Abstract
Objective
The study determined the seatbelt pre-pretensioner force needed and the time required to reposition average male front-seat passengers from forward-leaning to upright using finite element simulations of the Active SAFER Human Body Model (Active SHBM).
Methods
The Active SHBM was positioned in an initial forward-leaning position (29° forward from upright) on a deformable vehicle seat. A pre-pretensioner was modeled as a pre-loaded spring and its ability to reposition the forward-leaning Active SHBM to an upright position was simulated for twenty-four different pre-crash conditions. Four parameters were varied: (1) Automated Emergency Braking (AEB) active with 11 m/s2 or no AEB, (2) type of seatbelt system: Belt-In-Seat or B-pillar, (3) pre-pretensioner activation time (200 ms before, 100 ms before, or at the same time as AEB ramp-up), and (4) pre-pretensioner force (200 N, 300 N, 400 N, 600 N). The first thoracic vertebra fore-aft (T1 X) trajectories were compared against a reference upright position to determine the force and time needed to reposition and the effectiveness of repositioning in the different conditions.
Results
The lowest force enabling repositioning in all simulations was 400 N (no AEB, Belt-In-Seat). It took about 350 ms. In the presence of AEB, activating the pre-pretensioner 200 ms before AEB and using 600 N pre-pretensioner force was needed for repositioning (taking 200 ms with Belt-In-Seat and 260 ms with B-pillar installations). Repositioning was faster and thus more effective with the Belt-In-Seat seatbelt in all simulations.
Conclusions
All four parameters (presence of AEB, type of seatbelt system, pre-pretensioner activation time and force) affected the repositioning ability and time required. Far from all combinations repositioned a forward-leaning average male occupant model, but those found to be effective and fast appear as a feasible option for vehicle safety systems to reposition out-of-position occupants during pre-crash events.
Introduction
Vehicle occupants assume various sitting postures while traveling, with front-seat passengers adopting non-upright postures for most of their daily trips (Zhang et al. Citation2004). With the increasing popularity of autonomous vehicles, passengers will have greater freedom of movement compared to traditional vehicles, leading to the emergence of new postures. In particular, forward-leaning postures are becoming prevalent in current car passengers and are expected to become even more common as passengers engage in secondary tasks, such as using electronic devices while traveling (Koppel et al. Citation2019; Nie et al. Citation2020; Reed et al. Citation2020).
However, current safety systems are designed to protect occupants in traditional upright seating positions during emergency events or crashes. As a result, nontraditional postures of occupants may increase injury risks during crashes. Previous research has shown that leaning forward inboard or outboard can increase loadings, e.g., neck forces and moments during a crash (Benson et al. Citation1996; Reed et al. Citation2020; Viano et al. Citation2009). Additionally, forward-leaning passengers have exhibited greater excursions during pre-crash maneuvers, indicating poor upper body control (Leledakis et al. Citation2021; Reed et al. Citation2021). Therefore, effective countermeasures for forward-leaning vehicle occupants are needed for better protection in the event of a crash.
One such countermeasure is the seatbelt pre-pretensioner (PPT) that tenses the seatbelt during pre-crash to reduce belt slack during a crash and reduce forward displacements (Mages et al. Citation2011; Östh et al. Citation2015; Mishra et al. Citation2022). PPT was shown to reduce chest deflections and rib fracture risk in frontal crashes (Pipkorn and Wass Citation2017; Mishra et al. Citation2022). Furthermore, PPTs can effectively reposition forward-leaning occupants. For example, Graci et al. found that a shoulder belt load of 300 N reduced out-of-position during a low-speed frontal pre-crash, while Schilling et al. showed that a PPT force of 400 N was needed to reposition a UN R16 manikin leaning forward by 50 degrees in both physical tests and simulations (Schilling et al. Citation2021; Graci et al. Citation2022b). However, the effectiveness of PPTs in repositioning forward-leaning occupants during normal Automated Emergency Braking (AEB) maneuvers (up to 1 g and until 1 s) (Lindman et al. Citation2010; Brännström et al. Citation2014; Spitzhüttl and Liers Citation2019) has not been studied in previous literature.
Furthermore, previous studies have shown that avoidance maneuvers such as braking or steering precede half of the real-world crashes and influence the occupant posture (Ólafsdóttir et al. Citation2013; Stockman Citation2016; Ghaffari et al. Citation2018). Active Human Body Models (HBMs) can accurately simulate pre-crash muscular response and kinematics of vehicle occupants in addition to predicting kinematics and injuries during a crash (Meijer et al. Citation2013; Östh et al. Citation2015; Devane et al. Citation2019). Several active HBMs are available, including the Active SAFER HBM (Active SHBM), which has been validated to predict occupant kinematics during pre-crash maneuvers with and without PPT seatbelts (Ólafsdóttir et al. Citation2013; Östh et al. Citation2015; Larsson et al. Citation2019; Ólafsdóttir et al. Citation2019).
