount of sEVs under normoxic and hypoxic conditions (0.26E8 vs. 0.24E8 particles/mL).Citation54 Additionally, hypoxia has been found to increase the number of sEVs in non-tumor cells, indicating the widespread occurrence of hypoxia-induced sEV release.Citation55–57 Currently, it is widely accepted that hypoxia affects the release of sEVs through three main mechanisms. First, hypoxia affects key phases in sEV release, including cargo sorting, plasma membrane fusion,Citation58 and MVB transport.Citation58,Citation59 The hypoxic TME impacts the biogenesis and release of TDSEVs, as illustrated in . Second, hypoxia triggers certain releases by involving cofactors such as actin cytoskeletons,Citation60 hypoxia regulators,Citation56 microtubules,Citation61 and molecular motors.Citation62 Third, hypoxia’s involvement in the degradation process of MVBs leads to the release of intraluminal vesicles (ILVs) as exosomes.Citation63 However, the specific effects of hypoxia and interactions with these key molecules remain largely unexplored.
Figure 3. Schematic diagram illustrating key steps in the biogenesis and transport of tumor-derived exosomes in a hypoxic tumor microenvironment. ① Hypoxia influences cargo synthesis and sorting within exosomes. ② Hypoxia affects cargo loading into exosomes. ③ Hypoxia regulates exosome biogenesis by influencing the degradation of transporters (such as TSG101). ④ the hypoxic environment regulates exosome release by influencing the degradation of Rab GTPases (such as Rab27a). ⑤ the hypoxic environment regulates exosome release by affecting SNARE complexes specifically required for multivesicular body (MVB) fusion with the plasma membrane. ⑥ the hypoxic microenvironment influences the transport of tumor-derived exosomes to recipient cells. ⑦ the hypoxic microenvironment affects the recognition and uptake of tumor-derived exosomes by recipient cells.
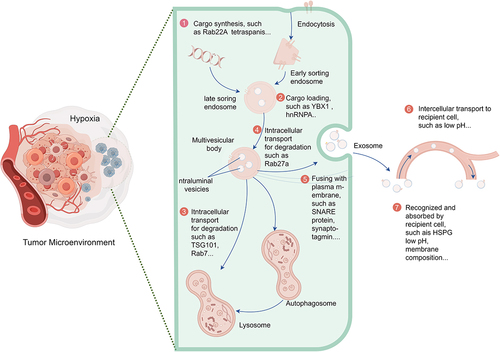
Notably, hyperoxia has been observed to diminish the release of sEVs from CRC cells compared to hypoxia.Citation48 Moreover, hypoxia can augment the heterogeneity of TDSEVs, which can be characterized by variations in size, cargo composition, and functional effects on recipient cells.Citation14 Empirical evidence in the literature suggests that hypoxia induces the release of smaller EVs, as demonstrated in studies on various tumor cell lines, including CRC,Citation49,Citation64 pancreatic cancer,Citation53 prostate cancer,Citation54 and bone marrow mesenchymal stem cells.Citation65 Additionally, hypoxia influences the cargo-sorting mechanism of TDSEVs.Citation66–69 Similarly, the composition of EV cargo, encompassing glycoconjugates, lipids, nucleic acids, and proteins, exhibits variability among sEVs derived from different cancer types. While the specific effects of hypoxia on different cancer cells may vary or remain unclear, hypoxia profoundly impacts the quantity, composition, and status of EV cargo. This influence extends to cargo loading in cancer cells through intricate sorting mechanisms. Investigating the precise mechanisms underlying EV cargo loading in hypoxic cancer cells promises significant advancements in developing sEV-based interventions tailored for the hypoxic TME, thereby enhancing cancer therapy.
3.1.2. Promoting TME remodeling
Hypoxia-induced TDSEVs possess the potential to promote tumor angiogenesis and metastasis. Recent studies have validated that hypoxic HCC-derived sEVs containing miR-1273f and miR-23a/b enhance the invasive phenotype of cancer cells, contributing to HCC progression.Citation70,Citation71 Additionally, Xue et al. demonstrated that sEVs derived from hypoxic bladder cancer (BCa) cells exhibit a greater capacity to stimulate BCa proliferation compared to those from normal BCa cells.Citation72 Moreover, TDSEVs induced by hypoxia influence cancer immune evasion. Accumulating evidence suggests that hypoxia-induced TDSEVs can induce T cell apoptosis, suppress natural killer (NK) cell activity, inhibit type II macrophage expression dependent on IFN-γ, alter monocyte differentiation, and increase the population of myeloid-derived suppressor cells (MSDCs).Citation72–74 Consequently, these immune response alterations lead to diminished immune surveillance and facilitate tumor evasion from immune recognition.
In summary, TDSEVs in hypoxic TME exhibit multifaceted functions and variations, emphasizing the importance of further exploration in this area to accurately delineate the TME landscape. Advancements in single-cell transcriptomic and spatial transcriptomic strategies could provide both challenges and opportunities for investigating different temporal, spatial, and subpopulations of sEVs within the hypoxic TME.
3.2. Acidic TME
The TME is characterized by altered tumor cell metabolism, resulting in an acidic pH, which stems from abnormal cell-cell interactions and disrupted homeostasis. In this metabolic state, tumor cells primarily rely on glycolysis over oxidative phosphorylation for energy production – a phenomenon known as anaerobic glycolysis. Consequently, there is a significant increase in lactate levels in the extracellular environment, accompanied by the diffusion of H+ ions into the cell stroma, ultimately leading to acidification of the extracellular pH within the TME.Citation75
3.2.1. Affecting the properties of TDSEVs
An acidic TME promotes the secretion of sEVs and facilitates their subsequent uptake and fusion with cells. Research has shown a significant increase in the levels of proteins, nucleic acids, and surface biomarkers in tumor-derived sEVs within an acidic environment, while these markers were not detected in an alkaline environment.Citation76 In vitro studies have demonstrated a notable increase in sEV secretion when the pH is shifted from 7.4 to the characteristic acidic pH of 6.5 found in cancer cells, highlighting the important significance of tumor acidity in promoting heightened sEV release in human cancer cell lines.Citation12,Citation77,Citation78 Parolini et al. reported that sEV release is enhanced under low pH conditions, accompanied by an increase in the content of sphingomyelin/ganglioside GM3 within sEVs, thereby promoting their uptake and fusion with recipient cells.Citation77 Recent investigations have revealed that cells experiencing similar conditions within the TME (i.e., low pH and hypoxia) show a notable increase in the uptake of tumor-derived sEVs by parental cells, particularly under low pH treatment.Citation79 The self-aggregation of glycerolipids between tumor cells and their sEVs has been identified as the underlying cause of the increased homologous uptake of TDSEVs.Citation79 Moreover, Hisey et al. quantified sEV release from ovarian cancer cells using nanoparticle tracking analysis (NTA) combined with nanoscale flow cytometry (NFC) and provided experimental evidence for the essential role of TME pH in regulating sEV production and release.Citation80 Therefore, alkalinization of the TME may hold promise as a strategy for the treatment of various cancer diseases.
3.2.2. Promoting tumor progression
The release of TDSEVs in response to an acidic TME contributes significantly to invasion and metastasis. In an acidic TME, TDSEVs facilitate communication between cancer cells and stromal cells, thereby driving the formation of pre-metastatic niches (PMNs) and promoting metastasis and resulting in the acquisition of metastatic properties within the primary tumor or at distant sites.Citation41 Tian et al. demonstrated that an acidic TME upregulated the expression levels of miR-21 and miR-10b in HCC-derived sEVs,Citation81 which promoted the migration and invasion of recipient HCC cells cultured under normal conditions, both in vitro and in vivo.Citation81 Furthermore, the presence of an acidic TME and the associated up-regulation of miR-21 and miR-10b in sEVs have been correlated with poor prognosis for HCC, highlighting the essential role of acidic TME-induced TDSEVs in invasion and metastasis.Citation81
Furthermore, TDSEVs regulate tumor angiogenesis through multiple pathways and targets by transporting and delivering various tumor angiogenic factors within the acidic TME. He et al. discovered that sEV miR-205 secreted by ovarian cancer cells induces tumor angiogenesis by modulating the PTEN-AKT signaling pathway.Citation82 Similarly, CRC-derived sEV miR-25-3p is transferred from cancer cells to endothelial cells (ECs), where it targets KLF2 and KLF4 and regulates the expression of VEGFR2, ZO-1, and Claudin5 proteins, thereby enhancing vascular permeability and promoting tumor angiogenesis.Citation83 Additionally, TDSEVs carry angiogenic molecules such as miR-221-3p, miR-1246, miR-3157, and miR-210-3p, which activate signaling pathways in ECs to boost tumor vascular density and promote angiogenesis in various cancers, including cervical squamous cell carcinoma,Citation84 non-small cell carcinoma,Citation85 and oral cancer.Citation86
In summary, experimental evidence confirms that an acidic TME enhances sEV release from cancer cells and results in the accumulation of sEVs within the tumor, which subsequently disseminate through the bloodstream, thereby promoting tumor growth and metastasis. Using this understanding for diagnostic and therapeutic purposes could represent a groundbreaking advancement in cancer treatment.
3.3. Immunosuppressive TME (iTME)
In contrast to other hypoxic, acidic, and nutrient-deprived microenvironments, there has been limited investigation into the regulatory mechanisms governing the release and cargo sorting of TDSEVs within the iTME. The impact of TDSEVs on immune cells within the TME is dualistic, as they can either stimulate or inhibit immune responses.Citation87,Citation88 TDSEVs can facilitate antigen presentation and thereby activate the immune system.Citation87 Conversely, TDSEVs can also induce immunosuppression by delivering ligands, proteins, and miRNAs that inhibit immune cell activity.Citation89 Presently, it is known that TDSEVs can regulate the TME by activating immune cells,Citation90 promoting the differentiation of tumor-associated macrophages (TAMs),Citation91 enhancing the inhibitory effect of TAMs,Citation91 and inhibiting immune cell function, such as T cells,Citation92 MDSCs,Citation73 and NK cellsCitation42 ().
Figure 4. Regulation of immune cells in an immunosuppressive microenvironment by tumor-derived small extracellular vesicles (TDSEVs). ① TDSEVs activate immune cells and promote antitumor immunity by carrying tumor-associated antigens (TAA) in their cargo. ② TDSEVs play a crucial role in the conversion of M0 macrophages to M2 macrophages, commonly referred to as tumor-associated macrophages (TAMs). ③ TDSEVs enhance the inhibitory effect of myeloid-derived suppressor cells (MDSCs). ④ it has been observed that TDSEVs can inhibit the function of immune cells.
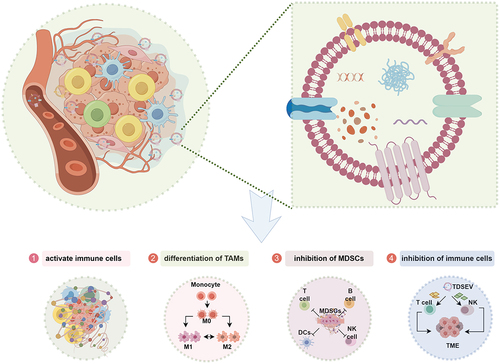
3.3.1. Activating immune cells
TDSEVs can activate immune cells and promote antitumor immunity by carrying tumor-associated antigens (TAAs) in their cargo.Citation93 The presence of these antigens, along with major histocompatibility complex (MHC) molecules, suggests the potential development of TDSEVs as anticancer vaccines.Citation87 Moreover, TDSEVs can deliver heat shock protein 70 (HSP70) and MHC class I molecules to dendritic cells (DCs), thereby inducing the activation of CD8+ T cells with the potential to exert an anticancer effect.Citation90,Citation94 Additionally, TDSEVs can create a localized inflammatory environment that enhances immune responses, making them a potent adjuvant in anticancer therapy.Citation95 However, the immune-stimulating ability of TDSEVs may not always be sufficient to impede tumor progression, possibly due to the dual opposing roles of these vesicles within the TME.
3.3.2. Promoting TAM differentiation
TAMs are polarized M2 macrophages that play essential roles in regulating the iTME.Citation96 Studies have confirmed the important roles of TDSEVs and their cargos in mediating the communication between tumor cells and TAMs, thereby reprogramming the host immune response.Citation97 Specifically, sEVs derived from breast cancer (BRCA) have been shown to induce M2 polarization of macrophages by down-regulating PTEN and activating the AKT/STAT3/6 signaling pathway, thereby promoting BRCA progression.Citation98 Similarly, the polarization of M2 macrophages and the secretion of sEV LINC00273, facilitated by tumor-derived sEV miR-19b-3p, contribute to the metastasis of lung adenocarcinoma via the Hippo pathway.Citation99 Moreover, the induction of M2 polarization in macrophages by tumor-derived sEV miR-934 promotes liver metastasis in CRC.Citation100 Despite significant advancements in understanding the mechanisms by which TDSEVs promote TAM differentiation, the communication network between TDSEVs, tumor cells, and TAMs remains incompletely understood.Citation101 Therefore, further elucidating the mechanisms of information transfer between TDSEVs, TAMs, and tumor cells, as well as their mechanisms of action, could facilitate the development of novel therapeutic strategies and their clinical applications.
3.3.3. Enhancing the immunosuppression of MDSC
MSDCs are a subset of neutrophils and monocytes that are pathologically activated with immunosuppressive activity, serving as negative regulators of immunity. Currently, TDSEVs have been shown to play a crucial role in the immunosuppression of MDSCs. Over a decade ago, Xiang et al. demonstrated that TDSEVs can promote MDSCs to produce inhibitory molecules and enhance their inhibitory activity in tumor models.Citation102 Subsequently, TDSEV proteins and nucleic acids have been identified as involved in regulating MDSC expansion and immunosuppression. For instance, mammary carcinoma-derived sEVs containing abundant prostaglandin E2 (PGE2) and transforming growth factor-beta (TGF-β) enhance the expansion and immunosuppression of MDSCs via the MyD88 pathway by increasing the production of interleukin-6 (IL-6) and vascular endothelial growth factor (VEGF).Citation103 Glioma-derived sEV miR-1246 promotes MDSC differentiation and activation.Citation104 Moreover, under hypoxic conditions, glioma cells secrete sEVs containing high levels of miR-29a and miR-92a, which can be transferred to MDSCs, thereby enhancing MDSC differentiation and function.Citation105 A recent study by Shokati et al. discussed the mechanism by which MDSC-derived sEV miRNAs and tumor-derived sEV miRNAs can regulate antitumor immunity by modulating the interaction between tumor cells and MDSCs in the TME.Citation106 Collectively, these studies highlight the importance of TDSEVs in MDSC cell biology, gradually revealing the regulatory mechanisms of TDSEVs on MDSCs and providing significant insights for the development of specific targetable therapeutic strategies for eliminating MDSC-induced immunosuppression.
3.3.4. Inhibiting T cell function
Emerging evidence suggests that TDSEVs are major contributors to inducing T cell dysfunction. TDSEVs can suppress T cell antitumor immunity through various mechanisms, including inhibiting T cell proliferation and response, promoting regulatory T cell (Treg) expansion, and inducing T cell apoptosis and exhaustion.Citation107 For instance, sEV TGF-β derived from breast cancer cell lines has been demonstrated to suppress T cell proliferation.Citation108 Interestingly, TDSEVs from breast cancer cell lines are significantly enhanced by the hypoxic TME, potentially contributing to breast cancer therapy resistance.Citation109 Moreover, pancreatic cancer-derived sEVs upregulate numerous genes associated with apoptosis and endoplasmic reticulum (ER) stress in T cells, and the uptake of these TDSEVs by T lymphocytes can trigger p38 MAP kinase signaling, leading to ER stress-induced apoptosis in T cells.Citation110 In addition to their direct effects on T cells, TDSEVs also induce T cell inhibition by affecting DCs and MSDCs. For example, prostate cancer-derived sEVs impair DC antigen presentation, promoting adenosine-mediated inhibition of CD8+ T cell activation.Citation111 Furthermore, melanoma-derived sEVs and TME accessory cells impair DC function, leading to the expansion of Tregs and MDSCs and limiting T cell cytotoxicity.Citation112 While TDSEVs have been reported to affect T cell exhaustion,Citation107 it remains largely unknown whether they also induce other states of T cell anergy, stemness, and senescence. Therefore, a deeper understanding of these molecular regulations will be crucial for the development of effective therapeutic strategies for cancer treatment.
