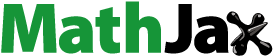
ABSTRACT
Cellulose aerogels are solid materials with high porosity, low density, and large surface area, and show excellent absorption of mechanical stress. These characteristics are appropriate for the development of new high-value products. However, over the years, it has been increasingly difficult to compare different studies about this material due to the different approaches used. In the present work, three different raw materials, bacterial cellulose from synthetic medium (BC), bacterial cellulose from cashew juice permeate (BCP), and eucalyptus nanocellulose (EC) were double-functionalized with TEMPO oxidation followed by silanization and freeze-dried, resulting in three different types of cellulose aerogels. These aerogels were evaluated for their performance in the absorption of organic oils/solvents and mechanical properties. The raw materials lead to aerogels with different characteristics. BC aerogel has the best mechanical properties and absorption capacity after 7 cycles; BCP aerogel presented the highest values for oil absorption and EC aerogel for organic solvent absorption.
摘要
纤维素气凝胶是一种具有高孔隙率、低密度和大表面积的固体材料, 具有良好的机械应力吸收性能. 这些特点适合开发新的高价值产品. 然而, 多年来, 由于使用的方法不同, 对这一材料的不同研究进行比较变得越来越困难. 在目前的工作中, 三种不同的原料, 即合成培养基中的细菌纤维素 (BC) 、腰果汁渗透液中的细菌纤维 (BCP) 和桉树纳米纤维素 (EC) 通过TEMPO氧化、硅烷化和冷冻干燥进行双重官能化, 形成三种不同类型的纤维素气凝胶. 对这些气凝胶的有机油/溶剂吸收性能和机械性能进行了评估. 这些原材料可以产生具有不同特性的气凝胶。BC气凝胶在7次循环后具有最佳的力学性能和吸收能力, BCP气凝胶的吸油率最高, EC气凝胶的有机溶剂吸收率最高.
Introduction
Aerogels are solid materials with a large surface area and low density. The term aerogel can be used to define a material with such characteristics in which the fluid in the gel pores is replaced with air. If the replacement is performed with freeze-drying, the material can be named cryogel (Beh et al. Citation2020; Boukind et al. Citation2021; Garcia-Bordejé, Benito, and Maser Citation2021; Guan et al. Citation2020; Takeshita et al. Citation2021; Wang et al. Citation2020; Wu et al. Citation2020). Modifications in the aerogel structure affect the application of the material. Therefore, it is possible to produce an aerogel suitable for particle filtering, selective absorption for oil and solvent spills, and wastewater treatment, based on the type of used functionalization (Abouzeid et al. Citation2019; Heath and Thielemans Citation2010; Hosseini et al. Citation2019, Citation2020).
Cellulose aerogels have a structure composed of natural and renewable polymer chains. They have excellent absorption of mechanical stress and high liquid absorption (Chen et al. Citation2011). Many articles were published describing protocols to produce cellulose aerogels and showing practical applications. Nevertheless, it has been increasingly difficult to compare different cellulose aerogels because there is a myriad of different approaches to produce them. These approaches are related to different types of chemical functionalization, nanocellulose extraction routes, and cellulose sources, which may affect the performance of the final cellulosic products (De Azeredo Citation2009; Dufresne Citation2012; Kvien and Oksman Citation2007).
TEMPO-mediated oxidation and silanization are procedures commonly used on cellulose aerogels. The cellulose oxidation mediated by TEMPO is a regioselective oxidative reaction of primary alcohols (Isogai and Kato Citation1998) that results in partially embrittled fibrils as a salt of celluronic acid (Huang et al. Citation2020; Motta Neves et al. Citation2020; Tanpichai and Wimolmala Citation2021; Wu and Cheng Citation2017; Wu et al. Citation2018). Many silanization approaches can be used to hydrophobized cellulose, such as the vapor deposition of silicon derivatives or silane solutions impregnation (Cheng et al. Citation2017; Fan et al. Citation2017; Feng et al. Citation2015; Jin et al. Citation2015; Piperopoulos et al. Citation2021; Xiao et al. Citation2015).
Nanocellulose can be obtained with bottom-up or top-down approaches. A common bottom-up procedure is using bacteria to produce a net of cellulose nanofibrils (Rathinamoorthy and Kiruba Citation2020; Rivas et al. Citation2004) while a typical top-down approach is breaking down the fibers (Yousefi et al. Citation2013). There are differences in the crystalline structure between these two materials that may result in different products (de Amorim et al. Citation2020) or impact a chemical functionalization on the fibers.
A few companies worldwide are already scaling up the production of nanocellulose (Sharma et al. Citation2019). Agricultural byproducts or cellulose fibers from a commodity source, such as raw cotton linter or eucalyptus (de Amorim et al. Citation2020), are the main raw materials for top-down nanocellulose. The main source of bottom-up nanocellulose is still originated from bacterial cellulose produced in a synthetic medium, like HS (Nyakuma et al. Citation2021; Poddar and Dikshit Citation2021).
The HS medium is a standard for research related to bacterial cellulose, but it represents about 30% of the overall cost and 65% of the costs with fermentation (Rivas et al. Citation2004). Cheaper raw materials like agricultural residues or byproducts may reduce the bottom-up nanocellulose production cost (Rathinamoorthy et al. Citation2021). One of these materials is the cashew juice permeate, a by-product of the extraction process of the yellow dye from the cashew apple juice. This permeated juice is an extract rich in sugars and nitrogen (BARROS Citation2016), which can replace the HS growth medium.