This study aimed to determine the magnitude of PPT force and time needed to reposition an average male Active SHBM seated on the front seat of a vehicle from a forward-leaning (29° forward from upright) to an upright position during pre-crash events. This can guide the development and fleet-deployment of PPT of the right specifications to ensure repositioning in the most common pre-crash events.
Methods
The Active SHBM version 10.0 (Pipkorn et al. Citation2023) was used to represent a forward-leaning occupant seated in the passenger seat of a mid-sized car. The Active SHBM represents an average male weighing 77 kg with a height of 175 cm (Robbins Citation1983). The active muscles are modeled with 1D Hill-type elements, and a closed-loop control strategy is followed for the muscle activation of the cervical and lumbar regions, and upper arms (Larsson et al. Citation2019). In this study, active muscles were used only for the neck and lumbar regions. The Active SHBM uses an angular position feedback control system to predict occupant postural responses during pre-crash scenarios.
A pre-simulation of 400 ms was run to position the Active SHBM in an upright position on the passenger seat using the Marionette method (Poulard et al. Citation2015). The Active SHBM H-point matched that of a SAE mannikin in the same vehicle environment. The angle of the torso, measured between a point on the shoulder (lateral acromion) and the H-point, was 21° from the vertical. The X-coordinate of the first thoracic (T1) vertebra was extracted from this position, normalized to 0, and used as the reference upright position or the upright threshold. A forward-leaning angle of 29° forward from the upright position (–8° from the vertical) was chosen to position the SHBM in a forward-leaning posture (). The forward-leaning position approximately matched with previous volunteer tests which measured an angle of 40°, measured between the seatback and the shoulder of the SHBM with the vertex on the seat bight (Graci et al. Citation2022b). A separate pre-simulation of 400 ms was then run to position the Active SHBM in the forward-leaning position on the passenger seat using the Marionette method and markers on the head center of gravity, T1, and arms (Poulard et al. 2015).
Figure 1. The average male Active SHBM seated in a forward-leaning posture, 29° forward from an upright position (shown in translucent shading) on a deformable seat inside the vehicle environment with the two different types of seatbelt systems: BPI (green belt textile) and BIS (red belt textile). Shoulder belt force can be measured as magnitude in the seatbelt direction or as X-component in direction of the global X-coordinate. Note: The BPI and BIS simulations were run separately. They are shown in the same figure just for comparison.
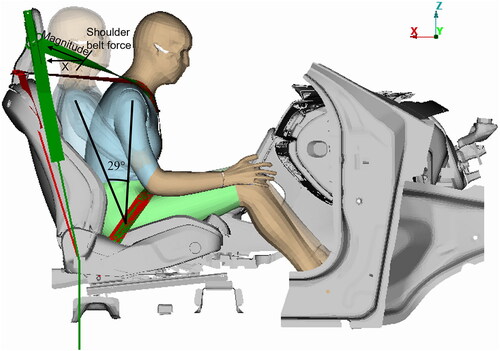
The vehicle interior finite element model comprised a deformable seat model developed for vehicle crash simulations, similar to the one previously used by Östh et al. (Citation2020). The seat model consisted of a steel chassis with springs modeled with beams, supporting a low-density foam for the seat bolster. A three-point seatbelt model with the D-ring located at the B-pillar (BPI) was used. Separate simulations were also carried out with a Belt-In-Seat seatbelt (BIS) with the D-ring located above the seatback, as shown in . The slipring friction coefficients at the D-ring and the buckle were 0.2 and 0.13, respectively. The seatbelt mesh included a combination of 1-dimensional (1D) and 2-dimensional (2D) elements. The 1D elements were used to connect the seatbelt to the retractor and the anchor point while the 2D elements were used for the seatbelt webbing. The width of the 2D seatbelt was 48 mm with 8 elements across the width. The material model used was a seatbelt material model (*MAT_SEATBELT) with a defined force-strain relationship giving 7% elongation at approximately 10 kN tensile force. The seatbelt model was used with a PPT at different force levels to reposition the Active SHBM to an upright position during braking. The PPT was simplified to a pre-loaded spring which gets released at the point of time as defined and results in an immediate increase in belt force. The in-crash functionalities of the seatbelt such as pyrotechnical retractor pretensioning and load limiting were not used as they typically would not be active in the low-force events simulated. The seatbelt system has been validated in-house (at both system and component levels) for pre-crash braking followed by frontal crash tests in an initial upright position (Mishra et al. Citation2022).