3.3.5. Inhibiting NK cell function
NK cells serve as the frontline defense against malignant cell transformation. However, tumor cells can undermine NK cell function through various mechanisms, with TDSEVs playing a pivotal role in inducing NK cell dysfunction. Multiple studies have indicated that TDSEVs can deliver their cargo to NK cells via cell membrane fusion, thus hindering their antitumor activity.Citation113 TDSEVs employ six main strategies to inhibit NK cell function. Firstly, the uptake or interaction of TDSEVs with NK cells is believed to contribute to immune suppression and tumor evasion. For example, sEVs from pancreatic cancer cells (L3.6pl) and murine mammary carcinoma cells (TS/A) are internalized by NK cells and remain stably present in the cytoplasm, leading to decreased cytotoxic activity.Citation114,Citation115 Secondly, TDSEVs regulate NK cell migration and recruitment. Hong et al. demonstrated that sEVs isolated from patients with acute myeloid leukemia significantly reduced the migration of NK-92 cells toward tumor cells.Citation116 Thirdly, TDSEVs influence NK cell proliferation and survival. According to Hong et al., sEVs derived from gastric cancer reduce the proliferation of NK-92 cells and decrease the frequency of CD8+ T and NK cells.Citation116 By investigating the impact of TDSEVs on NK cell proliferation and survival, Liu et al. observed that pre-treatment with sEVs derived from murine breast carcinoma decreased the number and percentage of NK cells in vitro.Citation115 Furthermore, TDSEVs modulate the cytolytic activity of NK cells. For instance, NK cells exposed to sEVs from pancreatic cancer display reduced cytotoxicity against pancreatic cancer stem cells.Citation114 Although co-incubation of NK cells with oral cancer-derived sEVs initially enhances their killing effect on oral cancer cells, prolonged exposure leads to a significant decrease in cytotoxicity, suggesting that while TDSEVs may stimulate NK cell cytotoxicity in the short term, long-term exposure inhibits their cytolytic function, promoting immune evasion and cancer progression.Citation117 Additionally, TDSEVs regulate cytokine production by NK cells. Cholangiocarcinoma-derived sEVs were found to significantly reduce the release of TNF-α by NK cells, impairing their ability to combat tumors.Citation118 Similarly, NK cells exposed to sEVs from pancreatic cancer exhibited a notable decrease in TNF-1 and IFN-γ secretion.Citation114 Moreover, TDSEVs alter the expression patterns of receptors and molecules in NK cells, potentially contributing to tumor-associated NK cell dysfunction. Since TDSEVs reflect parental cell contents, they likely play a role in modulating receptor and molecular expression, further influencing NK cell function.Citation119 Recent research indicates that oral cancer-derived sEVs initially increase the expression of activating receptors on NK cells for 24 hours, but this effect diminishes over 7 days.Citation117 Overall, studying the effects of TDSEVs on NK cells underscores the role of tumor-derived sEVs in immunosuppression. However, numerous other unknown or understudied TDSEV molecules contribute to NK cell dysfunction in tumors.Citation120 Further research is needed to elucidate how TDSEVs affect NK cell function and improve cancer treatment.
3.4. Nutrient-deprived TME
The Warburg effect, a hallmark of tumor energy metabolism, is intricately regulated by the TME. In this nutrient-deficient milieu, characterized by high glucose consumption, recent research led by Kimryn Rathmell’s group revealed that myeloid cells display the highest capacity for glucose uptake within the tumor.Citation6 Surprisingly, cancer cells exhibit a predominant uptake of glutamine,Citation6 challenging the conventional idea of metabolic competition between cancer and immune cells in the TME. This discrepancy underscores the selective distribution of nutrients orchestrated by an intrinsic program within the TME.
3.4.1. TDSEVs-mediated metabolic reprogramming
Metabolic reprogramming, a hallmark of cancer, allows cancer cells to thrive and proliferate within the nutrient-deprived TME, with TDSEVs emerging as pivotal mediators in this process.Citation121 TDSEVs modulate the metabolism of recipient cells to satisfy energy and biosynthesis demands.Citation11 Park et al. reported that sEVs derived from pancreatic cancer are enriched in adenosine diphosphate ribosylation factor 6 (Arf6), which enhances the glycolytic rate of cancer cells, thereby regulating the Warburg effect, fulfilling the energy and nutrient requisites of tumor cells, and facilitating tumor cell proliferation.Citation122 Furthermore, TDSEVs play a crucial role in orchestrating the formation of premetastatic niches through metabolic reprogramming, thereby promoting tumor metastasis. Morrissey et al. demonstrated that TDSEVs induce the polarization of macrophages into an immunosuppressive phenotype by upregulating programmed death-ligand 1 (PD-L1) expression through NF-KB-dependent, glycolysis-dominated metabolic reprogramming, thereby establishing a premetastatic microenvironment.Citation123 Recently, Li et al. uncovered a metabolic interplay involving sEVs-glutamine-fructose-6-phosphate amidotransferase 1 (GFAT1) from BCa and heightened angiogenic activity in ECs within the nutrient-deprived TME. They proposed inhibiting sEV-mediated GFAT1 secretion and targeting seryl-tRNA synthetase (SerRS) O-GlcNAcylation in ECs as potential strategies for antiangiogenic therapy in BCa.Citation124 Moreover, TDSEVs contribute to metabolic reprogramming and remodeling of the microenvironment, exacerbating tumor angiogenesis and drug resistance. Among angiogenic factors such as proteins and nucleic acids, TDSEVs induce changes in ECs, augmenting their proliferation, migration, and angiogenic potential.Citation36,Citation125 Additionally, TDSEVs transferred from drug-resistant tumor cells to drug-sensitive counterparts confer increased resistance.Citation126,Citation127 Although TDSEVs mediate metabolic reprogramming that fuels tumor progression, studies on lipid and amino acid metabolism orchestrated by TDSEVs remain scarce. Thus, further investigation into lipid and amino acid metabolism is warranted to unravel how TDSEVs transport metabolites that foster tumor development.
3.4.2. Indirect regulation of TDSEVs release
Within the hypoxic TME, lactate production is governed by the Warburg effect, which regulates glycolytic substrate phosphorylation and mitochondrial oxidative phosphorylation. Additionally, the acidic TME indirectly influences the release of TDSEVs. Pyruvate kinase M2 (PKM2), a crucial component of the Warburg effect in tumor cells, is overexpressed in the TME. Wei et al. concluded that PKM2, upon phosphorylation and dimerization, not only switches tumor cell metabolism from oxidative phosphorylation to aerobic glycolysis but also promotes tumor cell sEV secretion by directly phosphorylating synaptosomal-associated protein 23 (SNAP-23).Citation128 The bidirectional metabolic reprogramming mediated by sEVs occurs between tumor cells and the TME. TDSEVs induce non-tumor cells in the TME to acquire a malignant phenotype, leading to the secretion of sEV cargo that regulates the metabolic reprogramming of tumor cells, thereby accelerating tumor progression and increasing TDSEV release and heterogeneity. Consequently, malignant positive feedback regulation patterns are established between the nutrient-deficient TME and tumor cells through TDSEVs.
4. Targeted TME and TDSEVs strategies
TDSEVs play a crucial role in shaping the TME and facilitating intercellular communication between cancerous and stem cells. This interaction ultimately promotes the maturation of the TME, leading to enhanced tumor growth and proliferation. Consequently, strategic targeting of TDSEVs and/or the TME presents a promising approach to prevent metastasis, overcome acquired resistance, and improve the overall efficacy of therapeutic interventions.Citation129,Citation130 Herein, we comprehensively outline the tumor’s hypoxic, acidic, metabolic and immunosuppressive microenvironment, along with the targeting strategies of TDSEVs, as illustrated in .
Figure 5. Therapeutic strategies based on targeting characteristics of the tumor microenvironment (TME) and tumor-derived small extracellular vesicles (TDSEVs). A. Targeting the hypoxic TME. These strategies mainly include the use of electron affinity radiosensitizers, enhancing tumor tissue oxygenation, hypoxia-activating prodrugs (HAPs), and targeting hypoxia-inducible factors (HIFs) and downstream targets. B. Targeting the acidic TME. This involves the development of acidic pH-selective antibodies, acidic pH-selective chimeric antigen receptor T (CAR-T) cells, and targeted inhibition of pH modulators. C. Targeting TME metabolism. Major strategies focus on aerobic glycolysis and glutamine metabolism pathways. D. Targeting the immunosuppressive TME. Strategies involve focusing on tumor-associated macrophages (TAMs) and myeloid-derived suppressor cells (MDSCs). E. Targeting TDSEVs in the TME. Major strategies include genetic manipulation, drug inhibition, and clearance of TDSEVs.
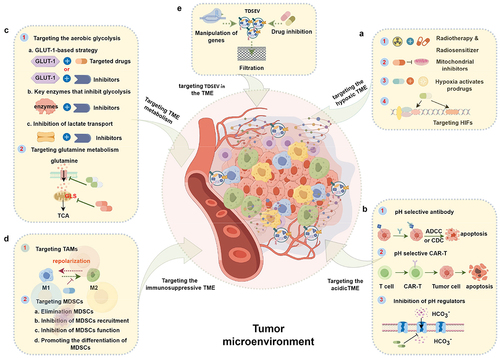
4.1. Targeted hypoxic TME strategy
Hypoxia is a prevalent condition within solid TMEs, profoundly influencing tumor cell behavior such as proliferation, invasion, and metastasis. Additionally, it significantly diminishes the effectiveness of various treatment modalities like chemotherapy, radiotherapy, and photodynamic therapy. Therefore, targeting the hypoxic TME holds promise for enhancing antitumor effectiveness.
4.1.1. Electron affinity radiosensitizer strategy
During the 1970s, it was shown that electron affinity compounds like metronidazole and Tirapazamine (TPZ), alongside hypoxia-activated cytotoxic drugs, could act as oxygen-independent radiosensitizers, enhancing the effectiveness of radiotherapy for hypoxic tumors. However, the heterogeneity of tumors and the uneven distribution of oxygen within them, especially in regions distant from blood vessels, present significant challenges for conventional hypoxia-activating drugs. As a result, sensitizing hypoxic tumors to radiotherapy remains a considerable challenge.Citation131,Citation132 In a recent study, Zhang et al. developed a multifunctional nanocapsular system incorporating cisplatin, metronidazole, and intracapsular encapsulated TPZ.Citation133 This system demonstrated synergistic enhancement of radiosensitivity, a significant reduction in radiation dose, and substantial inhibition of tumor growth and metastasis under hypoxic conditions. Meanwhile, Xiao et al. designed multifunctional Au@AgBiS2 nanoparticles as highly effective radiosensitizers with significant antitumor immune activity, preventing tumor growth and lung metastasis.Citation134 Moreover, Ma et al. utilized M1 macrophage-derived sEVs as a conceptual model to design effective radiosensitizers, achieving effective alleviation of tumor hypoxia, enhancement of DNA damage, inhibition of DNA damage repair, and ultimately remodeling the tumor suppressive microenvironment, leading to significant antitumor effects.Citation135 The demonstrated efficacy of engineered immune cell-derived sEVs as radio-sensitive agents motivates future efforts to explore different types of immune cell-derived sEVs for enhanced radiotherapy.
4.1.2. Enhancing oxygenation strategy
Starting from trials conducted in the 1960s exploring hyperbaric oxygen as a radiosensitizer, numerous clinical investigations have addressed tumor hypoxia by enhancing tissue oxygenation. In a recent study, Ashton et al. identified inhibitors targeting the mitochondrial electron transport chain (ETC) complex to impede oxygen consumption by tumor parenchyma.Citation136 Skwarski et al. demonstrated that Atovarone, an FDA-approved mitochondrial inhibitor, effectively augmented tumor oxygen levels and suppressed hypoxic gene expression in individuals diagnosed with non-small-cell lung cancer (NSCLC).Citation137 Additionally, Papaverine, an FDA-approved antispasmodic agent, and its derivatives have shown the ability to decrease mitochondrial oxygen consumption by inhibiting ETC complex I and alleviate tumor hypoxia in preclinical models.Citation138 Furthermore, several nanoparticle platforms that enhance tumor oxygenation are undergoing preclinical development, including agents that carry or produce O2.Citation139,Citation140 Advances in these therapeutic techniques to improve their stability, biocompatibility, payload composition, and tumor targeting may enable drug and TME-modified therapies to be delivered to tumors. Although there have been no previous reports on the involvement of sEVs in tumor oxygenation strategies, recently Zhong et al. showed that ischemic limb-targeting sEVs derived from stem cells and oxygen-releasing nanoparticles can effectively treat critical limb ischemia in diabetic mice.Citation141 This pioneering work suggests that co-delivery of sEVs and oxygen may be a promising strategy for tumor-targeted therapy.
4.1.3. Hypoxia-activating prodrugs strategy
Hypoxia-activated prodrugs (HAPs) display selectivity in generating active anticancer agents under hypoxic conditions and have attracted significant attention in preclinical and clinical trials as primary drugs targeting hypoxia. Among these HAPs, TPZ and Evofosfamide have progressed to Phase III trials, showing promising clinical utility, although they have not yet received regulatory approval. Recently, Huang et al. achieved a remarkable antitumor rate of over 90% through the synergistic application of battery/HAPs.Citation142 Additionally, Zhang et al. developed and synthesized a novel nanoparticle coated with TPZ as HAPs and zinc phthalocyanine (ZnPc) as a photosensitizer, demonstrating that this nanoparticle can activate HAPs effectively by photosensitive enhancement of hypoxia, thereby inhibiting glioma growth.Citation143 These innovative approaches to synergistically sensitize and activate HAPs hold promise as potential strategies for tumor suppression and regulation of the TME.
4.1.4. Targeted hypoxia-inducible factor strategy
Targeting hypoxia-inducible factors (HIFs) can disrupt their transcriptional regulatory function within the hypoxic microenvironment, thereby reducing the tolerance and adaptability of tumor cells to hypoxia. Pharmacological agents that target HIF and its downstream genes have shown significant efficacy in tumor suppression and have the potential to improve survival rates while exhibiting improved tolerability.Citation144 Notably, studies have demonstrated that HIF-2α antagonists, such as PT2385 and PT2399, can inhibit the transcriptional activity of HIF-2, indicating strong antitumor properties.Citation145 Belzutifan (PT2399), an inhibitor directly targeting HIF-2α, was shown in a phase III trial to be effective in renal cancer and other tumors in patients with von Hippel-Lindau syndrome,Citation146 and it was recently approved by the FDA for this indication. However, these pharmaceutical agents may have specific toxicological and side effects, and some clinical trials lack sufficient data to comprehensively evaluate their safety and efficacy.Citation147 Activation and inhibition of HIFs have become therapeutic targets for various cancers, with pharmacological approaches currently dominating the molecular strategy for targeting HIFs.Citation148 In the future, sEV delivery systems based on precise targeting of HIFs are likely to be explored as an option.Citation149
4.2. Targeted acidic TME strategy
The acidic pH observed in tumor environments stems from lactate production by tumor cells through the glycolytic pathway. This acidic pH creates a suppressive immune microenvironment, aiding tumor cells in evading immune detection and impacting the availability of classical antibodies. Currently, three primary strategies focus on targeting the acidic microenvironment of tumors.
4.2.1. Development of acidic pH-selective antibodies
Sulea et al. have developed a pH-dependent HER2 antibody (bH1-P5P8) that exhibits enhanced antigen binding in acidic pH compared to neutral environments, leading to significant growth inhibition.Citation150 Moreover, modifying the antibody Fc region to enable pH-dependent binding to C1q and FcγR can optimize the effector functions of antibody drugs, particularly antibody-dependent cell-mediated cytotoxicity (ADCC) and complement-dependent cytotoxicity (CDC).Citation151 In a recent study, Li et al. successfully developed a tumor-selective, pH-dependent anti-CD47 antibody (BC31M4) that demonstrates both safety and potent efficacy in a xenograft solid tumor model.Citation152 Additionally, Su et al. introduced a novel therapeutic approach involving the dual immune checkpoint blockade of CD47/PD-L1, specifically targeting the acidic microenvironment of tumors.Citation153 This intervention effectively activates CD47/PD-L1, leading to a significant enhancement of the efficacy of CD47/PD-L1 antibody treatment for lung cancer.Citation153 Despite being limited to preclinical stages at present, the application of pH-selective antibodies shows significant promise in improving the TME and addressing the challenges associated with the efficacy and safety of antibody therapy as a standalone treatment.
4.2.2. Developing acidic pH-selective CAR-T therapies
The pH-dependent antibody scFv variable fragment functions as the extracellular targeting domain of chimeric antigen receptors (CARs), allowing for the selective delivery of CAR-T cells to the acidic TME. This targeted approach presents a potent strategy for reducing off-target toxicity. In a mouse model, a HER2-directed CAR incorporating a pH-restricted binding domain showed significant expansion of CAR-T cells and regression of HER2-positive tumor cells.Citation154 However, despite the development of CAR-T products activated by acidic pH, most of these products remain in preclinical or clinical trial stages, and their safety profile continues to face significant challenges.
4.2.3. Targeted inhibition of pH regulators
Acidification of the TME is essential for driving cancer progression as this influences the composition and function of stromal cells and provides favorable conditions for the survival and proliferation of malignant cells.Citation5,Citation155 Additionally, the acidic TME directly affects the effectiveness of immune checkpoint inhibitors.Citation156 Therefore, targeting key pH regulators to prevent tumor acidification is of paramount significance. Mazzone et al. demonstrated that SLC4A4, highly expressed in pancreatic cancer, promotes the formation of an acidic TME through bicarbonate transportation. Importantly, targeted inhibition of SLC4A4 resulted in the reversal and reactivation of CD8+ T cell antitumor activity, improving immunosuppression and immunotherapy resistance.Citation157
However, while the strategies targeting the acidic TME mentioned above have demonstrated a certain level of efficacy, their safety is yet to be fully clarified. Recently, multimodal targeted therapies based on the acidic TME have shown promising prospects for clinical translation. Tang et al. developed a pH-responsive AIE light-sensitive agent for the acidic TME, expected to demonstrate promising therapeutic effects on tumors while minimizing damage to normal tissues for precision photodynamic therapy.Citation158 Gong et al. discovered that low-pH reprogrammed TDSEVs serve as a smart drug delivery platform, capable of specifically targeting tumor cells and selectively releasing various chemical drugs in response to sEV rupture caused by reactive oxygen species outbreak triggered by near-infrared irradiation. This approach demonstrates a safe and enhanced antitumor effect, with the potential for personalization for homologous tumors.Citation79 These findings pave the way for novel avenues in sEV reprogramming.