The effect of the type of cellulose (top-down or bottom-up) and the origin of the cellulose (from a synthetic or a natural medium), even after chemical functionalization, may impact the final properties of the material. In this research, three different types of nanocellulose were double functionalized, with a TEMPO-oxidation followed by silanization: BC of synthetic medium (BC), BC of cashew juice permeate (BCP), and eucalyptus cellulose (EC). To the best of our knowledge, no research has been published on the effect of cellulose sources on the properties of aerogels. Furthermore, no report examines the structure and mechanical properties of aerogels obtained from cashew juice permeate.
Materials and methods
Raw materials and reagents
Bacterial cellulose obtained in synthetic medium HS (Schramm and Hestrin Citation1954) (BC) and those obtained in the cashew juice permeate medium (BCP) were produced by the strain Komagataeibacter xylinus ATCC 53,582 under static conditions in the Laboratory of Microbiology of Embrapa Tropical Agroindustry (Pereira et al. Citation2020). The nano fibrillated eucalyptus cellulose (EC), at a concentration of 3.0% (w/w), was supplied by Suzano Pulp and Paper (Microfibrillated cellulose; source: Eucalyptus bleached kraft pulp; Limeira, SP, Brazil, product code 13.465–970).
The NaOH, NaBr, NaOCl, and toluene were from Dynamics (Caxias do Sul, Brazil). The TEMPO (2,2,6,6-tetramethylpiperidine-1-oxyl, 156.25 mol·g−1) radical, methyltrimethoxysilane (MTMS), acetone, ethanol, and mineral oil were obtained from Sigma Aldrich (São Paulo, Brazil). Chloroform was purchased from Synth (Diadema, Brazil). Mobil DTE 25 (Spring, USA) engine oil was obtained from a commercial establishment. The reagents were used without any modification or process.
Processing of different cellulose sources
TEMPO-mediated oxidation
The BC, BCP, and EC were oxidized using the method proposed by Saito et al. (Saito et al. Citation2007) with adaptations (Pereira et al. Citation2020). After the complete dissolution of NaBr and TEMPO, 1 g of cellulose (dry basis) was added. After homogenization, NaOCl (5.0 mmol) was added dropwise, under stirring (1000 rpm) at 25 ± 1°C. The pH was adjusted to 10.0–10.5 with NaOH 1.0 mol. L−1 and the conditions were maintained for 150 min. Ethanol 95% v/v (60 mL) was added to stop the reaction, and the characteristic yellow color of the reactional medium changed to white. Each oxidized cellulose was washed with 200 mL of distilled H2O, centrifuged twice (4°C, 24,456 x g, 15 min; Hitachi Koki Himac CR22GIII, Japan), and the formed hydrogel stored in a refrigerator (4 °C).
Nanofibrillation
The BC (1.76 dry weight), BCP (1.76 dry weight), and EC (1.89 dry weight) were resuspended in 500 mL of distilled H2O at 1.0% (w/w) concentration and subjected to nanofibrillation in a high rotation blender (Vitamix, Vita-Prep 3 model) at maximum rotation (24,000 rpm, 38,707 x g) for 30 min. Finally, the three suspensions were stored in a refrigerator (4 °C) for further characterization and processing.
Silanization
Silanization was performed with a polymethylsiloxane solution. In a beaker, under rotor stirring (3,000 rpm, 605x g) and at 25 ± 1°C, 0.1 mol·L−1 HCl was added until pH 4.0. Methyltrimethoxysilane (MTMS) was added slowly to a final concentration of 2.0% (w/w). A MTMS solution (100 g, 2.0% w/w), pH 4.0, was added to a nanofibrils suspension (100 g, 1.0% w/w) dropwise over 1 h under vigorous stirring at room temperature (25 ± 1 °C). After the addition of polymethylsiloxane, the suspension was stirred for 2 h (Zhang et al. Citation2014), and stored in a refrigerator (4 °C).
Production of cellulose aerogels
The BC, BCP, and EC suspensions were used to prepare the aerogels. The suspensions (30 mL) were deposited into 50 mL Falcon tubes, flash-frozen in liquid nitrogen, and stored in Ultrafreezer (SANYO, MDF-U33 V, Moriguchi, Japan) at −80°C. The samples were freeze-dried (K105 Liotop) at a pressure of 1000–1200 μHg, and temperature from −100 to −30°C for 48 h. All the aerogels were prepared in duplicate.
Characterization
Determination of carboxylate content by conductometry
A titration method based on Besbes, Alila, and Boufi (Citation2011), and Saito and Isogai (Citation2004) (Besbes, Alila, and Boufi Citation2011; Saito and Isogai Citation2004) was performed to determine the – COO- content in the oxidized cellulose. An amount of 183.3 mL of distilled H2O and 16.67 mL of 0.01 mol. L−1 NaCl was added to 1.0 g of dry cellulose and the system was homogenized. Then, a solution of 0.1 mol·L−1 HCl was added to adjust the pH to 2.5–3.0. The titration was done by the addition of 0.04 mol·L−1 NaOH. The carboxylate content in mol·g−1 was determined by EquationEquation (1)(1)
(1) :
where CNaOH is the NaOH concentration used in the titration, m is the cellulose mass, V0 is the volume of NaOH required for the HCl neutralization (strong acid), and V1, is for the -COOH neutralization (weak acid).
Fourier transform infrared absorption spectroscopy (FTIR)
The FTIR spectra were performed on a Shimadzu FTIR-8300 spectrophotometer, with 30 scans, in the range of 4000–400 cm−1. The samples were oven-dried at 50°C and mixed with KBr at a ratio of 3.0% (w/w). Graphics were generated in OriginPro9.1 and modified in freeware Gimp 2.6 (version 2.10.14, https://www.gimp.org/).