Two pre-crash scenarios were simulated. The first scenario represented an 11 m/s2 AEB, using a generic pulse representative of a modern car in which maximum braking was achieved after a 200 ms delay followed by a 200 ms ramp-up time (as shown in ) (Östh et al. Citation2022). The maximum braking level used was higher than reported by Graci et al. (Citation2021) but comparable to the maximum values reported by Mahdinia et al. (Citation2022). The total AEB duration simulated was 900 ms, realistic based on previously published data (Spitzhüttl and Liers Citation2019). The second scenario was at a constant velocity, without any vehicle dynamics intervention. The total simulation time for each simulation was 1200 ms, which included a 300 ms model initialization phase needed for the Active SHBM for initializing the muscle activations. All simulations were carried out using LS-DYNA explicit finite element solver, double precision version R9.3.1 (LSTC, Livermore, CA, USA).
Figure 2. The constant velocity pulse, i.e., “No AEB” pulse in black and the generic AEB pulse in blue. The dashed vertical lines indicate the different PPT activation times used.
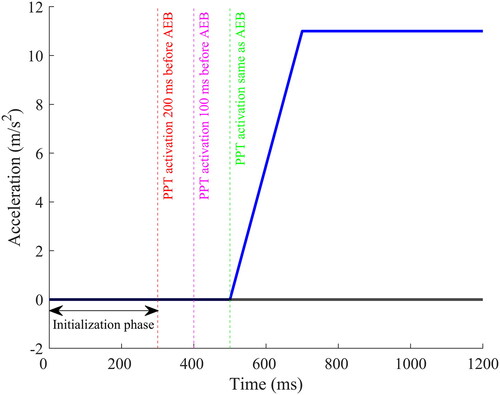
The simulation matrix comprised 24 simulations as shown in the Appendix (Table A1, see supplementary materials), with four parameters varied: AEB or No AEB, BPI or BIS system, PPT activation time, and PPT force. 16 simulations were run for the AEB scenario with either the BPI or BIS system. For the AEB and BPI simulations, three variations of the PPT activation time were simulated: PPT activated with the onset of AEB ramp-up, 100 ms before the AEB ramp-up, and 200 ms before the AEB ramp-up. For the AEB and BIS simulations, the PPT was only activated 200 ms before the AEB ramp-up. Four different PPT force levels were simulated based on previous studies that used PPT for repositioning (Schilling et al. Citation2021; Graci et al. Citation2022b) or different purposes (Ólafsdóttir et al. Citation2013; Weller et al. Citation2022): 200 N (similar to the 170 N used in (Ólafsdóttir et al. Citation2013)), 300 N (Graci et al. Citation2022b), 400 N (Schilling et al. Citation2021), and 600 N (Weller et al. Citation2022).
For the No AEB scenario, 8 simulations were performed with either the BPI or BIS system. PPT was activated at 300 ms, i.e., at the end of the model initialization phase for all simulations. The same four PPT force levels were used as for the AEB scenario.
Nodal X-coordinates (X direction is the frontal direction as shown in ) of the T1 vertebra relative to the vehicle motion from the forward-leaning simulations were extracted and presented with respect to time. These were then compared to the reference position to determine the PPT force and time needed to reposition the Active SHBM to an upright position.
Time histories of the magnitude of shoulder belt forces, X components of shoulder belt forces (measured at a cross-section between the D-ring and the shoulder, as shown in ) and muscle activation levels from the cervical region: sternocleidomastoid (SCM) and C4-level multifidus (C4M) muscles, and lumbar region: rectus abdominis (RA) and lumbar multifidus (LMF) muscles were also extracted and compared.
Results
The Active SHBM was considered to be fully repositioned if the T1 X-coordinate was no farther than 10 mm (chosen arbitrarily) from the upright threshold at zero of the X-coordinate at some time during the time series. Smaller X-coordinate values indicate more upright postures (negative values indicating recline). compares the nodal X trajectories of the T1 vertebra (plotted relative to the PPT activation time) from the simulations passing the upright threshold. From initial forward-leaning (at approximately 220 mm), we see how the Active SHBM moves backwards to more upright postures. The repositioning in the conditions NoAEB_BIS_600N and AEB_BIS_200ms_600N (pink and red lines) was fastest. The Active SHBM was fully repositioned within approximately 200 ms in these conditions. NoAEB_BIS_400N (pink dashed line) had the lowest force and was slowest (approximately 350 ms) until full repositioning. Repositioning was slower with the BPI seatbelt (green and black lines) than the BIS seatbelt.