4.3. Targeting TME metabolic strategy
The metabolic activities in tumors significantly influence essential biological processes such as cell proliferation, growth, migration, and invasion. Thus, it is imperative to target tumor cell metabolic pathways and enhance the nutritional environment of the TME to prevent tumor onset and progression. Herein, we discuss potential strategies aimed at disrupting aerobic glycolytic pathways and glutamine metabolism pathways that could be considered to achieve these objectives.
4.3.1. Targeting the aerobic glycolysis pathway
The overexpression of glucose transporter 1 (GLUT-1) facilitates increased glucose uptake by tumor cells, leading to metabolic reprogramming and significant alterations in the TME.Citation159 The increased glucose uptake facilitated by overexpressed GLUT-1 has led to the development of sugar binders capable of traversing GLUT-1 and delivering anticancer agents without inhibiting GLUT-1, emerging as a promising strategy for targeted antitumor therapy. Research in this domain has primarily focused on breast cancer, with Adriamycin,Citation160,Citation161 paclitaxel,Citation162 and platinum compoundsCitation163,Citation164 serving as the principal targeted drugs. Furthermore, STF-31, a small molecule inhibitor of GLUT1, has demonstrated notable antitumor effects.Citation165 Subsequent investigations have revealed that STF-31 inhibits nicotinamide phosphoribosyltransferase (NAMPT), suggesting that GLUT1 is not the sole target of STF-31 inhibition.Citation166 Another inhibitor of GLUT1, known as Glutor, targets GLUT1, GLUT2, and GLUT3 to impede glycolysis.Citation167 BAY-876 represents the initial highly selective GLUT1 inhibitor.Citation168 However, neither Glutor nor BAY-876 has exhibited in vivo antitumor efficacy,Citation167,Citation168 and whether they possess the requisite pharmacokinetic properties for clinical application remains to be determined. Hexokinase (HK) is the initial enzymatic step in the glycolytic pathway, and its isoform HK2 has been implicated in tumorigenesis.Citation169 Various inhibitors, including 3-bromopyruvate,Citation170 glucosamine derivatives,Citation171 benitrobenrazide,Citation150 and others, have demonstrated efficacy in mouse models by specifically targeting HK2.
Pyruvate kinase (PK), an essential enzyme in glycolysis, presents in three distinct isoforms: PKM1, PKM2, and PKLR. Inhibitors targeting PKM2 are believed to exert in vivo antitumor effects on xenografts derived from NSCLC by modulating biosynthesis and reducing the metabolic flux from glucose to lactate.Citation172 However, it has been observed that PKM2 is not indispensable for tumorigenesis in specific models, prompting further investigation into the potential role of PKM2 inhibitors or activators, such as TEPP-46, in cancer therapy. Lactate dehydrogenase (LDH) exists in the form of LDHA and LDHB homotetramers and heterotetramers, which play a crucial role in the Warburg effect. In a mouse model of lung cancer, tumor suppression was achieved through the knockdown of LDHA.Citation173 Despite the development of several potent LDHA inhibitors, selective inhibition of these inhibitors using small molecules has encountered limited success.Citation174–176
Moreover, inhibiting monocarboxylate transporter 1 (MCT1) or MCT4, components of the SLC16A family responsible for lactate transportation, can lead to intracellular lactate accumulation and subsequent glycolysis suppression. Therefore, MCT1 or MCT4 inhibition demonstrates potential antitumor properties.Citation177,Citation178 Nevertheless, it is essential to acknowledge that these inhibitors can cause severe adverse effects, and their clinical effectiveness requires further validation through additional trials.
4.3.2. Targeting glutamine metabolic pathways
The alanine-serine-cysteine transporter (ASCT2) facilitates active glutamine transportation into cells, where it is converted into glutamate through deamination by mitochondrial glutaminase GLS1 and GLS2 for metabolic purposes. The ASCT2 antagonist V-9302 has demonstrated antitumor properties and has also shown the ability to impede breast cancer progression by enhancing T-cell activation.Citation179 Moreover, co-administration of V-9302 with the glutaminase inhibitor CB-839 led to a significant reduction in human HCC xenograft growth.Citation180 Additionally, in line with V-9302‘s antitumor activity and its inhibition of L-type amino acid transporter 1 (LAT1)-dependent neutral amino acid transport, LAT1 was found to be essential for tumorigenesis in KRAS mutant CRC models.Citation181
Thus far, numerous metabolic pathways have been targeted by key enzymes in cancer treatment. However, the variation in susceptibility of different tumor types to specific inhibitors warrants further investigation. Addressing the challenge of metabolic plasticity remains crucial for effectively targeting specific metabolic enzymes. To enhance safety and targeting precision, Nguyen et al. developed bioreducible sEVs that deliver acoustic sonosensitizers [triphenylphosphine coupled chlorine e6 (T-Ce6)] and glycolysis inhibitors (FX11) specifically to tumor mitochondria.Citation182 Accumulation of T-Ce6 in mitochondria leads to their destruction upon exposure to ultrasound, resulting in accelerated cell death. Concurrently, F×11inhibits energy metabolism in cells, augmenting the efficacy of delivery therapies.Citation182 This study introduces a novel approach to cancer treatment utilizing bi-stimulatory response sEVs to target hypoxic and metabolic pathways.
4.4. Targeting iTME strategy
Despite significant advances in cancer immunotherapy, immune checkpoint-targeting drugs are not yet universally available for cancer treatment. Instead, focusing on the immunosuppressive microenvironment of the tumor may hold the key to effective immunotherapy.Citation183,Citation184
4.4.1. Targeting TAMs strategy
Currently, TAM-targeting drugs are classified into four main strategies. First, TAM removal focuses on the colony-stimulating factor 1 receptor (CSF1R) pathway, which regulates TAM function. CSF1R blockers and inhibitors can disrupt CSF1R signaling, effectively depleting TAMs, and have shown efficacy in preclinical models.Citation185–187 Notable CSF1R blockers or inhibitors include Atezolizumab (RG7155), IMC-CS4, FPA008, and Pexidartinib (PLX3397).Citation188,Citation189 However, clinical use of these agents has led to serious adverse events, possibly due to off-tumor effects such as fatigue, weakness, anemia, nausea, facial and peripheral edema, lupus erythematosus, and hepatotoxicity.Citation190 Secondly, inhibiting TAM recruitment involves disrupting the influx of TAMs by blocking circulating monocytes, which heavily rely on various chemokine signals.Citation191 The CCL2/CCR2 signaling pathway regulates the recruitment of circulating monocytes into the TME, making it a promising target for TAM therapy.Citation192 Inhibition of the CCL2/CCR2 signaling pathway has demonstrated antitumor effects in various experimental animal models.Citation193 However, discontinuation of CCL2/CCR2 inhibitors can lead to a significant release of monocytes previously sequestered in the bone marrow, resulting in an overshoot of metastases and accelerated mortality.Citation194 Therefore, alternative targets that address these limitations are crucial for designing future clinical trials aimed at achieving optimal and stable therapeutic responses. Thirdly, promoting TAM phagocytic activity involves inducing direct or indirect pro-inflammatory factors such as IFN, IL4, IL13, VEGF, GM-CSF, CSF-1, Ang-2, CCL2, and other chemokines to polarize TAMs toward an M1 phenotype and exert antitumor effects. The polarization of TAMs is influenced by the interactions between cytokines, chemokines, growth factors, and their receptors.Citation195 This interaction can be utilized either independently or in combination with other strategies to enhance antitumor therapy.Citation196–198 Modified TAMs can enhance the recruitment of cytotoxic lymphocytes and boost the tumor-killing capabilities of memory T cells.Citation197 Fourthly, targeting TAM receptors (TAMR) represents a promising therapeutic approach for TAMs.Citation199 TAMR comprises a family of receptor tyrosine kinases with a shared ligand, Gas6, and protein S, which drive macrophages toward a tumor-promoting M2-like phenotype.Citation199 Thus, blocking TAMR signaling holds potential as an immunotherapy strategy. Small molecule inhibitors, antibody-drug conjugates (ADCs), chimeric antigen receptor T-cell therapy (CAR-T), and fusion proteins targeting TAMR are currently under development.Citation199
4.4.2. Targeting MDSC strategy
MDSCs present a significant obstacle to the effectiveness of immunotherapy across various cancer types, underscoring the importance of targeting them to enhance treatment outcomes.Citation200 To this end, researchers are exploring several therapeutic avenues aimed at eradicating MDSCs or neutralizing their tumor-promoting activities. These approaches primarily fall into four categories. Initially, MDSCs were targeted using antibodies against surface markers like Gr-1 or Ly6G.Citation201 Subsequent strategies have focused on more selective methods, such as S100A9-based “peptibodies”,Citation202 apoptosis induction,Citation203 and the use of chemotherapy drugs to clear MDSCs.Citation204 Treatment employing these methods has shown promise in reducing MDSC numbers, leading to immune system recovery and tumor regression.Citation204,Citation205 However, clinical trials assessing the efficacy of these approaches have been limited, with inconsistent results regarding MDSC function and abundance.
Second, inhibiting the recruitment of MDSCs to the tumor site represents another potentially important strategy. Chemokine receptors play a pivotal role in facilitating MDSC migration, making blocking their interaction with ligands a logical approach to impede MDSC aggregation in the TME. Notably, targeting the interaction between the CCL2-CCR2 and CCL5-CCR5 axes has shown promising efficacy in combating tumor growth.Citation206–208 Additionally, inhibiting CSF1R has demonstrated a reduction in MDSC recruitment. CSF1R inhibitors such as IMC-CS4, GW2580, PLX3397, and AMG820 have exhibited antitumor efficacy by impeding the survival of monocytic MDSCs (M-MDSCs) and TAMs.Citation209 Several MDSC inhibitors, including rapamycin, AZD5069, Plexidartinib and Maraviroc, have been evaluated in clinical trials, particularly in phase I and II clinical trials.Citation210
Third, reversing the immunosuppressive function of MDSCs represents another crucial strategy. Blocking the immunosuppressive mechanisms of MDSCs is fundamental for reinvigorating T cell activity and enabling successful immunotherapy. Phosphodiesterase-5 (PDE-5) inhibitors have been found to impede the function of MDSCs by reducing the expression and activity of iNOS and ARG1. Administration of PDE-5 inhibitors such as sildenafil and tadalafil in mouse models has been shown to reactivate the immune response against tumors through T cells and NK cells, leading to prolonged survival.Citation211 Clinical trials have demonstrated enhanced intratumoral T-cell activity and improved outcomes in patients with head and neck squamous cell carcinoma (HNSCC) and metastatic melanoma.Citation212,Citation213 Additionally, targeting other molecules such as phosphatidylinositol 3-kinase (PI3K), Entinostat, and STAT3 inhibitors can also reverse the immunosuppressive function of MDSCs.Citation214–216
Fourth, inducing the differentiation of MDSCs into non-suppressive cells could be another promising strategy. Administration of all-trans retinoic acid (ATRA) has been shown to accelerate the differentiation of MDSCs into mature myeloid cells, such as macrophages and DCs, thereby enhancing the T cell response in cancer patients.Citation217 Additionally, vitamin D3 is another agent that has been reported to promote the maturation of myeloid cells and reduce the number of MDSCs in cancer patients.Citation218
While all four of the above strategies targeting MDSCs have shown promising advances in preclinical applications, two major challenges hinder their translation to the clinic. First, the heterogeneity of MDSCs poses a significant obstacle to their targeted therapies.Citation219 The immunophenotypes and mechanisms of MDSCs vary across different tumor types and even within the blood and tumor tissues of the same patient. Isolating and purifying MDSCs from the TME is challenging, and distinguishing between MDSCs and normal bone marrow stem cells is unclear, limiting specific detection and targeted therapy of MDSCs in cancer.Citation220 Second, the lack of molecular studies on the differentiation process and mechanism of action of MDSCs has impeded the development of antitumor therapeutics targeting MDSCs.Citation221 In the future, further understanding of the specific mechanisms of MDSCs in tumor occurrence and development, as well as their participation in the formation and evolution of polymorphonuclear MSDCs (PMN-MDSCs), is necessary to improve the verification and detection of MDSC phenotypes. This understanding will be conducive to enhancing targeted therapy strategies for MDSCs.
4.5. Inhibition strategies for TDSEVs
Emerging novel strategies, such as genetic manipulation, pharmacological inhibition, and TDSEV clearance, are being developed to selectively inhibit TDSEVs by targeting their biological production and release mechanism.
4.5.1. Genetic manipulation strategies
Prominent biotechnological tools, such as RNA interference (RNAi) and CRISPR-Cas9 systems, have been extensively used to attenuate or disrupt the expression of essential genes involved in TDSEV biogenesis and secretion.Citation222 The ESCRT pathway, pivotal in the mechanism of TDSEV biogenesis, plays an important role in this process. Using RNAi, a research team from Colombo silenced ESCRT-related components (HRS, STAM1, TSG101, etc.) in HeLa cells, leading to reduced secretion of TDSEVs and sEV MHC class II.Citation223 Similarly, Hoshino et al. knocked down HRS in SCC61 HNSCC cells, which significantly decreased the secretion of sEVs and proteins.Citation224 Disruption of HRS expression by RNAi has also been shown to markedly reduce sEV PD-L1 levels.Citation92 Consequently, targeting HRS holds promise for efficiently suppressing both TDSEVs and cargo.
Additionally, inhibition of TDSEVs has been reported to target Rab GTPases involved in intracellular vesicle transport. RNAi-mediated gene knockdowns of Rab27a or Rab27b have been reported to inhibit sEV secretion in various tumor cells, including BCa cells,Citation225 HNSCCs,Citation224,Citation226 and cervical cancer cells.Citation39 Similarly, Poggio et al. used CRISPR-Cas9 to knock out nSMase2 or Rab27a, achieving inhibition of sEV secretion in prostate cancer cells.Citation227 However, deleting nSMase2 only partially eliminated TDSEVs, indicating the need for further investigation into more effective strategies or targets. Additionally, RNAi targeting Rab7 in MCF-7 cells inhibited MVB transport and resulted in reduced TDSEV secretion.Citation228 Recently, it was shown that Rab31 regulates sEV biogenesis in HeLa cells through an ESCRT-independent pathway and drives cargo sorting, offering novel targets for TDSEV and cargo inhibition in future therapies.Citation229 For SNARE proteins (i.e., syntaxin 6, VAMP7, and YKT6), which mediate the fusion of MVB with the cell membrane, down-regulation of syntaxin 6 expression significantly reduced TDSEV secretion by prostate cancer cells.Citation230 Furthermore, Ruiz-Martinez et al. found YKT6 inhibition of TDSEV secretion in A549 cells.Citation231 TDSEV inhibition strategies based on genetic manipulation have promising applications in cancer research, but they still face many challenges.Citation232 First, off-target effects can reduce blocking effects and raise safety concerns.Citation233 Second, the specificity of current inhibition strategies is limited, as blocking TDSEVs also blocks non-TDSEV secretion, potentially inducing side effects in tumor therapy. Third, biosafety concerns may arise with genetic modification based on viral systems.Citation234 Therefore, a comprehensive understanding of TDSEV biogenesis and secretion is needed to identify more potential targets for future translation. Moreover, efforts should focus on developing multi-target strategies to inhibit each critical step in TDSEV generation, leading to a whole pathway of TDSEV inhibition.Citation227
4.5.2. Pharmacological inhibition strategies
Pharmacological inhibitors of TDSEVs have been extensively studied in recent decades and have shown great promise for therapeutic applications. Currently, pharmacological inhibition strategies can be divided into three categories, namely, TDSEV inhibitors, specific inhibition of genomic mutations, and shared regulatory mechanisms.
TDSEV inhibitors can be used to inhibit TDSEV biogenesis and secretion mechanisms. Among these inhibitors, GW4869 is widely recognized as the most frequently employed. In both in vitro and in vivo experiments, GW4869 inhibited sEV secretion from various tumor cells, including breast cancer cells,Citation235 epidermal cancer cells,Citation236 BCa cells,Citation225 HNSCC cells,Citation224 and malignant melanoma cells,Citation237 thereby promoting antitumor immunity.Citation235,Citation238–240 Yang et al. found that GW4869 inhibited TDSEV secretion and reduced the total protein content in breast cancer cells.Citation235 Moreover, in a mouse model of breast cancer, GW4869 suppressed tumor growth by inhibiting TDSEV, thereby promoting antitumor effects and significantly enhancing the therapeutic effect of the anti-PD-L1 antibody.Citation235 Wang et al. used hyaluronic acid to assemble nano-units of GW4869 and a ferro-death inducer (Fe3+), and the collaboration of the two active ingredients induced an antitumor immune response against B16F10 melanoma cells and stimulated cytotoxic T lymphocytes and immune memory.Citation238 Although GW4869-based inhibition strategies have been reported in a large number of cases, several drawbacks limit their practical application. First, GW4869 is an nSMase2 blocker that mediates the biogenesis and secretion of TDSEV and non-TDSEV in an ESCRT-independent pathway.Citation240 Thus, direct application of GW4896 without targeted delivery may result in nonspecific inhibition of non-TDSEV. Second, GW4869 removes nSMase in a noncompetitive manner, which may lead to less efficient TDSEV inhibition.Citation239 Lastly, nSMase2 has been shown to be involved in multiple important biological processes,Citation241 and GW4869 efficacy should be assessed in terms of biosafety before clinical application.