Porosity and density
The aerogels obtained from each sample were sectioned into a cylinder shape to calculate the apparent density. The height, diameter, and weight of the cylinders were measured in duplicate. The bulk density (ρS) was calculated by the ratio between the sample mass and its volume obtained by its size. The porosity was calculated using EquationEquation (2)(2)
(2) (Zhang et al. Citation2014):
where ρS is the density of a similar solid material, in this case, cellulose for non-silanized samples, and ρSM is a mean between the density of the cellulose and the polymethylsiloxane for silanized samples.
The ρSM density was calculated with the EquationEquations (3)(3)
(3) and (Equation4
(4)
(4) ) (Zhang et al. Citation2014):
where wCELLULOSE is the mass fraction of cellulose in the sample and wPOLI(MTMS) is the mass fraction of polymethylsiloxane. The ρCELLULOSE was fixed at 1500 kg m−3 and ρPOLI(MTMS) at 1900 kg.m−3, according to the literature (Zhang et al. Citation2014). wPOLI(MTMS) was estimated with the EquationEquation (5)(5)
(5) :
where wS and wNFC are the dry mass of the sample and the portion of cellulose nanofibrils in the sample, respectively. All measurements were performed in duplicate and the HSD Tukey’s test was made at 5% level of significance.
Scanning electron microscopy (SEM)
Fragments of the aerogels were mounted on stubs, covered with a thin layer of gold in an Emitech K550 metallic cover apparatus, analyzed in an SEM Inspect-50 and Vega 3 Tescan, and visualized under a 15 kV voltage acceleration at different magnifications (1.000x and 20.000x). The diameter of 15 clearly visible fibers was measured using the ImageJ image analysis software (version 1.52a, Wayne Hasband, National Institute of Health, USA). The freeware Gimp 2.6 (version 2.10.14, https://www.gimp.org/) was used to make the figure.
Contact angle
The contact angle values were measured according to the ASTM D-5725-99 (2003). Droplets of deionized water were deposited on the surface of the previously conditioned (25°C, under vacuum, 24 h) aerogels with a syringe coupled to the contact angle measurement system. The images were captured by a Nikon camera shortly after the formation of the drop. The angle obtained on the surface of the aerogel was calculated by the Angle Calculator program. The freeware Gimp 2.6 (version 2.10.14, https://www.gimp.org/) was used to make the figure. All measurements were performed at 25 ± 1°C, with three replicates and the HSD Tukey’s test was made at 5% level of significance.
Mechanical compression tests
The compression tests were performed in a universal EMIC DL30000 mechanical test machine with a 100 kN load cell. The aerogel samples were cut in 12 mm height and 25 mm diameter pellets and arranged in a pair of fixed platters with a diameter of 110 mm. The compression speed was 1 mm·min−1. The mechanical properties of the aerogels were evaluated using compression tests up to 50% deformation, based on the initial thickness of the sample. The shape recovery capacity was also evaluated, based on the initial and final thickness (Zhang et al. Citation2014). The tests were repeated twice and the HSD Tukey’s test was made at 5% level of significance.
Absorption capacity
A volume of 20 mL of different liquids (ethanol, acetone, toluene, chloroform, mineral oil, and engine oil) was used to immerse the aerogels in a 50 mL beaker at room temperature (25 ± 1 °C). After the immersion for at least 1 min, the aerogel was removed, the excess or non-adsorbed liquid was removed with filter paper, and the sample was weighed. A total of three replications per sample were obtained. The tested aerogels had an average weight of 20 mg. The absorption capacity (CAB) was calculated by the EquationEquation (6)(6)
(6) :
where m0, and mS are respectively the mass of dry aerogel and after saturation. This analysis was done in triplicate (Zhang et al. Citation2014) and the HSD Tukey’s test was made at 5% level of significance.
A cycle absorption assay was performed using ethanol (Pereira et al. Citation2020). The same procedure of absorption was used, followed by drying the saturated aerogel in an oven at 60°C for 1 h. After new weighting, the second cycle of absorption/drying begins. The aerogels were submitted to seven absorption/drying cycles. Graphics were generated in OriginPro9.1 and modified in freeware Gimp 2.6 (version 2.10.14, https://www.gimp.org/).
Results and discussion
TEMPO-oxidized nanofibrillated cellulose
Both BC and BCP purified membranes were deconstructed with a Vitamix blender, resulting in pastes. The BC () was lighter and thicker than the BCP (), resembling the viscosity and color of the EC, a commercial product received as a nanofibrillated purified cellulose paste (). All materials presented oxidation yields around 80% after the TEMPO treatment and oxidation degrees of 0.25. The carboxylate group content ranged from 1.5 COO-∙g−1 cellulose for BCP and EC to 1.6 COO1-∙g−1 cellulose for BC (Pereira et al. Citation2020), in agreement with the range of reported values (1.2–1.7 COO1-∙g−1 cellulose) (Isogai, Saito, and Fukuzumi Citation2011). These results indicate that the different cellulose sources presented low influence for the carboxylate group content after TEMPO oxidation.
Figure 1. (A) Bacterial cellulose from HS medium (BC) deconstructed paste, (b) bacterial cellulose from cashew juice permeated (BCP) deconstructed paste, and (c) eucalyptus nanocellulose paste. Nanofibrillated oxidized BC (d), BCP (e), and EC (f) hydrogels.