Figure 3. The T1 X-coordinates from the simulations passing the upright threshold plotted relative to the PPT activation time.
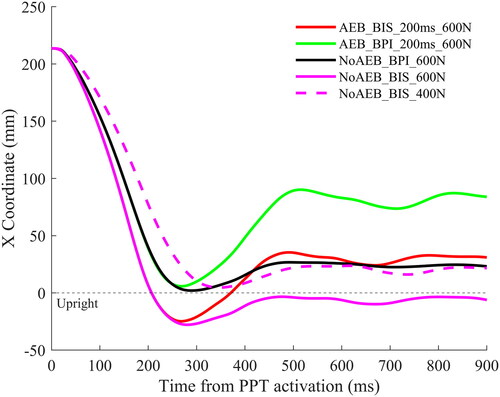
The peak T1 X-coordinates and time of the peak X-coordinates (most rearward position) were extracted for further analysis () from all simulations (except the AEB_BPI_Same_200N and AEB_BPI_Same_300N in which the Active SHBM did not move backwards from initial position). In the AEB_BPI_Same and AEB_BPI_100ms simulations, the SHBM was not repositioned under any condition. The AEB_BPI_200ms and AEB_BIS_200ms simulations were similar. The Active SHBM was pulled back faster and farther with increased force. The Active SHBM was fully repositioned to an upright position approximately 60 ms later in AEB_BPI_200ms_600N than in AEB_BIS_200ms_600N (). Additionally, in the AEB_BIS_200ms_400N simulation, the SHBM was nearly repositioned to an upright position in around 310 ms. In the No AEB scenario, the Active SHBM was fully repositioned in the NoAEB_BIS_600N and NoAEB_BIS_400N simulations, taking about 200 ms with 600 N and 350 ms with 400 N PPT forces, respectively. Moreover, it was also fully repositioned in NoAEB_BPI_600N simulations, taking about 290 ms.
Figure 4. T1 X-coordinates plotted relative to the PPT activation time. The different colors represent the different simulation conditions, and the markers represent the PPT force levels.
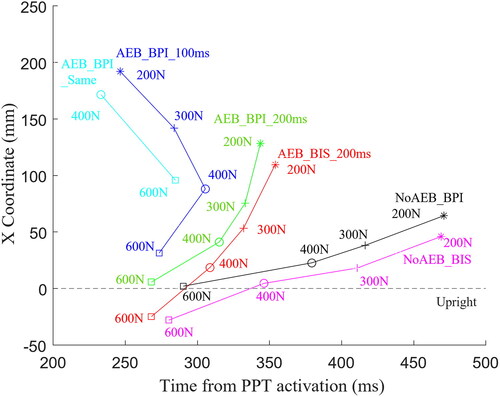
depicts a comparison of the X components of the shoulder belt forces from the simulations passing the upright threshold with regards to repositioning: AEB_BIS_200ms_600N, AEB_BPI_200ms_600N, NoAEB_BPI_600N, NoAEB_BIS_600N, and NoAEB_BIS_400N. The BIS system resulted in higher belt forces than the BPI system, even for the same PPT force level, mainly due to the different belt geometry in the BIS system as seen in . In addition, the belt force signals from the BPI simulations were noisy, likely due to the folding of the seatbelt near the cross-section where the force was measured. Figure A1 in the Appendix shows the magnitudes of the shoulder belt forces (see supplementary materials).
Figure 5. Time series of the X components of the shoulder belt forces from the simulations passing the upright threshold. Note: Here time is absolute, not relative to the PPT activation time. These belt forces are not the magnitude of the seatbelt force along the direction of the seatbelt, but the component in global X-coordinate direction.
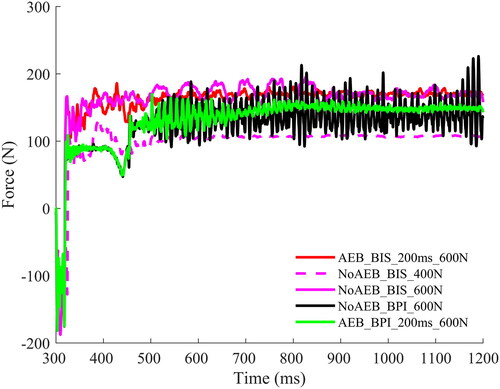
Muscle activations from the cervical and lumbar muscles were also compared in Appendix (see supplementary materials). Muscle activation levels were higher with the BIS system than the BPI system and increased with the increase in PPT force, consistent with the kinematics results. Furthermore, there were no substantial differences in muscle activation levels between the AEB and No AEB scenarios.