Genomic mutations that cause aberrant biogenesis and secretion of TDSEVs can also be pharmacologically inhibited. The RAS/RAF/ERK signaling pathway plays an essential role in intracellular and intercellular communication processes. The abnormal activation of the RAS signaling pathway is closely related to tumorigenesis. Farnesyltransferases (FTases) are the key enzymes for RAS protein activation. Thus, FTase inhibitors are antitumor drugs that target the Ras protein.Citation242 It has been found that the FTase inhibitors manumycin-A and Tipifarnib lead to the inhibition of TDSEV secretion by prostate cancer cells by selectively affecting the RAS/RAF/ERK pathway.Citation243,Citation244 Recently, Sasabe et al. found that EGFR inhibitors inhibit the malignant potential of oral squamous cell carcinoma (OSCC) cells by directly inhibiting EGFR downstream signaling pathways as well as by inhibiting the uptake of TDSEVs through macropinocytosis,Citation245 which confirms that anti-EGFR agents may be TDSEV inhibitors. Ketoconazole (KTZ) has been shown to inhibit pathways of sEV biogenesis and secretion.Citation246 Greenberg et al. provided the latest evidence for the use of TDSEV inhibitors as a novel option for cancer treatment by enhancing the efficacy of sunitinib by adding KTZ to cause TDSEVs inhibition and reduce tumor proliferation in sunitinib-resistant renal cancer cells (786-O).Citation247
Additionally, targeting the shared regulatory mechanisms and inhibition of the TME or genomic mutations could be considered. Given the observed over-expression and/or over-activation of key regulators in tumor cells, these molecules represent promising candidates for TDSEV inhibition.Citation248,Citation249 Syntenin-Syndecan-ALIX plays a key role in the biogenesis of EVs.Citation228 It has been established that heparan sulfate analogs specifically and effectively inhibit the secretion of TDSEVs by B16F10 melanoma cells via targeted Syndecan-Syntenin-Alix, resulting in attenuated tumor proliferation and invasion.Citation248 Im et al. used sulfamisoxazole (SFX) to selectively inhibit the transcription of Rab GTPases (Rab5, Rab7, and Rab27a) and ESCRT components (Alix, VPS4B), which inhibited multivesicular body (MVB) formation and secretion and ultimately resulted in the inhibition of TDSEV secretion.Citation250
However, pharmacological inhibition strategies are limited in terms of the complexity of TDSEV biosynthesis and secretion pathways, requiring the development of multi-target inhibitors to effectively block TDSEV production. Moreover, the diversity of circulating sEVs presents challenges in selectively inhibiting TDSEV, thus requiring careful targeting to avoid affecting non-TDSEVs.Citation251 Exploring modifications to the TMEs or targeting relevant signaling pathways may provide novel avenues for TDSEV inhibition. Additionally, the development of pharmacological inhibitors is a lengthy process and costly.Citation252,Citation253 Nevertheless, implementing a high-throughput screening system for TDSEV inhibitors, coupled with quantitative assays to simultaneously assess multiple candidate inhibitors, might enhance effectiveness.Citation243,Citation244,Citation250
4.5.3. Clearance strategies for TDSEVs
Orme et al. introduced therapeutic plasma exchange as a method to eliminate TDSEVs from the bloodstream in patients diagnosed with malignant melanoma.Citation254 Dialysis, another popular method for removing detrimental substances from the circulatory system, utilizes semi-permeable membranes with diameters smaller than 1 nm to effectively remove toxicants. Microporous membranes of suitable size are anticipated to be employed for TDSEV removal. Wu et al. utilized silica microspheres and hemofiltration devices to capture and eliminate a substantial quantity of circulating tumor cells and TDSEV, presenting a promising tumor treatment avenue.Citation255 The use of microfluidic chips, which have excellent compatibility and minute dimensions, allows the integration of numerous antibody-coated units to efficiently eradicate TDSEV, resulting in rapid and direct elimination.Citation256
Current strategies for removing TDSEV using ex vivo devices have limitations such as trauma, bleeding, and infection risks. Additionally, dialysis can remove both harmful and beneficial sEVs, further burdening cancer patients. Future strategies should aim to be more specific and gentle.Citation254,Citation257 There is evidence suggesting that the role of phagocytic clearance by macrophages may be altered in the TME.Citation258 High PD-1 levels in TME macrophages hinder phagocytosis, but blocking PD-1 could potentially boost TDSEV clearance. In the case of HNSCC, TDSEVs inhibit phagocytosis through CD73, thereby promoting tumor growth.Citation259 Therefore, activating macrophage phagocytosis in vivo may prove more effective than creating a clearance system in vitro.
5. Summary and prospect
The TME plays an essential role in tumor development, invasion, and metastasis. Stress conditions such as hypoxia, starvation, and acidosis can induce heterogeneous changes in the release of sEVs by tumor cells, affecting cargo sorting and transport, thereby contributing to malignant proliferation, metastasis, and tumor resistance to treatment. TDSEVs activate immune cells and drive antitumor immunity through TAAs in their cargo. Furthermore, the antigens and MHC molecules they carry enable the development of anticancer vaccines. However, the ability of TDSEVs to stimulate the immune system and thus prevent tumor progression remains challenging. While strategies targeting the inhibition and removal of TDSEVs have shown promise in cancer treatment, the highly heterogeneous physical characteristics and molecular composition of TDSEVs within the TME remain bottlenecks for clinical translation. Off-target effects and biological safety risks limit gene manipulation in TDSEV inhibition for clinical applications. Therapeutic strategies targeting the TME have demonstrated beneficial antitumor effects in preclinical studies. However, while remodeling the TME improves targeting efficiency, it also increases the risk of tumor migration and metastasis. Tumor heterogeneity and complexity pose major challenges for TME remodeling. In recent years, sEVs have emerged as promising vehicles for drug delivery. Compared to conventional nanocarriers, sEVs offer advantages in terms of immunogenicity, in vivo barrier crossing, and drug metabolism, presenting a promising avenue for tumor-targeted therapy. Therefore, harnessing the information transmission properties of EVs, enhancing TME targeting efficiency and improving susceptibility to immunotherapy offer a potential strategy for developing effective and safe delivery systems based on TDSEVs and/or TME modulation. Collectively, this multimodal approach could hold promising potential for overcoming resistance to tumor immunotherapy in the future.
Author contributions
Conceptualization, Guo Y.C., Guo X.Y., Song J.J., Liu M., and Ou X.Y.; validation, Guo Y.C., and Guo X.Y.; Data Curation, Guo X.Y., Song J.J., Liu M., and Ou X.Y.; investigation, Guo Y.C., Guo X.Y. and Song J.J.; writing – original draft preparation, Guo X.Y.; writing – review and editing, Guo Y.C., Guo X.Y., Song J.J., Liu M., and Ou X.Y.; visualization, Guo Y.C., Guo X.Y. and Song J.J.; supervision, Guo Y.C.; project administration, Guo Y.C.; funding acquisition, Guo Y.C. All authors have read and agreed to the published version of the manuscript.
Acknowledgments
All the images in the manuscript were created with figdraw.com on 22 or 23 Aug 2023. We acknowledge Figdraw for providing us with their platform for the preparation of images.
Disclosure statement
No potential conflict of interest was reported by the author(s).
Additional information
Funding
References
- Hui L, Chen Y. Tumor microenvironment: sanctuary of the devil. Cancer Lett. 2015;368(1):7–23. doi: 10.1016/j.canlet.2015.07.039.
- Zhou J, Li Q, Cao Y. Spatiotemporal heterogeneity across metastases and organ-specific response informs drug efficacy and patient survival in colorectal cancer. Cancer Res. 2021;81(9):2522–2533. doi: 10.1158/0008-5472.CAN-20-3665.
- Song M, Liu Q, Sun W, Zhang H. Crosstalk between thyroid carcinoma and tumor-correlated immune cells in the tumor microenvironment. Cancers Basel. 2023;15(10):2863. doi: 10.3390/cancers15102863.
- Michiels C, Tellier C, Feron O. Cycling hypoxia: a key feature of the tumor microenvironment. Biochim Biophys Acta. 2016;1866(1):76–86. doi: 10.1016/j.bbcan.2016.06.004.
- Boedtkjer E, Pedersen SF. The acidic tumor microenvironment as a driver of cancer. Annu Rev Physiol. 2020;82(1):103–126. doi: 10.1146/annurev-physiol-021119-034627.
- Reinfeld BI, Madden MZ, Wolf MM, Chytil A, Bader JE, Patterson AR, Sugiura A, Cohen AS, Ali A, Do BT, et al. Cell-programmed nutrient partitioning in the tumour microenvironment. Nature. 2021;593(7858):282–288. doi: 10.1038/s41586-021-03442-1.
- Gurusamy D, Clever D, Eil R, Restifo NP. Novel “elements” of immune suppression within the tumor microenvironment. Cancer Immunol Res. 2017;5(6):426–433. doi: 10.1158/2326-6066.CIR-17-0117.
- Welsh JA, Goberdhan DCI, O’Driscoll L, Buzas EI, Blenkiron C, Bussolati B, Cai H, Di Vizio D, Driedonks TAP, Erdbrügger U, et al. Minimal information for studies of extracellular vesicles (MISEV2023): from basic to advanced approaches. J Extracell Vesicles. 2024;13(2):e12404. doi: 10.1002/jev2.12404.
- Paskeh MDA, Entezari M, Mirzaei S, Zabolian A, Saleki H, Naghdi MJ, Sabet S, Khoshbakht MA, Hashemi M, Hushmandi K, et al. Emerging role of exosomes in cancer progression and tumor microenvironment remodeling. J Hematol Oncol. 2022;15(1):83. doi: 10.1186/s13045-022-01305-4.
- Wang X, Huang J, Chen W, Li G, Li Z, Lei J. The updated role of exosomal proteins in the diagnosis, prognosis, and treatment of cancer. Experiment Molecul Med. 2022;54(9):1390–1400. doi: 10.1038/s12276-022-00855-4.
- Tan S, Yang Y, Yang W, Han Y, Huang L, Yang R, Hu Z, Tao Y, Liu L, Li Y, et al. Exosomal cargos-mediated metabolic reprogramming in tumor microenvironment. J Exp Clin Cancer Res. 2023;42(1):59. doi: 10.1186/s13046-023-02634-z.
- Logozzi M, Mizzoni D, Angelini DF, Di Raimo R, Falchi M, Battistini L, Fais S. Microenvironmental pH and exosome levels interplay in human cancer cell lines of different histotypes. Cancers Basel. 2018;10(10):370. doi: 10.3390/cancers10100370.
- Suetsugu A, Honma K, Saji S, Moriwaki H, Ochiya T, Hoffman RM. Imaging exosome transfer from breast cancer cells to stroma at metastatic sites in orthotopic nude-mouse models. Adv Drug Deliv Rev. 2013;65(3):383–390. doi: 10.1016/j.addr.2012.08.007.
- Kalluri R, LeBleu VS. The biology, function, and biomedical applications of exosomes. Science. 2020;367(6478):eaau6977. doi: 10.1126/science.aau6977.
- Raposo G, Stoorvogel W. Extracellular vesicles: exosomes, microvesicles, and friends. J Cell Biol. 2013;200(4):373–383. doi: 10.1083/jcb.201211138.
- Urbanelli L, Magini A, Buratta S, Brozzi A, Sagini K, Polchi A, Tancini B, Emiliani C. Signaling pathways in exosomes biogenesis, secretion and fate. Genes. 2013;4(2):152–170. doi: 10.3390/genes4020152.
- Rastogi S, Sharma V, Bharti PS, Rani K, Modi GP, Nikolajeff F, Kumar S. The evolving landscape of exosomes in neurodegenerative diseases: exosomes characteristics and a promising role in early diagnosis. Int J Mol Sci. 2021;22(1):440. doi: 10.3390/ijms22010440.
- Radulovic M, Stenmark H. ESCRTs in membrane sealing. Biochem Soc Trans. 2018;46(4):773–778. doi: 10.1042/BST20170435.
- Kenific CM, Zhang H, Lyden D. An exosome pathway without an ESCRT. Cell Res. 2021;31(2):105–106. doi: 10.1038/s41422-020-00418-0.
- Cocucci E, Meldolesi J. Ectosomes and exosomes: shedding the confusion between extracellular vesicles. Trends Cell Biol. 2015;25(6):364–372. doi: 10.1016/j.tcb.2015.01.004.
- Colombo M, Raposo G, Théry C. Biogenesis, secretion, and intercellular interactions of exosomes and other extracellular vesicles. Annu Rev Cell Dev Biol. 2014;30(1):255–289. doi: 10.1146/annurev-cellbio-101512-122326.
- Antonyak MA, Wilson KF, Cerione RA. R(h)oads to microvesicles. Small GTPases. 2012;3(4):219–224. doi: 10.4161/sgtp.20755.
- Muralidharan-Chari V, Clancy J, Plou C, Romao M, Chavrier P, Raposo G, D’Souza-Schorey C. ARF6-regulated shedding of tumor cell-derived plasma membrane microvesicles. Curr Biol. 2009;19(22):1875–1885. doi: 10.1016/j.cub.2009.09.059.
- Sedgwick AE, Clancy JW, Olivia Balmert M, D’Souza-Schorey C. Extracellular microvesicles and invadopodia mediate non-overlapping modes of tumor cell invasion. Sci Rep. 2015;5(1):14748. doi: 10.1038/srep14748.
- Palmisano G, Jensen SS, Le Bihan MC, Lainé J, McGuire JN, Pociot F, Larsen MR. Characterization of membrane-shed microvesicles from cytokine-stimulated β-cells using proteomics strategies. Molecul Cellul Proteomic: MCP. 2012;11(8):230–243. doi: 10.1074/mcp.M111.012732.
- Tricarico C, Clancy J, D’Souza-Schorey C. Biology and biogenesis of shed microvesicles. Small GTPases. 2017;8(4):220–232. doi: 10.1080/21541248.2016.1215283.
- Li S, Man Q, Gao X, Lin H, Wang J, Su F-C, Wang H-Q, Bu L-L, Liu B, Chen G, et al. Tissue‐derived extracellular vesicles in cancers and non‐cancer diseases: present and future. J Extracellular Vesicle. 2021;10(14):e12175. doi: 10.1002/jev2.12175.
- Bai S, Wang Z, Wang M, Li J, Wei Y, Xu R, Du J. Tumor-derived exosomes modulate primary site tumor metastasis. Front Cell Dev Biol. 2022;10:752818. doi: 10.3389/fcell.2022.752818.
- Han QF, Li WJ, Hu KS, Gao J, Zhai W-L, Yang J-H, Zhang S-J. Exosome biogenesis: machinery, regulation, and therapeutic implications in cancer. Mol Cancer. 2022;21(1):207. doi: 10.1186/s12943-022-01671-0.
- De Toro J, Herschlik L, Waldner C, Mongini C. Emerging roles of exosomes in normal and pathological conditions: new insights for diagnosis and therapeutic applications. Front Immunol. 2015;6:203. doi: 10.3389/fimmu.2015.00203.
- Kosaka N. Decoding the secret of cancer by means of extracellular vesicles. JCM. 2016;5(2):22. doi: 10.3390/jcm5020022.
- Ciardiello C, Cavallini L, Spinelli C, Yang J, Reis-Sobreiro M, de Candia P, Minciacchi V, Di Vizio D. Focus on extracellular vesicles: new frontiers of cell-to-cell communication in cancer. Int J Mol Sci. 2016;17(2):175. doi: 10.3390/ijms17020175.
- Falasca M, Kim M, Casari I. Pancreatic cancer: current research and future directions. Biochim Biophys Acta. 2016;1865(2):123–132. doi: 10.1016/j.bbcan.2016.01.001.
- Shao H, Chung J, Issadore D. 2016. Diagnostic technologies for circulating tumour cells and exosomes. Biosci Rep. 36(1):e00292. doi: 10.1042/BSR20150180.
- Robbins PD, Morelli AE. Regulation of immune responses by extracellular vesicles. Nat Rev Immunol. 2014;14(3):195–208. doi: 10.1038/nri3622.
- King HW, Michael MZ, Gleadle JM. Hypoxic enhancement of exosome release by breast cancer cells. BMC Cancer. 2012;12(1):421. doi: 10.1186/1471-2407-12-421.
- Yu X, Harris SL, Levine AJ. The regulation of exosome secretion: a novel function of the p53 protein. Cancer Res. 2006;66(9):4795–4801. doi: 10.1158/0008-5472.CAN-05-4579.
- Thompson CA, Purushothaman A, Ramani VC, Vlodavsky I, Sanderson RD. Heparanase regulates secretion, composition, and function of tumor cell-derived exosomes. J Biol Chem. 2013;288(14):10093–10099. doi: 10.1074/jbc.C112.444562.
- Ostrowski M, Carmo NB, Krumeich S, Fanget I, Raposo G, Savina A, Moita CF, Schauer K, Hume AN, Freitas RP, et al. Rab27a and Rab27b control different steps of the exosome secretion pathway. Nat Cell Biol. 2010;12(1):19–30. doi: 10.1038/ncb2000.
- Bobrie A, Théry C. Unraveling the physiological functions of exosome secretion by tumors. Oncoimmunol. 2013;2(1):e22565. doi: 10.4161/onci.22565.
- Peinado H, Alečković M, Lavotshkin S, Matei I, Costa-Silva B, Moreno-Bueno G, Hergueta-Redondo M, Williams C, García-Santos G, Ghajar CM, et al. Melanoma exosomes educate bone marrow progenitor cells toward a pro-metastatic phenotype through MET. Nat Med. 2012;18(6):883–891. doi: 10.1038/nm.2753.
- Whiteside TL. Exosomes carrying immunoinhibitory proteins and their role in cancer. Clin Exp Immunol. 2017;189(3):259–267. doi: 10.1111/cei.12974.
- Wu B, Liu DA, Guan L, Myint PK, Chin L, Dang H, Xu Y, Ren J, Li T, Yu Z, et al. Stiff matrix induces exosome secretion to promote tumour growth. Nat Cell Biol. 2023;25(3):415–424. doi: 10.1038/s41556-023-01092-1.