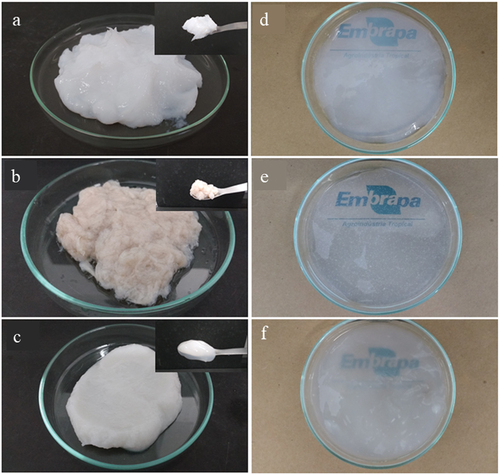
About 500 g of oxidized BC and BCP suspensions, at a concentration of 1% (w/w), were fibrillated with a Vitamix blender at maximum rotation for 30 min. EC was resuspended in the same conditions. The obtained materials were translucent and slightly white hydrogels (). After the silanization step, the suspensions of oxidized nanocellulose did not change in appearance but became less viscous. The flowchart () represents all the process steps.
Obtaining the bacterial cellulose aerogel
After lyophilization, BC, BCP, and EC aerogel monoliths were obtained (). The BCP is very similar to the BC aerogel, with no cracks, not very soft, and low brittleness. EC was obtained with cracks, but the non-collapsed region is as brittle as the BC aerogel. This is indicative of fractures during the freezing process.
Scanning electron microscopy (SEM)
The BCP aerogel has the lamellar configuration of the BC aerogel [33], but the lamellae are smaller (). At the edges of the structures, it is possible to see that they are made up of fibers organized in webs (), which were previously identified in BC aerogels (Zhang et al. Citation2014, Citation2015). EC aerogel is similar to BC aerogel, consisting of lamellae and fibers, but of smaller lamellae (). There are spherical clusters in (), resulting from the condensation of the polymethylsiloxane (Zhang et al. Citation2015). This behavior was also reported by Sai et al. (Citation2014) (Sai et al. Citation2014), who functionalized cellulose with Na2SiO3 and MTMS. In the work reported by Zhang et al. (Citation2015) (Zhang et al. Citation2015), the emergence of polymethylsiloxane condensates is linked to the spatial concentration of reagents, but in this work, the raw material seems to have a higher influence. This behavior was not seen in BC or BCP aerogel samples.
Aerogels produced from both types of bacterial cellulose substrate showed a lamellar configuration (). It seemed that the lamellae from the BCP aerogel were smaller than that of BC. It is noticeable at the edge of the lamellae that they presented fibers organized in webs, which were previously identified by Zhang et al. (Citation2014, Citation2015).
Aerogels made of EC appears to be more compact, as if the microfibrils have irreversibly collapsed, as in the hornification process. There are spherical clusters on the cellulose, resulting from the condensation of the polymethylsiloxane (Sai et al. Citation2014; Zhang et al. Citation2015). The EC was produced by a plant cellulose synthase. Differences in the enzymatic machinery between eukaryotes and prokaryotes result in types of cellulose with different structures, impacting, for example, the nanofibril width, crystallinity degree, and cellulose allomorph content (Hussain et al. Citation2019; Klemm et al. Citation2011; Sacui et al. Citation2014). The bacterial cellulose is assembled without hemicellulose or lignin and the water incorporated within the net of bacterial cellulose stabilizes the 3D structure of fibrils and pores (Klemm et al. Citation2011). The pores formed in the EC were created during the freeze-drying. The pores in the BC hydrogel may have a more uniform distribution than the EC hydrogel, resulting in the micromorphology differences, without collapsing.
The comparison between the three types of aerogels indicated that although the three materials have the same chemical composition, the cellulose fibrils organization is different. The different visibility of the open web of submicron nanofibers for the aerogels (diameter of 140–156 nm for BC fibers, 162–237 nm for BCP fibers, and 272–337 nm for EC fibers) is indicative that the size distribution for the pores may vary as a function of the original biomass, impacting the absorption efficiency for the aerogels. In general, the aerogel samples of BCP and EC have smaller structures, which may result in more porous aerogels.
Published articles indicate that the native structure of bacterial cellulose may have different characteristics based on the cultivation conditions, such as crystallinity (Hussain et al. Citation2019; Jozala et al. Citation2015). Cellulose production is a complex process, from the intracellular synthesis of polyglucose building blocks to the secretion of ß-1,4-glucan chains (Ross et al. Citation1991). Many genes related to enzymatic synthesis and regulation are involved in cellulose metabolism (Huang et al. Citation2020; Jang et al. Citation2019; Römling and Galperin Citation2015). K. xylinus is a versatile bacterial cellulose producer, which can be grown using more than two dozens of different sole carbon sources in the growth medium. Changes in the available nutrients change the genetic expression, impacting metabolic pathways from the tricarboxylic acid cycle to the bacterial cellulose synthase (Jang et al. Citation2019).
We hypothesize that macro and micronutrients in the BCP, not present in the synthetic medium HS, altered the genetic expression related to cellulose synthesis. These alterations may have changed the enzymatic efficiency, or the number of expressed enzymes, or even the structure of the enzymes. These differences do not impact major properties of bacterial cellulose from both media but may alter the microstructure of the fibril/pore networks, as detected on the micromorphology of different aerogels. These findings should be further investigated with a functional genomic analysis of the bacteria cultivated in different growth media to better understand the genetic impact on the final product.
Fourier transform infrared absorption spectroscopy (FTIR)
The FTIR analyses were performed to confirm the carboxylation as observed in the conductometric titration and the silanization of the materials. The shows bands at 1726 and 1623 cm−1 assigned to the presence of -COOH and -COO-, respectively. The bands at 780, 911, and 1275 cm−1 show the presence of chemical bonds with the silane (Chen et al. Citation2019; Jiang and Lo Hsieh Citation2016; Xiao et al. Citation2015; Zhang et al. Citation2015), confirming the successful double-functionalization of the different types of cellulose (). The peak transmittance at 780 cm−1 may represent the presence of Si ― O ― Si bonds, which would result in polymethylsiloxane chains, or Si ― C bonds, from methyl groups. The peak at 911 cm−1 denotes Si ― OH bonds or Si ― C bonds. The peak at 1275 cm−1 is attributed to the presence of CH3 groups from polymethylsiloxane.