Discussion
This study determined the magnitude of PPT force, and the time required to reposition an average male Active SHBM from a forward-leaning to an upright position during pre-crash events. The lowest force enabling repositioning in all simulations was 400 N (no AEB, BIS). It took about 350 ms.
The repositioning was faster and the Active SHBM was pulled back farther with the BIS system even for the same PPT force and activation time. Thus, repositioning was more effective with the BIS system than the BPI system. It was due to the belt geometry of the BIS system resulting in a higher X component (in the direction of the pulling back) on the shoulder belt force even at the same PPT force level as the BPI system. As expected, higher forces and earlier activation aided repositioning.
With AEB, activating the PPT 200 ms before AEB ramp-up, 600 N PPT force was needed for full repositioning, regardless of the belt system. However, repositioning was faster with BIS (needing 200 ms) than BPI (needing 260 ms). Without AEB, the seatbelt PPT was more effective in repositioning, as even 400 N was enough to fully reposition with the BIS system in about 350 ms. However, with the BPI system (which is standard in current midsize cars), 400 N was not enough, with full repositioning being achieved only with 600 N in around 290 ms.
Our findings are similar to a previous study that found 400 N PPT force was needed to reposition a UN R16 dummy without AEB in a BPI system (Schilling et al. Citation2021). However, in our study, 400 N was sufficient to reposition the Active SHBM without AEB in a BIS system, not in a BPI system. This could be due to a difference in mass distribution and lumbar stiffness between the dummy and the HBM models, or due to the Active SHBM’s muscle controller functions using feedback control to generate a muscle response that tries moving the model back to its reference position (the initial forward-leaning position in this study). Therefore, the Active SHBM is constantly trying to go back to the initial forward-leaning position while it is being pulled back by the seatbelt PPT. Coming to the results with AEB, it has been reported earlier that 300 N PPT force was needed to reposition volunteers under 0.95 g AEB that lasted for 300 ms (Graci et al. Citation2022b). In the current study, a 1.1 g AEB lasting 900 ms was used. Therefore, more force was needed to reposition the Active SHBM in the AEB scenario simulated here.
There were no substantial differences in muscle activation levels between the AEB and No AEB conditions; however, they were higher with the BIS system. The muscle activations also increased with the increase in PPT force, contrary to the results from a previous volunteer study, which reported lower activation with higher PPT force (Graci et al. Citation2022a). This was also due to the Active SHBM trying to maintain its initial forward-leaning position and hence a larger deviation from the reference (forward-leaning) position gave a higher muscle activation. Focusing on the individual muscles, the SCM and RA muscles, which are flexors, had higher muscle activation levels than the C4M and LMF muscles, which are extensors. The cervical and lumbar flexors are used to flex or bend the torso forward, while the extensors are used to extend or move back, i.e., maintain an upright posture. Hence, it is logical that the flexors have more activation than the extensors as the Active SHBM was trying to maintain its initial forward-leaning position, resisting the repositioning to an upright position. However, this kind of model response may not be physiological as muscle activation trends are contrary to those reported in volunteer testing (Graci et al. Citation2022a). Future updates of the Active SHBM’s feedback controller should also consider repositioning load cases for a more physiological response of the model.
The findings in our study may have implications for the design and development of seatbelt systems, particularly in relation to pre-crash events. We identified the PPT activation time (PPT activated 200 ms before AEB ramp-up starts) and the seatbelt type (BIS) to have substantial impacts on the repositioning of occupants from forward-leaning to upright. Although more work in the form of field data analysis is needed, the ability to reposition occupants rapidly and effectively from a forward-leaning to an upright position could reduce the risk of injury during crash events later. The use of PPTs could be a feasible option as a powerful PPT (of at least 400 N), preferably activated before a crash, is capable of repositioning. We see no fundamental obstacles for implementation; PPT seatbelts have been available in some cars for more than two decades now (Schoeneburg and Breitling Citation2005), even if they are not standard systems and any reliable data about their field acceptance is not available yet. It has been demonstrated in testing that activating the PPT before an AEB, as opposed to simultaneously, can significantly reduce forward displacements (Mages et al. Citation2011); such systems are in development (Straßburger et al. Citation2023). The SAFE-UP project suggested a PPT with 200 to 1000 N force to be common in cars in five to ten years (Östling et al. Citation2022). A prototype PPT with 600 N force has been tested in a volunteer study and shown to be safe (Weller et al. Citation2022). Thus, developing a powerful PPT activated before AEB ramp-up appears technically feasible.