- Luo C, Xin H, Zhou Z, Hu Z, Sun R, Yao N, Sun Q, Borjigin U, Wu X, Fan J, et al. Tumor-derived exosomes induce immunosuppressive macrophages to foster intrahepatic cholangiocarcinoma progression. Hepatology. 2022;76(4):982–999. doi: 10.1002/hep.32387.
- Bister N, Pistono C, Huremagic B, Jolkkonen J, Giugno R, Malm T. Hypoxia and extracellular vesicles: a review on methods, vesicular cargo and functions. J Extracell Vesicles. 2020;10(1):e12002. doi: 10.1002/jev2.12002.
- Kumar A, Deep G. Hypoxia in tumor microenvironment regulates exosome biogenesis: molecular mechanisms and translational opportunities. Cancer Lett. 2020;479:23–30. doi: 10.1016/j.canlet.2020.03.017.
- Jung KO, Jo H, Yu JH, Gambhir SS, Pratx G. Development and MPI tracking of novel hypoxia-targeted theranostic exosomes. Biomater. 2018;177:139–148. doi: 10.1016/j.biomaterials.2018.05.048.
- Wang Y, Yin K, Tian J, Xia X, Ma J, Tang X, Xu H, Wang S. Granulocytic myeloid-derived suppressor cells promote the stemness of colorectal cancer cells through Exosomal S100A9. Adv Sci (Weinh). 2019;6(18):1901278. doi: 10.1002/advs.201901278.
- Ren R, Sun H, Ma C, Liu J, Wang H. Colon cancer cells secrete exosomes to promote self-proliferation by shortening mitosis duration and activation of STAT3 in a hypoxic environment. Cell Biosci. 2019;9(1):62. doi: 10.1186/s13578-019-0325-8.
- Li J, Yuan H, Xu H, Zhao H, Xiong N. Hypoxic cancer-secreted exosomal miR-182-5p promotes glioblastoma angiogenesis by targeting Kruppel-like factor 2 and 4. Mol Cancer Res. 2020;18(8):1218–1231. doi: 10.1158/1541-7786.MCR-19-0725.
- Xia X, Wang S, Ni B, Xing S, Cao H, Zhang Z, Yu F, Zhao E, Zhao G. Hypoxic gastric cancer-derived exosomes promote progression and metastasis via MiR-301a-3p/PHD3/HIF-1α positive feedback loop. Oncogene. 2020;39(39):6231–6244. doi: 10.1038/s41388-020-01425-6.
- Matsuura Y, Wada H, Eguchi H, Gotoh K, Kobayashi S, Kinoshita M, Kubo M, Hayashi K, Iwagami Y, Yamada D, et al. Exosomal miR-155 derived from hepatocellular carcinoma cells under hypoxia promotes angiogenesis in endothelial cells. Dig Dis Sci. 2019;64(3):792–802. doi: 10.1007/s10620-018-5380-1.
- Patton MC, Zubair H, Khan MA, Singh S, Singh AP. Hypoxia alters the release and size distribution of extracellular vesicles in pancreatic cancer cells to support their adaptive survival. J Cell Biochem. 2020;121(1):828–839. doi: 10.1002/jcb.29328.
- Ramteke A, Ting H, Agarwal C, Mateen S, Somasagara R, Hussain A, Graner M, Frederick B, Agarwal R, Deep G, et al. Exosomes secreted under hypoxia enhance invasiveness and stemness of prostate cancer cells by targeting adherens junction molecules. Mol Carcinog. 2015;54(7):554–565. doi: 10.1002/mc.22124.
- Liu W, Li L, Rong Y, Qian D, Chen J, Zhou Z, Luo Y, Jiang D, Cheng L, Zhao S, et al. Hypoxic mesenchymal stem cell-derived exosomes promote bone fracture healing by the transfer of miR-126. Acta Biomater. 2020;103:196–212. doi: 10.1016/j.actbio.2019.12.020.
- Zhang W, Zhou X, Yao Q, Liu Y, Zhang H, Dong Z. HIF-1-mediated production of exosomes during hypoxia is protective in renal tubular cells. Am J Physiol Renal Physiol. 2017;313(4):F906–F913. doi: 10.1152/ajprenal.00178.2017.
- Zhu LP, Tian T, Wang JY, He J-N, Chen T, Pan M, Xu L, Zhang H-X, Qiu X-T, Li C-C, et al. Hypoxia-elicited mesenchymal stem cell-derived exosomes facilitates cardiac repair through miR-125b-mediated prevention of cell death in myocardial infarction. Theranostic. 2018;8(22):6163–6177. doi: 10.7150/thno.28021.
- van Niel G, D’Angelo G, Raposo G. Shedding light on the cell biology of extracellular vesicles. Nat Rev Mol Cell Biol. 2018;19(4):213–228. doi: 10.1038/nrm.2017.125.
- Villarroya-Beltri C, Baixauli F, Mittelbrunn M, Fernández-Delgado I, Torralba D, Moreno-Gonzalo O, Baldanta S, Enrich C, Guerra S, Sánchez-Madrid F, et al. ISGylation controls exosome secretion by promoting lysosomal degradation of MVB proteins. Nat Commun. 2016;7(1):13588. doi: 10.1038/ncomms13588.
- Zieseniss A. Hypoxia and the modulation of the actin cytoskeleton - emerging interrelations. Hypoxia (Auckl). 2014;2:11–21. doi: 10.2147/HP.S53575.
- Cao H, Yu D, Yan X, Wang B, Yu Z, Song Y, Sheng L. Hypoxia destroys the microstructure of microtubules and causes dysfunction of endothelial cells via the PI3K/Stathmin1 pathway. Cell & Bioscience. 2019;9(1):20. doi: 10.1186/s13578-019-0283-1.
- Lee HJ, Jung YH, Oh JY, Choi GE, Chae CW, Kim JS, Lim JR, Kim SY, Lee S-J, Seong JK, et al. BICD1 mediates HIF1α nuclear translocation in mesenchymal stem cells during hypoxia adaptation. Cell Death Differ. 2019;26(9):1716–1734. doi: 10.1038/s41418-018-0241-1.
- He G, Peng X, Wei S, Yang S, Li X, Huang M, Tang S, Jin H, Liu J, Zhang S, et al. Exosomes in the hypoxic TME: from release, uptake and biofunctions to clinical applications. Mol Cancer. 2022;21(1):19. doi: 10.1186/s12943-021-01440-5.
- Bjørnetrø T, Redalen KR, Meltzer S, Thusyanthan NS, Samiappan R, Jegerschöld C, Handeland KR, Ree AH. An experimental strategy unveiling exosomal microRnas 486-5p, 181a-5p and 30d-5p from hypoxic tumour cells as circulating indicators of high-risk rectal cancer. J Extracell Vesicles. 2019;8(1):1567219. doi: 10.1080/20013078.2019.1567219.
- Zhang X, Sai B, Wang F, Wang L, Wang Y, Zheng L, Li G, Tang J, Xiang J. Hypoxic BMSC-derived exosomal miRnas promote metastasis of lung cancer cells via STAT3-induced EMT. Mol Cancer. 2019;18(1):40. doi: 10.1186/s12943-019-0959-5.
- Mo F, Xu Y, Zhang J, Zhu L, Wang C, Chu X, Pan Y, Bai Y, Shao C, Zhang J, et al. Effects of hypoxia and radiation-induced exosomes on migration of lung cancer cells and angiogenesis of umbilical vein endothelial cells. Radiat Res. 2020;194(1):71–80. doi: 10.1667/RR15555.1.
- Deep G, Jain A, Kumar A, Agarwal C, Kim S, Leevy WM, Agarwal R. Exosomes secreted by prostate cancer cells under hypoxia promote matrix metalloproteinases activity at pre-metastatic niches. Mol Carcinog. 2020;59(3):323–332. doi: 10.1002/mc.23157.
- Hannafon BN, Gin AL, Xu YF, Bruns M, Calloway CL, Ding WQ. Metastasis-associated protein 1 (MTA1) is transferred by exosomes and contributes to the regulation of hypoxia and estrogen signaling in breast cancer cells. Cell Commun Signal. 2019;17(1):13. doi: 10.1186/s12964-019-0325-7.
- Wang W, Han Y, Jo HA, Lee J, Song YS. Non-coding RNAs shuttled via exosomes reshape the hypoxic tumor microenvironment. J Hematol Oncol. 2020;13(1):67. doi: 10.1186/s13045-020-00893-3.
- Yu Y, Min Z, Zhihang Z, Linhong M, Tao R, Yan L, Song H. Hypoxia-induced exosomes promote hepatocellular carcinoma proliferation and metastasis via miR-1273f transfer. Experiment Cell Res. 2019;385(1):111649. doi: 10.1016/j.yexcr.2019.111649.
- Liu Y, Tan J, Ou S, Chen J, Chen L. Adipose-derived exosomes deliver miR-23a/b to regulate tumor growth in hepatocellular cancer by targeting the VHL/HIF axis. J Physiol Biochem. 2019;75(3):391–401. doi: 10.1007/s13105-019-00692-6.
- Xue M, Chen W, Xiang A, Wang R, Chen H, Pan J, Pang H, An H, Wang X, Hou H, et al. Hypoxic exosomes facilitate bladder tumor growth and development through transferring long non-coding RNA-UCA1. Mol Cancer. 2017;16(1):143. doi: 10.1186/s12943-017-0714-8.
- Li L, Cao B, Liang X, Lu S, Luo H, Wang Z, Wang S, Jiang J, Lang J, Zhu G, et al. Microenvironmental oxygen pressure orchestrates an anti- and pro-tumoral γδ T cell equilibrium via tumor-derived exosomes. Oncogene. 2019;38(15):2830–2843. doi: 10.1038/s41388-018-0627-z.
- Wang X, Luo G, Zhang K, Cao J, Huang C, Jiang T, Liu B, Su L, Qiu Z. Hypoxic tumor-derived exosomal miR-301a mediates M2 macrophage polarization via PTEN/PI3Kγ to promote pancreatic cancer metastasis. Cancer Res. 2018;78(16):4586–4598. doi: 10.1158/0008-5472.CAN-17-3841.
- Chen LQ, Pagel MD. Evaluating pH in the extracellular tumor microenvironment using CEST MRI and other imaging methods. Adv Radiol. 2015;2015:1–25. doi: 10.1155/2015/206405.
- Ban JJ, Lee M, Im W, Kim M. Low pH increases the yield of exosome isolation. Biochem Biophys Res Commun. 2015;461(1):76–79. doi: 10.1016/j.bbrc.2015.03.172.
- Parolini I, Federici C, Raggi C, Lugini L, Palleschi S, De Milito A, Coscia C, Iessi E, Logozzi M, Molinari A, et al. Microenvironmental pH is a key factor for exosome traffic in tumor cells. J Biol Chem. 2009;284(49):34211–34222. doi: 10.1074/jbc.M109.041152.
- Logozzi M, Angelini DF, Iessi E, Mizzoni D, Di Raimo R, Federici C, Lugini L, Borsellino G, Gentilucci A, Pierella F, et al. Increased PSA expression on prostate cancer exosomes in in vitro condition and in cancer patients. Cancer Lett. 2017;403:318–329. doi: 10.1016/j.canlet.2017.06.036.
- Gong C, Zhang X, Shi M, Li F, Wang S, Wang Y, Wang Y, Wei W, Ma G. Tumor exosomes reprogrammed by low pH are efficient targeting vehicles for smart drug delivery and personalized therapy against their homologous tumor. Adv Sci (Weinh). 2021;8(10):2002787. doi: 10.1002/advs.202002787.
- Hisey CL, Dorayappan KDP, Cohn DE, Selvendiran K, Hansford DJ. Microfluidic affinity separation chip for selective capture and release of label-free ovarian cancer exosomes. Lab Chip. 2018;18(20):3144–3153. doi: 10.1039/c8lc00834e.
- Tian XP, Wang CY, Jin XH, Li M, Wang F-W, Huang W-J, Yun J-P, Xu R-H, Cai Q-Q, Xie D, et al. Acidic microenvironment up-regulates exosomal miR-21 and miR-10b in early-stage hepatocellular carcinoma to promote cancer cell proliferation and metastasis. Theranostic. 2019;9(7):1965–1979. doi: 10.7150/thno.30958.
- He L, Zhu W, Chen Q, Yuan Y, Wang Y, Wang J, Wu X. Ovarian cancer cell-secreted exosomal miR-205 promotes metastasis by inducing angiogenesis. Theranostic. 2019;9(26):8206–8220. doi: 10.7150/thno.37455.
- Zeng Z, Li Y, Pan Y, Lan X, Song F, Sun J, Zhou K, Liu X, Ren X, Wang F, et al. Cancer-derived exosomal miR-25-3p promotes pre-metastatic niche formation by inducing vascular permeability and angiogenesis. Nat Commun. 2018;9(1):5395. doi: 10.1038/s41467-018-07810-w.
- Zhou CF, Ma J, Huang L, Yi H-Y, Zhang Y-M, Wu X-G, Yan R-M, Liang L, Zhong M, Yu Y-H, et al. Cervical squamous cell carcinoma-secreted exosomal miR-221-3p promotes lymphangiogenesis and lymphatic metastasis by targeting VASH1. Oncogene. 2019;38(8):1256–1268. doi: 10.1038/s41388-018-0511-x.
- Ma Z, Wei K, Yang F, Guo Z, Pan C, He Y, Wang J, Li Z, Chen L, Chen Y, et al. Tumor-derived exosomal miR-3157-3p promotes angiogenesis, vascular permeability and metastasis by targeting TIMP/KLF2 in non-small cell lung cancer. Cell Death Dis. 2021;12(9):840. doi: 10.1038/s41419-021-04037-4.
- Wang H, Wang L, Zhou X, Luo X, Liu K, Jiang E, Chen Y, Shao Z, Shang Z. OSCC exosomes regulate miR-210-3p targeting EFNA3 to promote oral cancer angiogenesis through the PI3K/AKT pathway. BioMed Res Int. 2020;2020:1–13. doi: 10.1155/2020/2125656.
- Muhsin-Sharafaldine MR, Saunderson SC, Dunn AC, Faed JM, Kleffmann T, McLellan AD. Procoagulant and immunogenic properties of melanoma exosomes, microvesicles and apoptotic vesicles. Oncotarget. 2016;7(35):56279–56294. doi: 10.18632/oncotarget.10783.
- Li Y, Chen ZK, Duan X, Zhang H-J, Xiao B-L, Wang K-M, Chen G. Targeted inhibition of tumor-derived exosomes as a novel therapeutic option for cancer. Experiment Molecul Med. 2022;54(9):1379–1389. doi: 10.1038/s12276-022-00856-3.
- Tang Q, Yang S, He G, Zheng H, Zhang S, Liu J, Wei S, Fan Q, Peng X, Li X, et al. Tumor-derived exosomes in the cancer immune microenvironment and cancer immunotherapy. Cancer Lett. 2022;548:215823. doi: 10.1016/j.canlet.2022.215823.
- Wolfers J, Lozier A, Raposo G, Regnault A, Théry C, Masurier C, Flament C, Pouzieux S, Faure F, Tursz T, et al. Tumor-derived exosomes are a source of shared tumor rejection antigens for CTL cross-priming. Nat Med. 2001;7(3):297–303. doi: 10.1038/85438.
- Li M, Xu H, Qi Y, Pan Z, Li B, Gao Z, Zhao R, Xue H, Li G. Tumor-derived exosomes deliver the tumor suppressor miR-3591-3p to induce M2 macrophage polarization and promote glioma progression. Oncogene. 2022;41(41):4618–4632. doi: 10.1038/s41388-022-02457-w.
- Chen G, Huang AC, Zhang W, Zhang G, Wu M, Xu W, Yu Z, Yang J, Wang B, Sun H, et al. Exosomal PD-L1 contributes to immunosuppression and is associated with anti-PD-1 response. Nature. 2018;560(7718):382–386. doi: 10.1038/s41586-018-0392-8.
- Rezaei R, Baghaei K, Hashemi SM, Zali MR, Ghanbarian H, Amani D. Tumor-derived exosomes enriched by miRNA-124 promote anti-tumor immune response in CT-26 tumor-bearing mice. Front Med. 2021;8:619939. doi: 10.3389/fmed.2021.619939.
- Altieri SL, Khan ANH, Tomasi TB. Exosomes from plasmacytoma cells as a tumor vaccine. J Immunother. 2004;27(4):282–288. doi: 10.1097/00002371-200407000-00004.
- Cheng L, Wang Y, Huang L. Exosomes from M1-polarized macrophages potentiate the cancer vaccine by creating a pro-inflammatory microenvironment in the lymph node. Mol Ther. 2017;25(7):1665–1675. doi: 10.1016/j.ymthe.2017.02.007.
- Vitale I, Manic G, Coussens LM, Kroemer G, Galluzzi L. Macrophages and metabolism in the tumor microenvironment. Cell Metab. 2019;30(1):36–50. doi: 10.1016/j.cmet.2019.06.001.
- Xu Z, Chen Y, Ma L, Chen Y, Liu J, Guo Y, Yu T, Zhang L, Zhu L, Shu Y, et al. Role of exosomal non-coding RNAs from tumor cells and tumor-associated macrophages in the tumor microenvironment. Mol Ther. 2022;30(10):3133–3154. doi: 10.1016/j.ymthe.2022.01.046.
- Jiang Z, Zhang Y, Zhang Y, Jia Z, Zhang Z, Yang J. Cancer derived exosomes induce macrophages immunosuppressive polarization to promote bladder cancer progression. Cell Commun Signal. 2021;19(1):93. doi: 10.1186/s12964-021-00768-1.