Figure 4. FTIR spectra of oxidized-silanized bacterial cellulose from HS medium (BC), from cashew juice permeated (BCP), and from eucalyptus nanocellulose (EC) aerogels.
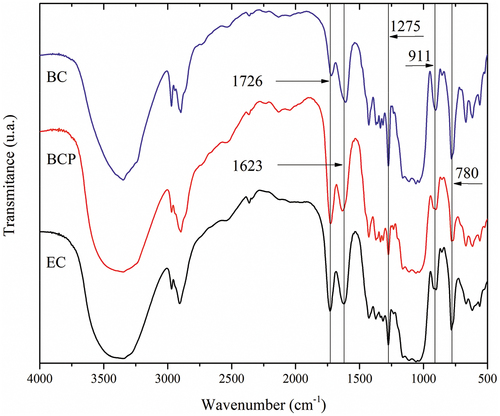
The presence of these five bands in the materials BC, BCP, and EC, in comparison with the untreated cellulose (Pereira et al. Citation2020), indicates that the double chemical functionalization was successfully achieved. Nevertheless, just the presence of silicon and carboxylic groups in the samples will not guarantee that the three different materials have the same mechanical and absorptive properties, and other analyses were performed.
Porosity, density, and mechanical tests of aerogels
The BCP and EC aerogels are very similar to the BC aerogel (Pereira et al. Citation2020), as shown in , demonstrating that the method can be applied successfully in different cellulose sources. The aerogels obtained have similar density and deformation. Aerogels also suffered a greater shrinkage but within the limit of 30% (Aegerter, Leventis, and Koebel Citation2011). The values for apparent density, and porosity were very close to those reported in similar works: 11 kg·m−3 and 99.3% (Zhang et al. Citation2014), 6.18–16.39 kg·m−3 and 98.98–99.58% (Chen et al. Citation2019), 3.1–7.9 kg·m−3 and 99.48–99.81% (Laitinen et al. Citation2017), 11 kg·m−3 and 99.4% (Sai et al. Citation2014). It is interesting to highlight that the porosity within treatments is so uniform that even a difference of 0.1% is statistically significant (), indicating that this parameter has low variability. This is an indication that the different types of aerogels are not fully identical. When other mechanical properties are evaluated, the differences become more evident.
Table 1. Average values of apparent density, porosity, shrinkage, maximum compression stress at 50% deformation (σMAX, ε50%), elasticity modulus (E) and final deformation after 24 h of the compression test (εFINAL) of BC (bacterial cellulose from the synthetic medium HS), BCP (bacterial cellulose from cashew juice permeate), and EC (eucalyptus nanocellulose) aerogels.
Compression tests were performed up to 50% deformation to evaluate the mechanical properties of the aerogels. The capacity to recover shape was also measured. The values for the maximum stress in the deformation of 50% and the modulus of elasticity were similar for the BCP and EC aerogels, and both values are smaller than those observed for BC. Samples of the aerogel made of plant cellulose and BC from the alternative source have less mechanical resistance but they can still recover about 85% of their initial shape. The tension values in this work () were in the range of those reported in similar works: 4 kPa (Zhang et al. Citation2014) and 10 kPa (Nguyen et al. Citation2014). The deformation recovery is close to the value observed by Zhang et al. (Citation2014) (Zhang et al. Citation2014) (86%) and higher than that reported by Nguyen et al. (Citation2014)(50%).
The parameters with no statistical difference may be related to the chemical composition of the aerogels. The different types of cellulose were successfully modified by the proposed chemical double-functionalization (Section 3.1). Nevertheless, differences in the microstructure of aerogels () impact the mechanical properties, reinforcing the impact that the raw material has on the final product.
Contact angle
The BCP aerogel (126.8° ± 1.7°) is more hydrophobic than the BC aerogel (120.0° ± 2.5°) (Pereira et al. Citation2020) and the EC aerogel (129.6° ± 0.8°) is the most hydrophobic (). According to Wenzel’s Law for wettability of a material, the rougher the surface, the greater the hydrophobicity (Sai et al. Citation2014). This can be understood in terms of surface area. The BCP and EC aerogels have a larger area, as they have much smaller structures than the large, flat lamellas of BC aerogel. Furthermore, the silane deposition on the fibers () for the EC aerogel increases the roughness of the surface, having a higher impact on the hydrophobicity. The methods/processes used to obtain the aerogel can be applied for different cellulose sources, but the raw material can influence the properties of the double-functionalized aerogel. The observed values of contact angle () were similar to those reported by other authors: 117.6°-124.6° (Sai et al. Citation2014), 136° (Zhang et al. Citation2014), and 139° (Wan et al. Citation2015).
Figure 5. Average values of the contact angle between water and aerogel samples and test images. Means followed by a common letter are not significantly different by the HSD Tukey’s test at 5% level of significance. BC: bacterial cellulose from the synthetic medium HS, BCP: bacterial cellulose from cashew juice permeate, EC: eucalyptus nanocellulose.