Limitations
This is a limited study which represents a limited set of possible conditions. Only force and time required to reposition an average male Active SHBM were studied. It is possible that the force required to reposition other occupants, such as children, females, 95th percentile, or elderly individuals, may differ substantially. In particular, age and body mass index can significantly affect the head forward displacements in braking (Reed et al. Citation2018). Therefore, further research is needed to investigate the force required to reposition other occupant groups. The force required to reposition is also dependent on the target upright threshold. We chose an arbitrary target of 10 mm from upright at any point during the time series due to the lack of a standardized target. It could be possible that leaning forward up to 50 mm from upright is acceptable in terms of injury risks in a subsequent crash event. Thus, more work is needed to agree on a standardized upright threshold target. In addition, it is also worth noting that our study only focused on AEB or no AEB. Other potential pre-crash events, such as steering or combined AEB and steering, may require different types of occupant repositioning, and further research should investigate the force needed for such maneuvers. Moreover, the PPT was modeled using a pre-loaded spring, similar to some previous studies, resulting in an immediate force ramp-up (Mishra et al. Citation2022). In reality, it takes about 100 ms for a PPT to reach the desired force level (Schilling et al. Citation2021). Future work modeling PPTs more accurately is needed, for example by using feedback control loop modeling of the mechatronic parts of a PPT (Schilling et al. Citation2021). Finally, the Active SHBM used in the study is not validated for such repositioning simulations. It relies on a muscle controller system that has been validated for occupant kinematics in an upright position during pre-crash maneuvers. Thus, its response during repositioning from out-of-position initial postures is only an extrapolation. More work, both testing and modeling, is needed to better understand the human kinematic and muscle responses during repositioning load cases and thus validate the Active SHBM for such cases.
Conclusion
Our study provides insights into the PPT force, and the time required to reposition an average male Active SHBM from a forward-leaning to an upright position during pre-crash events. Activating the PPT earlier and with higher forces resulted in more effective repositioning. The seatbelt geometry also substantially influenced repositioning: repositioning was faster and thus more effective with the BIS seatbelt than with the BPI seatbelt. Modeling and evaluating PPT as a pre-loaded spring with rapid force increase suggests that the use of PPTs, with at least 400 N force in the absence of AEB, and 600 N force in the presence of AEB, is a feasible option to reposition out-of-position occupants. Future research should refine HBM and safety system models to strengthen further evaluation with varying occupant characteristics (such as height and weight) and pre-crash conditions.
Supplemental Material
Download Zip (497.8 KB)Acknowledgments
The authors want to thank our partners at SAFER: The Vehicle and Traffic Safety Centre at Chalmers, specifically Chalmers University of Technology, Dynamore Nordic AB, and Volvo Cars for their joint efforts in developing the SAFER HBM.
Additional information
Funding
References
- Benson BR, Smith GC, Kent RW, Monson CR. 1996. Effect of seat stiffness in out-of-position occupant response in rear-end collisions. SAE transactions. 1958–1971
- Brännström M, Coelingh E, Sjöberg J. 2014. Decision-making on when to brake and when to steer to avoid a collision. Int J Veh Saf. 7(1):87–106. doi:10.1504/IJVS.2014.058243.
- Devane K, Johnson D, Gayzik FS. 2019. Validation of a simplified human body model in relaxed and braced conditions in low-speed frontal sled tests. Traffic Inj Prev. 20(8):832–837. doi:10.1080/15389588.2019.1655733.
- Ghaffari G, Brolin K, Bråse D, Pipkorn B, Svanberg B, Jakobsson L, Davidsson J. 2018. Passenger kinematics in Lane change and Lane change with Braking Manoeuvres using two belt configurations: standard and reversible pre-pretensioner. Proceedings of the International Research Council on Biomechanics of Injury (IRCOBI) Conference. Athens, Greece.
- Graci V, Griffith M, Seacrist T, Brase D, Mishra E, Pipkorn B, Lubbe N, Arbogast K. 2022a. Bracing strategies in forward leaning occupants: the effect of seat-belt pre-pretension and a startle warning during braking acceleration. Proceedings of the International Research Council on Biomechanics of Injury (IRCOBI) Conference. Porto, Portugal.