- Chen J, Zhang K, Zhi Y, Wu Y, Chen B, Bai J, Wang X. Tumor-derived exosomal miR-19b-3p facilitates M2 macrophage polarization and exosomal LINC00273 secretion to promote lung adenocarcinoma metastasis via hippo pathway. Clin Transl Med. 2021;11(9):e478. doi: 10.1002/ctm2.478.
- Zhao S, Mi Y, Guan B, Zheng B, Wei P, Gu Y, Zhang Z, Cai S, Xu Y, Li X, et al. Tumor-derived exosomal miR-934 induces macrophage M2 polarization to promote liver metastasis of colorectal cancer. J Hematol Oncol. 2020;13(1):156. doi: 10.1186/s13045-020-00991-2.
- Chen Q, Li Y, Gao W, Chen L, Xu W, Zhu X. Exosome-mediated crosstalk between tumor and tumor-associated macrophages. Front Mol Biosci. 2021;8:764222. doi: 10.3389/fmolb.2021.764222.
- Xiang X, Poliakov A, Liu C, Liu Y, Deng Z-B, Wang J, Cheng Z, Shah SV, Wang G-J, Zhang L, et al. Induction of myeloid-derived suppressor cells by tumor exosomes. Int J Cancer. 2009;124(11):2621–2633. doi: 10.1002/ijc.24249.
- Liu Y, Xiang X, Zhuang X, Zhang S, Liu C, Cheng Z, Michalek S, Grizzle W, Zhang HG. Contribution of MyD88 to the tumor exosome-mediated induction of myeloid derived suppressor cells. Am J Pathol. 2010;176(5):2490–2499. doi: 10.2353/ajpath.2010.090777.
- Qiu W, Guo X, Li B, Wang J, Qi Y, Chen Z, Zhao R, Deng L, Qian M, Wang S, et al. Exosomal miR-1246 from glioma patient body fluids drives the differentiation and activation of myeloid-derived suppressor cells. Mol Ther. 2021;29(12):3449–3464. doi: 10.1016/j.ymthe.2021.06.023.
- Guo X, Qiu W, Wang J, Liu Q, Qian M, Wang S, Zhang Z, Gao X, Chen Z, Guo Q, et al. Glioma exosomes mediate the expansion and function of myeloid-derived suppressor cells through microRNA-29a/Hbp1 and microRNA-92a/Prkar1a pathways. Int J Cancer. 2019;144(12):3111–3126. doi: 10.1002/ijc.32052.
- Shokati E, Safari E. The immunomodulatory role of exosomal microRNA networks in the crosstalk between tumor-associated myeloid-derived suppressor cells and tumor cells. Int Immunopharmacol. 2023;120:110267. doi: 10.1016/j.intimp.2023.110267.
- Ma F, Vayalil J, Lee G, Wang Y, Peng G. Emerging role of tumor-derived extracellular vesicles in T cell suppression and dysfunction in the tumor microenvironment. J Immunother Cancer. 2021;9(10):e003217. doi: 10.1136/jitc-2021-003217.
- Rong L, Li R, Li S, Luo R. Immunosuppression of breast cancer cells mediated by transforming growth factor-β in exosomes from cancer cells. Oncol Lett. 2016;11(1):500–504. doi: 10.3892/ol.2015.3841.
- Muz B, de la Puente P, Azab F, Azab AK. The role of hypoxia in cancer progression, angiogenesis, metastasis, and resistance to therapy. Hypoxia (Auckl). 2015;3:83–92. doi: 10.2147/HP.S93413.
- Shen T, Huang Z, Shi C, Pu X, Xu X, Wu Z, Ding G, Cao L. Pancreatic cancer-derived exosomes induce apoptosis of T lymphocytes through the p38 MAPK-mediated endoplasmic reticulum stress. FASEB J. 2020;34(6):8442–8458. doi: 10.1096/fj.201902186R.
- Salimu J, Webber J, Gurney M, Al-Taei S, Clayton A, Tabi Z. Dominant immunosuppression of dendritic cell function by prostate-cancer-derived exosomes. J Extracell Vesicles. 2017;6(1):1368823. doi: 10.1080/20013078.2017.1368823.
- Tucci M, Mannavola F, Passarelli A, Stucci LS, Cives M, Silvestris F. Exosomes in melanoma: a role in tumor progression, metastasis and impaired immune system activity. Oncotarget. 2018;9(29):20826–20837. doi: 10.18632/oncotarget.24846.
- Batista IA, Quintas ST, Melo SA. The interplay of exosomes and NK cells in cancer biology. Cancers Basel. 2021;13(3):473. doi: 10.3390/cancers13030473.
- Zhao J, Schlößer HA, Wang Z, Qin J, Li J, Popp F, Popp MC, Alakus H, Chon S-H, Hansen HP, et al. Tumor-derived extracellular vesicles inhibit natural killer cell function in pancreatic cancer. Cancers Basel. 2019;11(6):874. doi: 10.3390/cancers11060874.
- Liu C, Yu S, Zinn K, Wang J, Zhang L, Jia Y, Kappes JC, Barnes S, Kimberly RP, Grizzle WE, et al. Murine mammary carcinoma exosomes promote tumor growth by suppression of NK cell function. J Immunol. 2006;176(3):1375–1385. doi: 10.4049/jimmunol.176.3.1375.
- Hong CS, Sharma P, Yerneni SS, Simms P, Jackson EK, Whiteside TL, Boyiadzis M. Circulating exosomes carrying an immunosuppressive cargo interfere with cellular immunotherapy in acute myeloid leukemia. Sci Rep. 2017;7(1):14684. doi: 10.1038/s41598-017-14661-w.
- Zhu X, Qin X, Wang X, Wang Y, Cao W, Zhang J, Chen W, Zhu X, Qin X, Wang X, et al. Oral cancer cell‑derived exosomes modulate natural killer cell activity by regulating the receptors on these cells. Int J Mol Med. 2020;46(6):2115–2125. doi: 10.3892/ijmm.2020.4736.
- Chen JH, Xiang JY, Ding GP, Cao LP. Cholangiocarcinoma-derived exosomes inhibit the antitumor activity of cytokine-induced killer cells by down-regulating the secretion of tumor necrosis factor-α and perforin. J Zhejiang Univ Sci B. 2016;17(7):537–544. doi: 10.1631/jzus.B1500266.
- Mincheva-Nilsson L, Baranov V. Cancer exosomes and NKG2D receptor-ligand interactions: impairing NKG2D-mediated cytotoxicity and anti-tumour immune surveillance. Semin Cancer Biol. 2014;28:24–30. doi: 10.1016/j.semcancer.2014.02.010.
- Cao Y, Wang X, Jin T, Tian Y, Dai C, Widarma C, Song R, Xu F. Immune checkpoint molecules in natural killer cells as potential targets for cancer immunotherapy. Signal Transduct Target Ther. 2020;5(1):250. doi: 10.1038/s41392-020-00348-8.
- Malla RR, Shailender G, Kamal MA. Exosomes: critical mediators of tumour microenvironment reprogramming. Curr Med Chem. 2021;28(39):8182–8202. doi: 10.2174/0929867328666201217105529.
- Zaoui K, Rajadurai CV, Duhamel S, Park M. Arf6 regulates RhoB subcellular localization to control cancer cell invasion. J Cell Biol. 2019;218(11):3812–3826. doi: 10.1083/jcb.201806111.
- Morrissey SM, Zhang F, Ding C, Montoya-Durango DE, Hu X, Yang C, Wang Z, Yuan F, Fox M, Zhang H-G, et al. Tumor-derived exosomes drive immunosuppressive macrophages in a pre-metastatic niche through glycolytic dominant metabolic reprogramming. Cell Metab. 2021;33(10):2040–2058.e10. doi: 10.1016/j.cmet.2021.09.002.
- Li X, Peng X, Zhang C, Bai X, Li Y, Chen G, Guo H, He W, Zhou X, Gou X, et al. Bladder cancer-derived small extracellular vesicles promote tumor angiogenesis by inducing HBP-related metabolic reprogramming and SerRS O-GlcNAcylation in endothelial cells. Adv Sci. 2022;9(30):e2202993. doi: 10.1002/advs.202202993.
- Kucharzewska P, Christianson HC, Welch JE, Svensson KJ, Fredlund E, Ringnér M, Mörgelin M, Bourseau-Guilmain E, Bengzon J, Belting M, et al. Exosomes reflect the hypoxic status of glioma cells and mediate hypoxia-dependent activation of vascular cells during tumor development. Proc Natl Acad Sci U S A. 2013;110(18):7312–7317. doi: 10.1073/pnas.1220998110.
- Wang X, Zhang H, Yang H, Bai M, Ning T, Deng T, Liu R, Fan Q, Zhu K, Li J, et al. Exosome-delivered circRNA promotes glycolysis to induce chemoresistance through the miR-122-PKM2 axis in colorectal cancer. Mol Oncol. 2020;14(3):539–555. doi: 10.1002/1878-0261.12629.
- Faict S, Oudaert I, D’Auria L, Dehairs J, Maes K, Vlummens P, De Veirman K, De Bruyne E, Fostier K, Vande Broek I, et al. The transfer of sphingomyelinase contributes to drug resistance in multiple myeloma. Cancers Basel. 2019;11(12):1823. doi: 10.3390/cancers11121823.
- Wei Y, Wang D, Jin F, Bian Z, Li L, Liang H, Li M, Shi L, Pan C, Zhu D, et al. Pyruvate kinase type M2 promotes tumour cell exosome release via phosphorylating synaptosome-associated protein 23. Nat Commun. 2017;8(1):14041. doi: 10.1038/ncomms14041.
- Dai J, Su Y, Zhong S, Cong L, Liu B, Yang J, Tao Y, He Z, Chen C, Jiang Y, et al. Exosomes: key players in cancer and potential therapeutic strategy. Signal Transduct Target Ther. 2020;5(1):145. doi: 10.1038/s41392-020-00261-0.
- Pitt JM, Marabelle A, Eggermont A, Soria JC, Kroemer G, Zitvogel L. Targeting the tumor microenvironment: removing obstruction to anticancer immune responses and immunotherapy. Ann Oncol. 2016;27(8):1482–1492. doi: 10.1093/annonc/mdw168.
- Meng L, Cheng Y, Tong X, Gan S, Ding Y, Zhang Y, Wang C, Xu L, Zhu Y, Wu J, et al. Tumor oxygenation and hypoxia inducible Factor-1 functional inhibition via a reactive oxygen species responsive nanoplatform for enhancing radiation therapy and abscopal effects. Acs Nano. 2018;12(8):8308–8322. doi: 10.1021/acsnano.8b03590.
- Barker HE, Paget JTE, Khan AA, Harrington KJ. The tumour microenvironment after radiotherapy: mechanisms of resistance and recurrence. Nat Rev Cancer. 2015;15(7):409–425. doi: 10.1038/nrc3958.
- Zhang L, Du X, Li Q, Qian L, Chen J, Liu C, Yu Q, Gan Z. A multimodal therapeutic nanoplatform overcoming tumor hypoxia heterogeneity for improved tumor chemoradiotherapy. Adv Funct Mater. 2022;32(34):2204629. doi: 10.1002/adfm.202204629.
- Xiao L, Chen B, Wang W, Tian T, Qian H, Li X, Yu Y. Multifunctional Au@AgBiS2 nanoparticles as high-efficiency radiosensitizers to induce pyroptosis for cancer radioimmunotherapy. Adv Sci (Weinh). 2023 Sep 8;10(30):e2302141. 10.1002/advs.202302141.
- Ma X, Yao M, Gao Y, Yue Y, Li Y, Zhang T, Nie G, Zhao X, Liang X. Functional immune cell-derived exosomes engineered for the trilogy of radiotherapy sensitization. Adv Sci. 2022;9(23):e2106031. doi: 10.1002/advs.202106031.
- Ashton TM, McKenna WG, Kunz-Schughart LA, Higgins GS. Oxidative phosphorylation as an emerging target in cancer therapy. Clin Cancer Res. 2018;24(11):2482–2490. doi: 10.1158/1078-0432.CCR-17-3070.
- Skwarski M, McGowan DR, Belcher E, Di Chiara F, Stavroulias D, McCole M, Derham JL, Chu K-Y, Teoh E, Chauhan J, et al. Mitochondrial inhibitor atovaquone increases tumor oxygenation and inhibits hypoxic gene expression in patients with non–small cell lung cancer. Clin Cancer Res. 2021;27(9):2459–2469. doi: 10.1158/1078-0432.CCR-20-4128.
- Benej M, Hong X, Vibhute S, Scott S, Wu J, Graves E, Le Q-T, Koong AC, Giaccia AJ, Yu B, et al. Papaverine and its derivatives radiosensitize solid tumors by inhibiting mitochondrial metabolism. Proc Natl Acad Sci U S A. 2018;115(42):10756–10761. doi: 10.1073/pnas.1808945115.
- Wu P, Zhou Q, Zhu H, Zhuang Y, Bao J. Enhanced antitumor efficacy in colon cancer using EGF functionalized PLGA nanoparticles loaded with 5-fluorouracil and perfluorocarbon. BMC Cancer. 2020;20(1):354. doi: 10.1186/s12885-020-06803-7.
- Zou MZ, Liu WL, Li CX, Zheng D-W, Zeng J-Y, Gao F, Ye J-J, Zhang X-Z. A multifunctional biomimetic nanoplatform for relieving hypoxia to enhance chemotherapy and inhibit the PD-1/PD-L1 axis. Small. 2018;14(28):e1801120. doi: 10.1002/smll.201801120.
- Zhong T, Gao N, Guan Y, Liu Z, Guan J. Co-delivery of bioengineered exosomes and oxygen for treating critical limb ischemia in diabetic mice. Acs Nano. 2023;17(24):25157–25174. doi: 10.1021/acsnano.3c08088.
- Huang J, Yu P, Liao M, Dong X, Xu J, Ming J, Bin D, Wang Y, Zhang F, Xia Y, et al. 2023. A self-charging salt water battery for antitumor therapy. Sci Adv. 9(13):eadf3992. doi: 10.1126/sciadv.adf3992.
- Zhang H, Shi C, Han F, Li M, Ma H, Sui R, Long S, Sun W, Du J, Fan J, et al. Precise gliomas therapy: hypoxia-activated prodrugs sensitized by nano-photosensitizers. Biomater. 2022;289:121770. doi: 10.1016/j.biomaterials.2022.121770.
- Rini BI, Pal SK, Escudier BJ, Atkins MB, Hutson TE, Porta C, Verzoni E, Needle MN, McDermott DF. Tivozanib versus sorafenib in patients with advanced renal cell carcinoma (TIVO-3): a phase 3, multicentre, randomised, controlled, open-label study. Lancet Oncol. 2020;21(1):95–104. doi: 10.1016/S1470-2045(19)30735-1.
- Chen W, Hill H, Christie A, Kim MS, Holloman E, Pavia-Jimenez A, Homayoun F, Ma Y, Patel N, Yell P, et al. Targeting renal cell carcinoma with a HIF-2 antagonist. Nature. 2016;539(7627):112–117. doi: 10.1038/nature19796.
- Jonasch E, Donskov F, Iliopoulos O, Rathmell WK, Narayan VK, Maughan B, Oudard S, Else T, Maranchie JK, Welsh SJ, et al. Belzutifan for renal cell carcinoma in von Hippel–Lindau disease. N Engl J Med. 2021;385(22):2036–2046. doi: 10.1056/NEJMoa2103425.
- Chen Z, Han F, Du Y, Shi H, Zhou W. Hypoxic microenvironment in cancer: molecular mechanisms and therapeutic interventions. Signal Transduct Target Ther. 2023;8(1):70. doi: 10.1038/s41392-023-01332-8.
- Yuan X, Ruan W, Bobrow B, Carmeliet P, Eltzschig HK. Targeting hypoxia-inducible factors: therapeutic opportunities and challenges. Nat Rev Drug Discov. 2023 Dec 20;23(3):175–200. doi:10.1038/s41573-023-00848-6.
- Dimik M, Abeysinghe P, Logan J, Mitchell M. The exosome: a review of current therapeutic roles and capabilities in human reproduction. Drug Deliv Transl Res. 2023;13(2):473–502. doi: 10.1007/s13346-022-01225-3.
- Sulea T, Rohani N, Baardsnes J, Corbeil CR, Deprez C, Cepero-Donates Y, Robert A, Schrag JD, Parat M, Duchesne M, et al. Structure-based engineering of pH-dependent antibody binding for selective targeting of solid-tumor microenvironment. Mabs-austin. 2020;12(1):1682866. doi: 10.1080/19420862.2019.1682866.
- Liu Y, Lee AG, Nguyen AW, Maynard JA. An antibody Fc engineered for conditional antibody-dependent cellular cytotoxicity at the low tumor microenvironment pH. J Biol Chem. 2022;298(4):101798. doi: 10.1016/j.jbc.2022.101798.
- Li Y, Liu J, Chen W, Wang W, Yang F, Liu X, Sheng Y, Du K, He M, Lyu X, et al. A pH-dependent anti-CD47 antibody that selectively targets solid tumors and improves therapeutic efficacy and safety. J Hematol Oncol. 2023;16(1):2. doi: 10.1186/s13045-023-01399-4.
- Su Z, Dong S, Chen Y, Huang T, Qin B, Yang Q, Jiang X, Zou C. Microfluidics-enabled nanovesicle delivers CD47/PD-L1 antibodies to enhance antitumor immunity and reduce immunotoxicity in lung adenocarcinoma. Adv Sci (Weinh). 2023;10(20):e2206213. doi: 10.1002/advs.202206213.