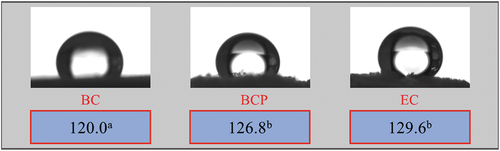
Absorption tests
BCP, EC, and BC aerogels (Pereira et al. Citation2020) have different absorption performances (). The EC aerogel absorbs a greater amount of organic solvents but does not show the same behavior for oils (acetone = 58 ± 3%, ethanol = 59 ± 4%, mineral oil = 41 ± 6%, motor oil = 45 ± 2%, toluene = 55 ± 7%, chloroform = 82 ± 1%). The BCP aerogel absorbs the same amount of acetone and ethanol, a lower amount of toluene and chloroform, and a higher amount of oil (acetone = 42 ± 3%, ethanol = 44 ± 2%, mineral oil = 65 ± 4%, motor oil = 67 ± 3%, toluene = 45 ± 3%, chloroform = 70 ± 3%) than the BC (acetone = 40 ± 1%, ethanol = 45 ± 2%, mineral oil = 36 ± 3%, motor oil = 52 ± 6%, toluene = 35 ± 2%, chloroform = 77 ± 1%). The BCP and EC aerogels absorb almost the same amount of chloroform, demonstrating that for this liquid the double functionalization works regardless of the raw cellulosic material. The order of toluene absorption follows the order of hydrophobicity, EC> BCP> BC.
Figure 6. a) Absorption capacity of BC aerogels for different organic oils/solvents (means followed by the same lowercase letter for each type of solvent are not significantly different by the HSD Tukey’s test at 5% level of significance), and b) Cycles of ethanol absorption/drying of aerogels. BC: bacterial cellulose from synthetic medium, BCP: bacterial cellulose from cashew juice permeate, EC: eucalyptus nanocellulose.
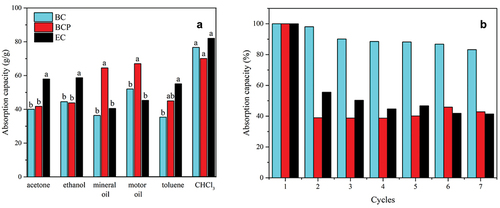
EC aerogel is hydrophobic, but it is not as oleophilic as BCP aerogel, which absorbs a smaller amount of organic solvents. Although cellulose is amphiphilic, and double functionalization is applied in the same way for all of the raw materials, the cellulose source plays an important role in the oleophilicity of the aerogel. The three types of aerogels demonstrated different profiles of absorption behavior and there may be a different optimum application for each one. For example, to clean up motor or mineral oils, BCP is the best. For toluene, the EC aerogel is more appropriate. The absorption capacity values () were close to those reported in similar works: 12 and 22 times (ethanol and chloroform) (Cheng et al. Citation2017), 40 to 60 times (ethanol, acetone, and motor oil) (Jin et al. Citation2015), 90–150 times (ethanol, acetone, toluene, chloroform, and motor oil) (Laitinen et al. Citation2017) and 49–102 times (ethanol, acetone, toluene, chloroform, motor oil, and mineral oil) (Zhang et al. Citation2014). This is another evidence indicating that the choice of the raw material impacts the physical properties of the aerogel and may affect the final product.
The capacity for several absorption-drying cycles of the aerogel was evaluated (). In comparison with the BC aerogel (Pereira et al. Citation2020), the BCP and EC aerogels have their capacity reduced to 40 and 55% already in the second cycle, respectively. The absorption for EC has the first decay to 55% and it continues to decline until 45%. BCP has a greater decay than EC, declining the absorption to 40%. The great shrinkage in drying is an indication that the microscopic structures collapse easily, but the macroscopic structures are maintained throughout the cycles, preserving around 45% of the initial absorption capacity.
Conclusion
Double-functionalized aerogels were successfully obtained using the oxidation-silanization approach to three different cellulosic raw materials: eucalyptus nanocellulose (EC), bacterial cellulose from HS synthetic medium (BC), and cashew juice permeate (BCP). EC and BCP have similar chemical composition, resistance to deformation, and density to the BC aerogel. The EC and BCP aerogels have lower mechanical resistance to stress than BC but higher absorption capacities for acetone and methanol (EC), and oils (BCP).
Considering the properties analyzed, different types of aerogels may have different potential uses. For example, BC aerogels can be applied in situations that demand robustness, BCP aerogel for oil spills, and EC aerogels for recovering organic solvents. These results are indicative that the cellulose origin impacts the mechanical and absorption properties of the obtained aerogels. On the one hand, the properties of the final product may demand a careful control of the raw material when the aerogel is produced, but on the other hand, the possibility to generate different products with the same chemical functionalization may expand the technological application for different natural materials.
Highlights
Bacterial cellulose aerogels had the best mechanical properties.
Aerogels from 3 different types of cellulose materials were double-functionalized.
The cellulose type impacts on mechanical and absorption properties of the aerogels.
Data availability
The raw/processed data required to reproduce these findings cannot be shared at this time due to technical or time limitations.
Ethical approval
The authors declare that they have no known competing financial interests or personal relationships that could have appeared to influence the work reported in this paper. We confirm that the manuscript, or its contents in some other forms, has not been submitted or published previously by any of the authors and is not under consideration for publication in another journal. All authors have seen and approved the submission of the manuscript.
Acknowledgments
The authors gratefully acknowledge the financial support of the Empresa Brasileira de Pesquisa Agropecuária (Embrapa, 02.14.03.012.00.00 and 03.14.04.007.00.00) and the Ceará Foundation for the Support of Scientific and Technological Development (FUNCAP, PR2-0101-00023.01.00/15), and Celli R. Muniz (Embrapa Agroindustria Tropical) and Analytical Center of Universidade Federal do Ceará (UFC) for the SEM analyses. They also thank CAPES (2017SLR-17925) for the scholarship granted to Pereira. Feitosa thanks INCT-INOMAT. Author Rosa thanks CNPq for their Research Productivity fellowships (305504/2016-9). They also thank Suzano Pulp and Paper for the nanofibrilated eucalyptus cellulose sample.