- Graci V, Griffith M, Seacrist T, Brase D, Mishra E, Pipkorn B, Lubbe N, Arbogast KB. 2022b. Repositioning forward-leaning vehicle occupants with a pre-pretensioner belt and a startle-based warning in pre-crash scenarios. Traffic Inj Prev. 2022:1–6. doi:10.1080/15389588.2022.2115294.
- Graci V, Maltenfort M, Schneider M, Griffith M, Seacrist T, Arbogast KB. 2021. Quantitative characterization of AEB pulses across the modern fleet. Traffic Inj Prev. 22(sup1):S62–S67. doi:10.1080/15389588.2021.1961227.
- Koppel S, Jiménez Octavio J, Bohman K, et al. 2019. Seating configuration and position preferences in fully automated vehicles. Traffic Inj Prev. 20(sup2):S103–S109. doi: 10.1080/15389588.2019.1625336.
- Larsson E, Iraeus J, Fice J, Pipkorn B, Jakobsson L, Brynskog E, Brolin K, Davidsson J. 2019. Active human body model predictions compared to volunteer response in experiments with braking, lane change, and combined manoeuvres. Proceedings of the International Research Council on Biomechanics of Injury (IRCOBI) Conference. Florence, Italy.
- Leledakis A, Östh J, Davidsson J, Jakobsson L. 2021. The influence of car passengers’ sitting postures in intersection crashes. Accid Anal & Prev. 157:106170. doi:10.1016/j.aap.2021.106170.
- Lindman M, Ödblom A, Bergvall E, Eidehall A, Svanberg B, Lukaszewicz T. 2010. Benefit estimation model for pedestrian auto brake functionality. 4th International Conference on Expert Symposium on Accident Research. Hannover, Germany.
- Mages M, Seyffert M, Class U. 2011. Analysis of the pre-crash benefit of reversible belt pre-pretensioning in different accident scenarios. Proceedings of the 22nd International Technical Conference on The Enhanced Safety of Vehicles (ESV) Conference. Washington DC, USA.
- Mahdinia I, Khattak AJ, Mohsena Haque M. 2022. How effective are pedestrian crash prevention systems in improving pedestrian safety? Harnessing large-scale experimental data. Accid Anal Prev. 171:106669. doi:10.1016/j.aap.2022.106669.
- Meijer R, Elrofai H, Broos J, van Hassel E. 2013. Evaluation of an active multi-body human model for braking and frontal crash events. Proceedings of the 23rd International Technical Conference on The Enhanced Safety of Vehicles (ESV) Conference. Seoul, South Korea.
- Mishra E, Mroz K, Pipkorn B, Lubbe N. 2022. Effects of automated emergency braking and seatbelt pre-pretensioning on occupant injury risks in high-severity frontal crashes. Front Future Transp. 3:883951. doi:10.3389/ffutr.2022.883951.
- Nie B, Gan S, Chen W, Zhou Q. 2020. Seating preferences in highly automated vehicles and occupant safety awareness: A national survey of Chinese perceptions. Traffic Inj Prev. 21(4):247–253. doi:10.1080/15389588.2020.1738013.
- Ólafsdóttir JM, Östh J, Brolin K. 2019. Modelling reflex recruitment of neck muscles in a finite element human body model for simulating omnidirectional head kinematics. Proceedings of the International Research Council on Biomechanics of Injury (IRCOBI) Conference. Florence, Italy.
- Ólafsdóttir JM, Östh J, Davidsson J, Brolin K. 2013. Passenger kinematics and muscle responses in autonomous braking events with standard and reversible pre-tensioned restraints. Proceedings of the International Research Council on Biomechanics of Injury (IRCOBI) Conference. Gothenburg, Sweden.
- Östh J, Bohman K, Jakobsson L. 2020. Evaluation of kinematics and restraint interaction when repositioning a driver from a reclined to an upright position prior to frontal impact using active human body model simulations. Proceedings of the International Research Council on Biomechanics of Injury (IRCOBI) Conference. Munich, Germany.
- Östh J, Brolin K, Bråse D. 2015. A human body model with active muscles for simulation of pretensioned restraints in autonomous braking interventions. Traffic Inj Prev. 16:304–313. doi:10.1080/15389588.2014.931949.
- Östh J, Larsson E, Jakobsson L. 2022. Human body model muscle activation influence on crash response. Proceedings of the International Research Council on Biomechanics of Injury (IRCOBI) Conference. Porto, Portugal.