- Zhang W, He Q, Lopez B, Hu J, Kundu A, Andraza MC, Kerner AR, Schreiber GH, Shepard HM, Frost GI, et al. Abstract PO074: Logic-gating HER2 CAR-T to the tumor microenvironment mitigates on-target, off-tumor toxicity without compromising cytotoxicity against HER2-over-expressing tumors. Cancer Immunol Res. 2021;9(2_Supplement):PO074–PO074. doi: 10.1158/2326-6074.TUMIMM20-PO074.
- Corbet C, Feron O. Tumour acidosis: from the passenger to the driver’s seat. Nat Rev Cancer. 2017;17(10):577–593. doi: 10.1038/nrc.2017.77.
- Renner K, Bruss C, Schnell A, Koehl G, Becker HM, Fante M, Menevse A-N, Kauer N, Blazquez R, Hacker L, et al. Restricting glycolysis preserves T cell effector functions and augments checkpoint therapy. Cell Rep. 2019;29(1):135–150.e9. doi: 10.1016/j.celrep.2019.08.068.
- Cappellesso F, Orban MP, Shirgaonkar N, Berardi E, Serneels J, Neveu M-A, Di Molfetta D, Piccapane F, Caroppo R, Debellis L, et al. Targeting the bicarbonate transporter SLC4A4 overcomes immunosuppression and immunotherapy resistance in pancreatic cancer. Nat Cancer. 2022;3(12):1464–1483. doi: 10.1038/s43018-022-00470-2.
- Yang X, Xu C, Zhang X, Li P, Sun F, Liu X, Wang X, Kwok RTK, Yang J, Lam JWY, et al. Development of sulfonamide‐functionalized charge‐reversal AIE photosensitizers for precise photodynamic therapy in the acidic tumor microenvironment. Adv Funct Mater. 2023;33(30):2300746. doi: 10.1002/adfm.202300746.
- Botha H, Farah CS, Koo K, Cirillo N, McCullough M, Paolini R, Celentano A. The role of glucose transporters in oral squamous cell carcinoma. Biomolecules. 2021;11(8):1070. doi: 10.3390/biom11081070.
- Cao J, Cui S, Li S, Du C, Tian J, Wan S, Qian Z, Gu Y, Chen WR, Wang G, et al. Targeted cancer therapy with a 2-deoxyglucose–based adriamycin complex. Cancer Res. 2013;73(4):1362–1373. doi: 10.1158/0008-5472.CAN-12-2072.
- Sztandera K, Działak P, Marcinkowska M, Stańczyk M, Gorzkiewicz M, Janaszewska A, Klajnert-Maculewicz B. Sugar modification enhances cytotoxic activity of PAMAM-doxorubicin conjugate in glucose-deprived MCF-7 cells – possible role of GLUT1 transporter. Pharm Res. 2019;36(10):140. doi: 10.1007/s11095-019-2673-9.
- Lin YS, Tungpradit R, Sinchaikul S, An FM, Liu DZ, Phutrakul S, Chen ST. Targeting the delivery of glycan-based paclitaxel prodrugs to cancer cells via glucose transporters. J Med Chem. 2008;51(23):7428–7441. doi: 10.1021/jm8006257.
- Liu P, Lu Y, Gao X, Liu R, Zhang-Negrerie D, Shi Y, Wang Y, Wang S, Gao Q. Highly water-soluble platinum(II) complexes as GLUT substrates for targeted therapy: improved anticancer efficacy and transporter-mediated cytotoxic properties. Chem Commun (Camb). 2013;49(24):2421–2423. doi: 10.1039/c3cc38589b.
- Wang H, Yang X, Zhao C, Wang PG, Wang X. Glucose-conjugated platinum(IV) complexes as tumor-targeting agents: design, synthesis and biological evaluation. Bioorg Med Chem. 2019;27(8):1639–1645. doi: 10.1016/j.bmc.2019.03.006.
- Chan DA, Sutphin PD, Nguyen P, Turcotte S, Lai EW, Banh A, Reynolds GE, Chi J-T, Wu J, Solow-Cordero DE, et al. Targeting GLUT1 and the Warburg effect in renal cell carcinoma by chemical synthetic lethality. Sci Transl Med. 2011;3(94):94ra70. doi: 10.1126/scitranslmed.3002394.
- Adams DJ, Ito D, Rees MG, Seashore-Ludlow B, Puyang X, Ramos AH, Cheah JH, Clemons PA, Warmuth M, Zhu P, et al. NAMPT is the cellular target of STF-31-like small-molecule probes. ACS Chem Biol. 2014;9(10):2247–2254. doi: 10.1021/cb500347p.
- Reckzeh ES, Karageorgis G, Schwalfenberg M, Ceballos J, Nowacki J, Stroet MCM, Binici A, Knauer L, Brand S, Choidas A, et al. Inhibition of glucose transporters and glutaminase synergistically impairs tumor cell growth. Cell Chem Biol. 2019;26(9):1214–1228.e25. doi: 10.1016/j.chembiol.2019.06.005.
- Siebeneicher H, Cleve A, Rehwinkel H, Neuhaus R, Heisler I, Müller T, Bauser M, Buchmann B. Identification and optimization of the first highly selective GLUT1 inhibitor BAY-876. Chem Med. 2016;11(20):2261–2271. doi: 10.1002/cmdc.201600276.
- Patra KC, Wang Q, Bhaskar PT, Miller L, Wang Z, Wheaton W, Chandel N, Laakso M, Muller W, Allen E, et al. Hexokinase 2 is required for tumor initiation and maintenance and its systemic deletion is therapeutic in mouse models of cancer. Cancer Cell. 2013;24(2):213–228. doi: 10.1016/j.ccr.2013.06.014.
- Ganapathy-Kanniappan S, Geschwind JFH, Kunjithapatham R, Buijs M, Vossen JA, Tchernyshyov I, Cole RN, Syed LH, Rao PP, Ota S, et al. Glyceraldehyde-3-phosphate dehydrogenase (GAPDH) is pyruvylated during 3-bromopyruvate mediated cancer cell death. Anticancer Res. 2009;29(12):4909–4918.
- Lin H, Zeng J, Xie R, Schulz MJ, Tedesco R, Qu J, Erhard KF, Mack JF, Raha K, Rendina AR, et al. Discovery of a novel 2,6-disubstituted glucosamine series of potent and selective hexokinase 2 inhibitors. ACS Med Chem Lett. 2016;7(3):217–222. doi: 10.1021/acsmedchemlett.5b00214.
- Suzuki A, Puri S, Leland P, Puri A, Moudgil T, Fox BA, Puri RK, Joshi BH. Subcellular compartmentalization of PKM2 identifies anti-PKM2 therapy response in vitro and in vivo mouse model of human non-small-cell lung cancer. PLoS One. 2019;14(5):e0217131. doi: 10.1371/journal.pone.0217131.
- Xie H, Hanai JI, Ren JG, Kats L, Burgess K, Bhargava P, Signoretti S, Billiard J, Duffy K, Grant A, et al. Targeting lactate dehydrogenase–a inhibits tumorigenesis and tumor progression in mouse models of lung cancer and impacts tumor-initiating cells. Cell Metabol. 2014;19(5):795–809. doi: 10.1016/j.cmet.2014.03.003.
- Rajeshkumar NV, Dutta P, Yabuuchi S, de Wilde RF, Martinez GV, Le A, Kamphorst JJ, Rabinowitz JD, Jain SK, Hidalgo M, et al. Therapeutic targeting of the Warburg effect in pancreatic cancer relies on an absence of p53 function. Cancer Res. 2015;75(16):3355–3364. doi: 10.1158/0008-5472.CAN-15-0108.
- Zhang SL, He Y, Tam KY. Targeting cancer metabolism to develop human lactate dehydrogenase (hLDH)5 inhibitors. Drug Discov Today. 2018;23(7):1407–1415. doi: 10.1016/j.drudis.2018.05.014.
- Billiard J, Dennison JB, Briand J, Annan RS, Chai D, Colón M, Dodson CS, Gilbert SA, Greshock J, Jing J, et al. Quinoline 3-sulfonamides inhibit lactate dehydrogenase a and reverse aerobic glycolysis in cancer cells. Cancer Metab. 2013;1(1):19. doi: 10.1186/2049-3002-1-19.
- Wang N, Jiang X, Zhang S, Zhu A, Yuan Y, Xu H, Lei J, Yan C. Structural basis of human monocarboxylate transporter 1 inhibition by anti-cancer drug candidates. Cell. 2021;184(2):370–383.e13. doi: 10.1016/j.cell.2020.11.043.
- Guan X, Morris ME. In vitro and in vivo efficacy of AZD3965 and Alpha-Cyano-4-Hydroxycinnamic acid in the murine 4T1 breast tumor model. Aaps J. 2020;22(4):84. doi: 10.1208/s12248-020-00466-9.
- Edwards DN, Ngwa VM, Raybuck AL, Wang S, Hwang Y, Kim LC, Cho SH, Paik Y, Wang Q, Zhang S, et al. Selective glutamine metabolism inhibition in tumor cells improves antitumor T lymphocyte activity in triple-negative breast cancer. J Clin Invest. 2021;131(4):e140100. doi: 10.1172/JCI140100.
- Jin H, Wang S, Zaal EA, Wang C, Wu H, Bosma A, Jochems F, Isima N, Jin G, Lieftink C, et al. A powerful drug combination strategy targeting glutamine addiction for the treatment of human liver cancer. Elife. 2020;9:e56749. doi: 10.7554/eLife.56749.
- Najumudeen AK, Ceteci F, Fey SK, Hamm G, Steven RT, Hall H, Nikula CJ, Dexter A, Murta T, Race AM, et al. The amino acid transporter SLC7A5 is required for efficient growth of KRAS-mutant colorectal cancer. Nat Genet. 2021;53(1):16–26. doi: 10.1038/s41588-020-00753-3.
- Nguyen Cao TG, Truong Hoang Q, Kang JH, Kang SJ, Ravichandran V, Rhee WJ, Lee M, Ko YT, Shim MS. Bioreducible exosomes encapsulating glycolysis inhibitors potentiate mitochondria-targeted sonodynamic cancer therapy via cancer-targeted drug release and cellular energy depletion. Biomater. 2023;301:122242. doi: 10.1016/j.biomaterials.2023.122242.
- Tang T, Huang X, Zhang G, Hong Z, Bai X, Liang T. Advantages of targeting the tumor immune microenvironment over blocking immune checkpoint in cancer immunotherapy. Signal Transduct Target Ther. 2021;6(1):72. doi: 10.1038/s41392-020-00449-4.
- Liu L, Li H, Wang J, Zhang J, Liang XJ, Guo W, Gu Z. Leveraging macrophages for cancer theranostics. Adv Drug Deliv Rev. 2022;183:114136. doi: 10.1016/j.addr.2022.114136.
- Anfray A, Ummarino U, Andón A, Allavena A. Current strategies to target tumor-associated-macrophages to improve anti-tumor immune responses. Cells. 2019;9(1):46. doi: 10.3390/cells9010046.
- Beltraminelli T, De Palma M. Biology and therapeutic targeting of tumour-associated macrophages. J Pathol. 2020;250(5):573–592. doi: 10.1002/path.5403.
- Gomez-Roca CA, Italiano A, Le Tourneau C, Cassier PA, Toulmonde M, D’Angelo SP, Campone M, Weber KL, Loirat D, Cannarile MA, et al. Phase I study of emactuzumab single agent or in combination with paclitaxel in patients with advanced/metastatic solid tumors reveals depletion of immunosuppressive M2-like macrophages. Ann Oncol. 2019;30(8):1381–1392. doi: 10.1093/annonc/mdz163.
- Zhu Y, Knolhoff BL, Meyer MA, Nywening TM, West BL, Luo J, Wang-Gillam A, Goedegebuure SP, Linehan DC, DeNardo DG, et al. CSF1/CSF1R blockade reprograms tumor-infiltrating macrophages and improves response to T-cell checkpoint immunotherapy in pancreatic cancer models. Cancer Res. 2014;74(18):5057–5069. doi: 10.1158/0008-5472.CAN-13-3723.
- Pradel LP, Ooi CH, Romagnoli S, Cannarile MA, Sade H, Rüttinger D, Ries CH. Macrophage susceptibility to emactuzumab (RG7155) treatment. Mol Cancer Ther. 2016;15(12):3077–3086. doi: 10.1158/1535-7163.MCT-16-0157.
- Cassier PA, Italiano A, Gomez-Roca CA, Le Tourneau C, Toulmonde M, Cannarile MA, Ries C, Brillouet A, Müller C, Jegg A-M, et al. CSF1R inhibition with emactuzumab in locally advanced diffuse-type tenosynovial giant cell tumours of the soft tissue: a dose-escalation and dose-expansion phase 1 study. Lancet Oncol. 2015;16(8):949–956. doi: 10.1016/S1470-2045(15)00132-1.
- Tacke F. Targeting hepatic macrophages to treat liver diseases. J Hepatol. 2017;66(6):1300–1312. doi: 10.1016/j.jhep.2017.02.026.
- Nagarsheth N, Wicha MS, Zou W. Chemokines in the cancer microenvironment and their relevance in cancer immunotherapy. Nat Rev Immunol. 2017;17(9):559–572. doi: 10.1038/nri.2017.49.
- Schmall A, Al-Tamari HM, Herold S, Kampschulte M, Weigert A, Wietelmann A, Vipotnik N, Grimminger F, Seeger W, Pullamsetti SS, et al. Macrophage and cancer cell cross-talk via CCR2 and CX3CR1 is a fundamental mechanism driving lung cancer. Am J Respir Crit Care Med. 2015;191(4):437–447. doi: 10.1164/rccm.201406-1137OC.
- Bonapace L, Coissieux MM, Wyckoff J, Mertz KD, Varga Z, Junt T, Bentires-Alj M. Cessation of CCL2 inhibition accelerates breast cancer metastasis by promoting angiogenesis. Nature. 2014;515(7525):130–133. doi: 10.1038/nature13862.
- Yoon J, Le XT, Kim J, Lee H, Nguyen NT, Lee WT, Lee ES, Oh KT, Choi H-G, Youn YS, et al. Macrophage-reprogramming upconverting nanoparticles for enhanced TAM-mediated antitumor therapy of hypoxic breast cancer. J Control Release. 2023;360:482–495. doi: 10.1016/j.jconrel.2023.07.009.
- Huang L, Xu R, Li W, Lv L, Lin C, Yang X, Yao Y, Saw PE, Xu X. Repolarization of macrophages to improve sorafenib sensitivity for combination cancer therapy. Acta Biomater. 2023;162:98–109. doi: 10.1016/j.actbio.2023.03.014.
- Cassetta L, Kitamura T. Macrophage targeting: opening new possibilities for cancer immunotherapy. Immunol. 2018;155(3):285–293. doi: 10.1111/imm.12976.
- Liu H, Zhao D, Li H, Zhang W, Lin Q, Wang X, Zheng S, Zhang L, Li L, Hu S, et al. Blocking iASPP/Nrf2/M-CSF axis improves anti-cancer effect of chemotherapy-induced senescence by attenuating M2 polarization. Cell Death Disease. 2022;13(2):166. doi: 10.1038/s41419-022-04611-4.
- Myers KV, Amend SR, Pienta KJ. Targeting Tyro3, Axl and MerTK (TAM receptors): implications for macrophages in the tumor microenvironment. Mol Cancer. 2019;18(1):94. doi: 10.1186/s12943-019-1022-2.
- De Cicco P, Ercolano G, Ianaro A. The new era of cancer immunotherapy: targeting Myeloid-Derived suppressor cells to overcome immune evasion. Front Immunol. 2020;11:1680. doi: 10.3389/fimmu.2020.01680.
- Bronte V, Serafini P, Apolloni E, Zanovello P. Tumor-induced immune dysfunctions caused by myeloid suppressor cells. J Immunother. 2001;24(6):431–446. doi: 10.1097/00002371-200111000-00001.
- Qin H, Lerman B, Sakamaki I, Wei G, Cha SC, Rao SS, Qian J, Hailemichael Y, Nurieva R, Dwyer KC, et al. Generation of a new therapeutic peptide that depletes myeloid-derived suppressor cells in tumor-bearing mice. Nat Med. 2014;20(6):676–681. doi: 10.1038/nm.3560.
- Dang Y, Rutnam ZJ, Dietsch G, Lu H, Yang Y, Hershberg R, Disis ML. TLR8 ligation induces apoptosis of monocytic myeloid-derived suppressor cells. J Leukoc Biol. 2018;103(1):157–164. doi: 10.1002/JLB.5AB0217-070R.
- Eriksson E, Wenthe J, Irenaeus S, Loskog A, Ullenhag G. Gemcitabine reduces MDSCs, tregs and TGFβ-1 while restoring the teff/treg ratio in patients with pancreatic cancer. J Transl Med. 2016;14(1):282. doi: 10.1186/s12967-016-1037-z.
- Kanterman J, Sade-Feldman M, Biton M, Ish-Shalom E, Lasry A, Goldshtein A, Hubert A, Baniyash M. Adverse immunoregulatory effects of 5FU and CPT11 chemotherapy on myeloid-derived suppressor cells and colorectal cancer outcomes. Cancer Res. 2014;74(21):6022–6035. doi: 10.1158/0008-5472.CAN-14-0657.
- Li X, Yao W, Yuan Y, Chen P, Li B, Li J, Chu R, Song H, Xie D, Jiang X, et al. Targeting of tumour-infiltrating macrophages via CCL2/CCR2 signalling as a therapeutic strategy against hepatocellular carcinoma. Gut. 2017;66(1):157–167. doi: 10.1136/gutjnl-2015-310514.