Disclosure statement
No potential conflict of interest was reported by the author(s).
References
- Abouzeid, R. E., R. Khiari, N. El-Wakil, and A. Dufresne. 2019. Current state and new trends in the use of cellulose nanomaterials for wastewater treatment. Biomacromolecules 20 (2):573–15. doi:10.1021/acs.biomac.8b00839.
- Aegerter, M., N. Leventis, and M. Koebel. 2011. Aerogels handbook (advances in sol-gel derived materials and technologies). New York: Springer. http://link.springer.com/content/pdf/10.1007/978-1-4419-7589-8.pdf%5Cnhttp://books.google.com/books?hl=en&lr=&id=3FXWzJFOlnAC&oi=fnd&pg=PA2&dq=Aerogels+Handbook&ots=MbUN5K6W9d&sig=5wg6moGTmfyVqBaD9YiAgvtub6I.
- BARROS, M. D. O. 2016. Avaliação do permeado DE caju como meio DE cultivo alternativo para a produção DE celulose bacteriana. Instituto Federal DE Educação, Ciência E Tecnologia Do Estado Do Ceará 1: 42.
- Beh, J. H., T. H. Lim, J. H. Lew, and J. C. Lai. 2020. Cellulose nanofibril-based aerogel derived from sago pith waste and its application on methylene blue removal. International Journal of Biological Macromolecules 160: 160. doi: 10.1016/j.ijbiomac.2020.05.227.
- Besbes, I., S. Alila, and S. Boufi. 2011. Nanofibrillated cellulose from TEMPO-oxidized eucalyptus fibres: effect of the carboxyl content. Carbohydrate Polymers 84 (3):975–83. doi:10.1016/j.carbpol.2010.12.052.
- Boukind, S., S. Sair, H. Ait Ousaleh, S. Mansouri, M. Zahouily, Y. Abboud, and A. El Bouari. 2021. Ambient pressure drying as an advanced approach to the synthesis of silica aerogel composite for building thermal insulation. Journal of Natural Fibers 29:1–15. October. doi:10.1080/15440478.2021.1993486.
- Cheng, H., B. Gu, M. P. Pennefather, T. X. Nguyen, N. Phan-Thien, and H. M. Duong. 2017. Cotton aerogels and cotton-cellulose aerogels from environmental waste for oil spillage cleanup. Materials & Design 130 (May):452–58. doi:10.1016/j.matdes.2017.05.082.
- Chen, Y., S. Yang, D. Fan, G. Li, and S. Wang. 2019. Dual-enhanced hydrophobic and mechanical properties of long-range 3D anisotropic binary-composite nanocellulose foams via bidirectional gradient freezing. ACS Sustainable Chemistry & Engineering 7 (15):12878–86. doi:https://doi.org/10.1021/acssuschemeng.9b01806.
- Chen, W., H. Yu, Q. Li, Y. Liu, and J. Li. 2011. Ultralight and highly flexible aerogels with long cellulose i nanofibers. Soft Matter 7 (21):10360–68. doi:http://dx.doi.org/10.1039/C1SM06179H.
- de Amorim, J. D. P., K. C. de Souza, C. R. Duarte, I. da Silva Duarte, F. de Assis Sales Ribeiro, G. S. Silva, P. M. A. de Farias, A. Stingl, A. F. S. Costa, G. M. Vinhas, et al. 2020. Plant and bacterial nanocellulose: Production, properties and applications in medicine, food, cosmetics, electronics and engineering. a review. Environmental Chemistry Letters. 18(3):851–69. doi:10.1007/s10311-020-00989-9.
- De Azeredo, H. M. C. 2009. Nanocomposites for food packaging applications. Food Research International 42 (9):1240–53. doi:http://dx.doi.org/10.1016/j.foodres.2009.03.019.
- Dufresne, A. 2012. Nanocellulose: From nature to high performance tailored materials. De Gruyter Textbook. De Gruyter. https://books.google.com.br/books?id=xa56tgAACAAJ.
- Fan, P., Y. Yuan, J. Ren, B. Yuan, Q. He, G. Xia, F. Chen, and R. Song. 2017. Facile and green fabrication of cellulose based aerogels for lampblack filtration from waste newspaper. Carbohydrate Polymers 162:108–14. doi:http://dx.doi.org/10.1016/j.carbpol.2017.01.015.
- Feng, J., S. T. Nguyen, Z. Fan, and H. M. Duong. 2015. Advanced fabrication and oil absorption properties of super-hydrophobic recycled cellulose aerogels. Chemical Engineering Journal 270:168–175. doi:10.1016/j.cej.2015.02.034 https://linkinghub.elsevier.com/retrieve/pii/S1385894715002326 .
- Garcia-Bordejé, E., A. M. Benito, and W. K. Maser. 2021. Graphene aerogels via hydrothermal gelation of graphene oxide colloids: fine-tuning of its porous and chemical properties and catalytic applications. Advances in Colloid and Interface Science 292:102420. doi:10.1016/j.cis.2021.102420.
- Guan, Y., J. Rao, Y. Wu, H. Gao, S. Liu, G. Chen, and F. Peng. 2020. Hemicelluloses-based magnetic aerogel as an efficient adsorbent for Congo red. International Journal of Biological Macromolecules 155:369–75. doi:10.1016/j.ijbiomac.2020.03.231.
- Heath, L., and W. Thielemans. 2010. Cellulose Nanowhisker Aerogels. Green Chemistry 12 (8):1448. doi:10.1039/c0gc00035c.