- Östling M, Daddetta GA, Lubbe N, da Silva Zimmer A. 2022. SAFE-UP D4.2: architecture of Passive Safety Systems (No. D4.2). Proactive SAFEty systems and tools for a constantly UPgrading road environment. https://static1.squarespace.com/static/5efaed43294db25b18168717/t/627e7860d8a9e0674d590787/1652455536483/SAFE-UP_Deliverable_D4.2.pdf.
- Pipkorn B, Wass J. 2017. Pre-crash triggered pretensioning of the seat belt for improved safety. Proceedings of the 25th International Technical Conference on the Enhanced Safety of Vehicles (ESV) Conference. Detroit, Michigan, USA.
- Pipkorn B, Jakobsson L, Iraeus J, Östh J. 2023. The SAFER HBM – A Human Body Model For Seamless Integrated Occupant Analysis For All Road Users. Proceedings of the 27th International Technical Conference on the Enhanced Safety of Vehicles (ESV). Yokohama, Japan.
- Poulard D, Subit D, Donlon JP, Kent RW. 2015. Development of a computational framework to adjust the pre-impact spine posture of a whole-body model based on cadaver tests data. J Biomech. 48(4):636–643. doi:10.1016/j.jbiomech.2014.12.050.
- Robbins DH. 1983. Anthropometric specifications for mid-sized male dummy, volume 2, and for small female and large male dummies, volume 3. Report No. UMTR I -83-53-2. Available online: https://deepblue.lib.umich.edu/bitstream/handle/2027.42/260/72269.0001.001.pdf?sequence=2
- Reed MP, Ebert SM, Jones ML, Park BKD, Hallman JJ, Sherony R. 2018. Passenger head kinematics in abrupt braking and lane change events. Traffic Inj Prev. 19(sup2):S70–S77. doi:10.1080/15389588.2018.1481957.
- Reed MP, Ebert SM, Jones ML, Hallman JJ. 2020. Prevalence of non-nominal seat positions and postures among front-seat passengers. Traffic Inj Prev. 21(sup1):S7–S12. doi:10.1080/15389588.2020.1793971.
- Reed MP, Ebert SM, Jones MH, Park BK. 2021. Occupant dynamics during crash avoidance maneuvers. United States. Department of Transportation. Report No. UMTRI-2020-10. National Highway Traffic Safety Administration; Feb 1. doi:10.7302/1700.
- Schilling S, Soni A, Hinrichs H, Verheyen C, Grikschat M, Eickhoff B, Lucht A, Cirstea A. 2021. Coupling feedback control loop-based model in Simulink to finite element model in LS-Dyna: application to reposition forward leaning occupant to upright posture. Proceedings of the 13th European LS-DYNA Conference. Ulm, Germany.
- Schoeneburg R, Breitling T. 2005. Enhancement of active and passive safety by future PRE-SAFE® systems. Proceedings of the 19th International Technical Conference On The Enhanced Safety Of Vehicles (ESV) Conference. Washington DC, USA.
- Spitzhüttl F, Liers H. 2019. Calculation of the Point of No Return (PONR) From Real-World Accidents. Proceedings of the 26th International Technical Conference On The Enhanced Safety Of Vehicles (Esv) Conference. Eindhoven, Netherlands.
- Stockman I. 2016. Safety for children in cars: focus on three point seatbelts in emergency events. Gothenburg, Sweden: Chalmers University of Technology.
- Straßburger P, Grotz B, Heiß S, Arbter B, Hachmann K, Metzger J, Ocelić N. 2023. Predictive safety: towards holistic top-down systems engineering for pre-crash systems. Proceedings of the 27th International Technical Conference on the Enhanced Safety of Vehicles (ESV) Conference. Yokohama, Japan.
- Viano DC, Parenteau CS, Burnett RA, James MB. 2009. Influence of Seating Position on Dummy Responses with ABTS Seats in Severe Rear Impacts. SAE Technical Paper. doi:10.4271/2009-01-0250.
- Weller G, Czepulowski O, Diwischek L, Hoffmann F, Miller T, Schnabl R, Sprongl P. 2022. Kontrollierbarkeit und Akzeptanz einer unvorhergesehenen, reversiblen Hochleistungsgurtstraffung. in Fahrzeugsicherheit 2022 Auf dem Weg zur Fahrzeugsicherheit 2030, VDI-Berichte. VDI Wissensforum GmbH. Germany: Dusseldorf. doi:10.51202/9783181023877.
- Zhang LL, Chen L, Vertiz A, Balci R. 2004. Survey of Front Passenger Posture Usage in Passenger Vehicles. SAE Technical Paper; No. 2004-01-0845. doi:10.4271/2004-01-0845.