- Umansky V, Blattner C, Gebhardt C, Utikal J. CCR5 in recruitment and activation of myeloid-derived suppressor cells in melanoma. Cancer Immunol Immunother. 2017;66(8):1015–1023. doi: 10.1007/s00262-017-1988-9.
- Blattner C, Fleming V, Weber R, Himmelhan B, Altevogt P, Gebhardt C, Schulze TJ, Razon H, Hawila E, Wildbaum G, et al. CCR5+ Myeloid-derived suppressor cells are enriched and activated in melanoma lesions. Cancer Res. 2018;78(1):157–167. doi: 10.1158/0008-5472.CAN-17-0348.
- Cannarile MA, Weisser M, Jacob W, Jegg AM, Ries CH, Rüttinger D. Colony-stimulating factor 1 receptor (CSF1R) inhibitors in cancer therapy. J Immunother Cancer. 2017;5(1):53. doi: 10.1186/s40425-017-0257-y.
- Law AMK, Valdes-Mora F, Gallego-Ortega D. Myeloid-derived suppressor cells as a therapeutic target for cancer. Cells. 2020;9(3):561. doi: 10.3390/cells9030561.
- Lin S, Wang J, Wang L, Wen J, Guo Y, Qiao W, Zhou J, Xu G, Zhi F. Phosphodiesterase-5 inhibition suppresses colonic inflammation-induced tumorigenesis via blocking the recruitment of MDSC. 2017;Am J Cancer Res. 7(1):41–52.
- Weed DT, Vella JL, Reis IM, De la Fuente AC, Gomez C, Sargi Z, Nazarian R, Califano J, Borrello I, Serafini P, et al. Tadalafil reduces myeloid-derived suppressor cells and regulatory T cells and promotes tumor immunity in patients with head and neck squamous cell carcinoma. Clin Cancer Res. 2015;21(1):39–48. doi: 10.1158/1078-0432.CCR-14-1711.
- Hassel JC, Jiang H, Bender C, Winkler J, Sevko A, Shevchenko I, Halama N, Dimitrakopoulou-Strauss A, Haefeli WE, Jäger D, et al. Tadalafil has biologic activity in human melanoma. Results of a pilot trial with tadalafil in patients with metastatic melanoma (TaMe). Oncoimmunol. 2017;6(9):e1326440. doi: 10.1080/2162402X.2017.1326440.
- Ali K, Soond DR, Pineiro R, Hagemann T, Pearce W, Lim EL, Bouabe H, Scudamore CL, Hancox T, Maecker H, et al. Inactivation of PI(3)K p110δ breaks regulatory T-cell-mediated immune tolerance to cancer. Nature. 2014;510(7505):407–411. doi: 10.1038/nature13444.
- Christmas BJ, Rafie CI, Hopkins AC, Scott BA, Ma HS, Cruz KA, Woolman S, Armstrong TD, Connolly RM, Azad NA, et al. Entinostat converts immune-resistant breast and pancreatic cancers into checkpoint-responsive tumors by reprogramming tumor-infiltrating MDSCs. Cancer Immunol Res. 2018;6(12):1561–1577. doi: 10.1158/2326-6066.CIR-18-0070.
- Tcyganov E, Mastio J, Chen E, Gabrilovich DI. Plasticity of myeloid-derived suppressor cells in cancer. Curr Opin Immunol. 2018;51:76–82. doi: 10.1016/j.coi.2018.03.009.
- Iclozan C, Antonia S, Chiappori A, Chen DT, Gabrilovich D. Therapeutic regulation of myeloid-derived suppressor cells and immune response to cancer vaccine in patients with extensive stage small cell lung cancer. Cancer Immunol Immunother. 2013;62(5):909–918. doi: 10.1007/s00262-013-1396-8.
- Lathers DMR, Clark JI, Achille NJ, Young MRI. Phase 1B study to improve immune responses in head and neck cancer patients using escalating doses of 25-hydroxyvitamin D3. Cancer Immunol Immunother. 2004;53(5):422–430. doi: 10.1007/s00262-003-0459-7.
- Fultang L, Panetti S, Ng M, Collins P, Graef S, Rizkalla N, Booth S, Lenton R, Noyvert B, Shannon-Lowe C, et al. MDSC targeting with gemtuzumab ozogamicin restores T cell immunity and immunotherapy against cancers. EBioMedicine. 2019;47:235–246. doi: 10.1016/j.ebiom.2019.08.025.
- Alshetaiwi H, Pervolarakis N, McIntyre LL, Ma D, Nguyen Q, Rath JA, Nee K, Hernandez G, Evans K, Torosian L, et al. Defining the emergence of myeloid-derived suppressor cells in breast cancer using single-cell transcriptomics. Sci Immunol. 2020;5(44):eaay6017. doi: 10.1126/sciimmunol.aay6017.
- Alissafi T, Hatzioannou A, Mintzas K, Barouni RM, Banos A, Sormendi S, Polyzos A, Xilouri M, Wielockx B, Gogas H, et al. Autophagy orchestrates the regulatory program of tumor-associated myeloid-derived suppressor cells. J Clin Invest. 2018;128(9):3840–3852. doi: 10.1172/JCI120888.
- Boettcher M, McManus MT. Choosing the Right Tool for the Job: RNAi, TALEN, or CRISPR. Mol Cell. 2015;58(4):575–585. doi: 10.1016/j.molcel.2015.04.028.
- Colombo M, Moita C, van Niel G, Kowal J, Vigneron J, Benaroch P, Manel N, Moita LF, Théry C, Raposo G, et al. Analysis of ESCRT functions in exosome biogenesis, composition and secretion highlights the heterogeneity of extracellular vesicles. J Cell Sci. 2013;126(Pt 24):5553–5565. doi: 10.1242/jcs.128868.
- Hoshino D, Kirkbride KC, Costello K, Clark ES, Sinha S, Grega-Larson N, Tyska MJ, Weaver AM. Exosome secretion is enhanced by invadopodia and drives invasive behavior. Cell Rep. 2013;5(5):1159–1168. doi: 10.1016/j.celrep.2013.10.050.
- Ostenfeld MS, Jeppesen DK, Laurberg JR, Boysen AT, Bramsen JB, Primdal-Bengtson B, Hendrix A, Lamy P, Dagnaes-Hansen F, Rasmussen MH, et al. Cellular disposal of miR23b by RAB27-dependent exosome release is linked to acquisition of metastatic properties. Cancer Res. 2014;74(20):5758–5771. doi: 10.1158/0008-5472.CAN-13-3512.
- Sinha S, Hoshino D, Hong NH, Kirkbride KC, Grega-Larson NE, Seiki M, Tyska MJ, Weaver AM. Cortactin promotes exosome secretion by controlling branched actin dynamics. J Cell Biol. 2016;214(2):197–213. doi: 10.1083/jcb.201601025.
- Poggio M, Hu T, Pai CC, Chu B, Belair CD, Chang A, Montabana E, Lang UE, Fu Q, Fong L, et al. Suppression of exosomal PD-L1 induces systemic anti-tumor immunity and memory. Cell. 2019;177(2):414–427.e13. doi: 10.1016/j.cell.2019.02.016.
- Baietti MF, Zhang Z, Mortier E, Melchior A, Degeest G, Geeraerts A, Ivarsson Y, Depoortere F, Coomans C, Vermeiren E, et al. Syndecan–syntenin–ALIX regulates the biogenesis of exosomes. Nat Cell Biol. 2012;14(7):677–685. doi: 10.1038/ncb2502.
- Wei D, Zhan W, Gao Y, Huang L, Gong R, Wang W, Zhang R, Wu Y, Gao S, Kang T, et al. RAB31 marks and controls an ESCRT-independent exosome pathway. Cell Res. 2021;31(2):157–177. doi: 10.1038/s41422-020-00409-1.
- Peak TC, Panigrahi GK, Praharaj PP, Su Y, Shi L, Chyr J, Rivera‐Chávez J, Flores‐Bocanegra L, Singh R, Vander Griend DJ, et al. Syntaxin 6-mediated exosome secretion regulates enzalutamide resistance in prostate cancer. Mol Carcinog. 2020;59(1):62–72. doi: 10.1002/mc.23129.
- Ruiz-Martinez M, Navarro A, Marrades RM, Viñolas N, Santasusagna S, Muñoz C, Ramírez J, Molins L, Monzo M. YKT6 expression, exosome release, and survival in non-small cell lung cancer. Oncotarget. 2016;7(32):51515–51524. doi: 10.18632/oncotarget.9862.
- Chen M, Mao A, Xu M, Weng Q, Mao J, Ji J. CRISPR-Cas9 for cancer therapy: opportunities and challenges. Cancer Lett. 2019;447:48–55. doi: 10.1016/j.canlet.2019.01.017.
- Vakulskas CA, Behlke MA. 2019. Evaluation and reduction of CRISPR off-target cleavage events. Nucleic Acid Ther. 29(4):167–174. doi: 10.1089/nat.2019.0790.
- Behr M, Zhou J, Xu B, Zhang H. 2021. In vivo delivery of CRISPR-Cas9 therapeutics: progress and challenges. Acta Pharm Sin B. 11(8):2150–2171. doi: 10.1016/j.apsb.2021.05.020.
- Yang Y, Li CW, Chan LC, Wei Y, Hsu J-M, Xia W, Cha J-H, Hou J, Hsu JL, Sun L, et al. Exosomal PD-L1 harbors active defense function to suppress T cell killing of breast cancer cells and promote tumor growth. Cell Res. 2018;28(8):862–864. doi: 10.1038/s41422-018-0060-4.
- Montermini L, Meehan B, Garnier D, Lee WJ, Lee TH, Guha A, Al-Nedawi K, Rak J. Inhibition of oncogenic epidermal growth factor receptor kinase triggers release of exosome-like extracellular vesicles and impacts their phosphoprotein and DNA content. J Biol Chem. 2015;290(40):24534–24546. doi: 10.1074/jbc.M115.679217.
- Matsumoto A, Takahashi Y, Nishikawa M, Sano K, Morishita M, Charoenviriyakul C, Saji H, Takakura Y. Accelerated growth of B16BL6 tumor in mice through efficient uptake of their own exosomes by B16BL6 cells. Cancer Sci. 2017;108(9):1803–1810. doi: 10.1111/cas.13310.
- Wang G, Xie L, Li B, Sang W, Yan J, Li J, Tian H, Li W, Zhang Z, Tian Y, et al. A nanounit strategy reverses immune suppression of exosomal PD-L1 and is associated with enhanced ferroptosis. Nat Commun. 2021;12(1):5733. doi: 10.1038/s41467-021-25990-w.
- Airola MV, Shanbhogue P, Shamseddine AA, Guja KE, Senkal CE, Maini R, Bartke N, Wu BX, Obeid LM, Garcia-Diaz M, et al. Structure of human nSmase2 reveals an interdomain allosteric activation mechanism for ceramide generation. Proc Natl Acad Sci U S A. 2017;114(28):E5549–E5558. doi: 10.1073/pnas.1705134114.
- Tschuschke M, Kocherova I, Bryja A, Mozdziak P, Angelova Volponi A, Janowicz K, Sibiak R, Piotrowska-Kempisty H, Iżycki D, Bukowska D, et al. Inclusion biogenesis, methods of isolation and clinical application of human cellular exosomes. J Clin Med. 2020;9(2):436. doi: 10.3390/jcm9020436.
- Yoo SW, Agarwal A, Smith MD, Khuder SS, Baxi EG, Thomas AG, Rojas C, Moniruzzaman M, Slusher BS, Bergles DE, et al. Inhibition of neutral sphingomyelinase 2 promotes remyelination. Sci Adv. 2020;6(40):eaba5210. doi: 10.1126/sciadv.aba5210.
- Mullard A. First-in-class tissue factor-targeted antibody–drug conjugate secures FDA approval. Nat Rev Drug Discov. 2021;20(11):806–806. doi: 10.1038/d41573-021-00167-8.
- Datta A, Kim H, Lal M, McGee L, Johnson A, Moustafa AA, Jones JC, Mondal D, Ferrer M, Abdel-Mageed AB, et al. Manumycin a suppresses exosome biogenesis and secretion via targeted inhibition of Ras/Raf/ERK1/2 signaling and hnRNP H1 in castration-resistant prostate cancer cells. Cancer Lett. 2017;408:73–81. doi: 10.1016/j.canlet.2017.08.020.
- Datta A, Kim H, McGee L, Johnson AE, Talwar S, Marugan J, Southall N, Hu X, Lal M, Mondal D, et al. High-throughput screening identified selective inhibitors of exosome biogenesis and secretion: a drug repurposing strategy for advanced cancer. Sci Rep. 2018;8(1):8161. doi: 10.1038/s41598-018-26411-7.
- Sasabe E, Tomomura A, Liu H, Sento S, Kitamura N, Yamamoto T. Epidermal growth factor/epidermal growth factor receptor signaling blockage inhibits tumor cell-derived exosome uptake by oral squamous cell carcinoma through macropinocytosis. Cancer Sci. 2022;113(2):609–621. doi: 10.1111/cas.15225.
- Chowdhury S, Matrana MR, Tsang C, Atkinson B, Choueiri TK, Tannir NM. Systemic therapy for metastatic non-clear-cell renal cell carcinoma: recent progress and future directions. Hematol Oncol Clin North Am. 2011;25(4):853–869. doi: 10.1016/j.hoc.2011.05.003.
- Greenberg JW, Kim H, Moustafa AA, Datta A, Barata PC, Boulares AH, Abdel-Mageed AB, Krane LS. Repurposing ketoconazole as an exosome directed adjunct to sunitinib in treating renal cell carcinoma. Sci Rep. 2021;11(1):10200. doi: 10.1038/s41598-021-89655-w.
- Wu X, Kang M, Wang D, Zhu M, Hu Y, Zhang Y, Deng C, Chen J, Teng L. Heparan sulfate analogues regulate tumor-derived exosome formation that attenuates exosome functions in tumor processes. Int J Biol Macromol. 2021;187:481–491. doi: 10.1016/j.ijbiomac.2021.07.110.
- Shin JM, Lee CH, Son S, Kim CH, Lee JA, Ko H, Shin S, Song SH, Park S-S, Bae J-H, et al. Sulfisoxazole elicits robust antitumour immune response along with immune checkpoint therapy by inhibiting Exosomal PD-L1. Adv Sci. 2022;9(5):e2103245. doi: 10.1002/advs.202103245.
- Im EJ, Lee CH, Moon PG, Rangaswamy GG, Lee B, Lee JM, Lee J-C, Jee J-G, Bae J-S, Kwon T-K, et al. Sulfisoxazole inhibits the secretion of small extracellular vesicles by targeting the endothelin receptor a. Nat Commun. 2019;10(1):1387. doi: 10.1038/s41467-019-09387-4.
- Catalano M, O’Driscoll L. Inhibiting extracellular vesicles formation and release: a review of EV inhibitors. J Extracell Vesicles. 2020;9(1):1703244. doi: 10.1080/20013078.2019.1703244.
- Pushpakom S, Iorio F, Eyers PA, Escott KJ, Hopper S, Wells A, Doig A, Guilliams T, Latimer J, McNamee C, et al. Drug repurposing: progress, challenges and recommendations. Nat Rev Drug Discov. 2019;18(1):41–58. doi: 10.1038/nrd.2018.168.
- Parvathaneni V, Kulkarni NS, Muth A, Gupta V. Drug repurposing: a promising tool to accelerate the drug discovery process. Drug Discov Today. 2019;24(10):2076–2085. doi: 10.1016/j.drudis.2019.06.014.
- Orme JJ, Enninga EAL, Lucien-Matteoni F, Dale H, Burgstaler E, Harrington SM, Ball MK, Mansfield AS, Park SS, Block MS, et al. Therapeutic plasma exchange clears circulating soluble PD-L1 and PD-L1-positive extracellular vesicles. J Immunother Cancer. 2020;8(2):e001113. doi: 10.1136/jitc-2020-001113.
- Wu TH, Wu CH, Huang CJ, Chang YC. Anticlogging hemofiltration device for mass collection of circulating tumor cells by ligand-free size selection. Langmuir. 2021;37(11):3399–3409. doi: 10.1021/acs.langmuir.0c03613.
- Tayebi M, Zhou Y, Tripathi P, Chandramohanadas R, Ai Y. Exosome purification and analysis using a facile microfluidic hydrodynamic trapping device. Anal Chem. 2020;92(15):10733–10742. doi: 10.1021/acs.analchem.0c02006.
- Ma C, Wang S, Wang G, Wu Y, Yang T, Shen W, Zhuang Y, Zhang L, Liu X, Yang L, et al. Protein spectrum changes in exosomes after therapeutic plasma exchange in patients with neuromyelitis optica. J Clin Apher. 2020;35(3):206–216. doi: 10.1002/jca.21781.
- Gordon SR, Maute RL, Dulken BW, Hutter G, George BM, McCracken MN, Gupta R, Tsai JM, Sinha R, Corey D, et al. PD-1 expression by tumour-associated macrophages inhibits phagocytosis and tumour immunity. Nature. 2017;545(7655):495–499. doi: 10.1038/nature22396.
- Lu T, Zhang Z, Zhang J, Pan X, Zhu X, Wang X, Li Z, Ruan M, Li H, Chen W, et al. CD73 in small extracellular vesicles derived from HNSCC defines tumour-associated immunosuppression mediated by macrophages in the microenvironment. J Extracell Vesicles. 2022;11(5):e12218. doi: 10.1002/jev2.12218.