- Hosseini, H., M. Teymouri, S. Saboor, A. Khalili, V. Goodarzi, F. Poudineh Hajipoor, H. A. Khonakdar, S. Shojaei, A. Asefnejad, and H. Bagheri. 2019. Challenge between sequence presences of conductive additives on flexibility, dielectric, and supercapacitance behaviors of nanofibrillated template of bacterial cellulose aerogels. European Polymer Journal 115 (March):335–45. doi:10.1016/j.eurpolymj.2019.03.033.
- Hosseini, H., A. Zirakjou, V. Goodarzi, S. M. Mousavi, H. A. Khonakdar, and S. Zamanlui. 2020. Lightweight aerogels based on bacterial cellulose/silver nanoparticles/polyaniline with tuning morphology of polyaniline and application in soft tissue engineering. International Journal of Biological Macromolecules 152:57–67. doi:10.1016/j.ijbiomac.2020.02.095.
- Huang, C., H. Ji, Y. Yang, B. Guo, L. Luo, Z. Meng, L. Fan, and J. Xu. 2020. TEMPO-oxidized bacterial cellulose nanofiber membranes as high-performance separators for lithium-ion batteries. Carbohydrate Polymers 230:115570. doi:https://doi.org/10.1016/j.carbpol.2019.115570.
- Hussain, Z., W. Sajjad, T. Khan, and F. Wahid. 2019. Production of bacterial cellulose from industrial wastes: A review. Cellulose 26 (5):2895–911. doi:10.1007/s10570-019-02307-1.
- Isogai, A., and Y. Kato. 1998. Preparation of polyuronic acid from cellulose by TEMPO-mediated oxidation. Cellulose February. 5(33):153–64. doi: 10.1023/A:1009208603673.
- Isogai, A., T. Saito, and H. Fukuzumi. 2011. TEMPO-oxidized cellulose nanofibers. Nanoscale 3 (1):71–85. doi:10.1039/C0NR00583E.
- Jang, W. D., T. Y. Kim, H. U. Kim, W. Y. Shim, J. Y. Ryu, J. H. Park, and S. Y. Lee. 2019. Genomic and metabolic analysis of komagataeibacter xylinus DSM 2325 producing bacterial cellulose nanofiber. Biotechnology and Bioengineering 116 (12):3372–81. doi:10.1002/bit.27150.
- Jiang, F., and Y. Lo Hsieh. 2016. Self-assembling of TEMPO oxidized cellulose nanofibrils as affected by protonation of surface carboxyls and drying methods. ACS Sustainable Chemistry & Engineering 4 (3):1041–49. doi:10.1021/acssuschemeng.5b01123.
- Jin, C., S. Han, J. Li, and Q. Sun. 2015. Fabrication of cellulose-based aerogels from waste newspaper without any pretreatment and their use for absorbents. Carbohydrate Polymers 123:150–56. doi:http://dx.doi.org/10.1016/j.carbpol.2015.01.056.
- Jozala, A. F., R. A. N. Pértile, C. A. dos Santos, V. de Carvalho Santos-Ebinuma, M. M. Seckler, F. M. Gama, and A. Pessoa. 2015. Bacterial cellulose production by gluconacetobacter xylinus by employing alternative culture media. Applied Microbiology and Biotechnology 99 (3):1181–90. doi:10.1007/s00253-014-6232-3.
- Klemm, D., F. Kramer, S. Moritz, T. Lindström, M. Ankerfors, D. Gray, and A. Dorris. 2011. Nanocelluloses: A new family of nature-based materials. Angewandte Chemie International Edition 24 (24):5438–66. doi:10.1002/anie.201001273.
- Kvien, I., and K. Oksman. 2007. Orientation of cellulose nanowhiskers in polyvinyl alcohol. Applied Physics A, Materials Science & Processing 87 (4):641–43. doi:10.1007/s00339-007-3882-3.
- Laitinen, O., T. Suopajärvi, M. Österberg, and H. Liimatainen. 2017. Hydrophobic, superabsorbing aerogels from choline chloride-based deep eutectic solvent pretreated and silylated cellulose nanofibrils for selective oil removal. ACS Applied Materials & Interfaces 9 (29):25029–37. doi:10.1021/acsami.7b06304.
- Motta Neves, R., K. Silveira Lopes, M. G. V. Zimmermann, M. Poletto, and A. J. Zattera. 2020. Cellulose nanowhiskers extracted from tempo-oxidized curaua fibers. Journal of Natural Fibers 17 (9):1355–65. doi:10.1080/15440478.2019.1568346.
- Nguyen, S. T., J. Feng, S. K. Ng, J. P. W. Wong, V. B. C. Tan, and H. M. Duong. 2014. Advanced thermal insulation and absorption properties of recycled cellulose aerogels. Colloids and Surfaces A, Physicochemical and Engineering Aspects 445:128–34. doi:10.1016/j.colsurfa.2014.01.015
- Nyakuma, B. B., S. Wong, L. N. Utume, T. A. T. Abdullah, M. Abba, O. Oladokun, T. J. P. Ivase, and E. B. Ogunbode. 2021. Comprehensive characterisation of the morphological, thermal and kinetic degradation properties of gluconacetobacter xylinus synthesised bacterial nanocellulose. Journal of Natural Fibers 00 (00):1–14. doi:10.1080/15440478.2021.1907833.
- Pereira, A. L. S., J. P. A. Feitosa, J. P. S. Morais, and M. de F Rosa. 2020.Bacterial cellulose aerogels: Influence of oxidation and silanization on mechanical and absorption properties. Carbohydrate Polymers 250: 250.doi: 10.1016/j.carbpol.2020.116927.
- Piperopoulos, E., A. Khaskhoussi, V. Fiore, and L. Calabrese. 2021. Surface modified Arundo donax natural fibers for oil spill recovery. Journal of Natural Fibers September. 6:1–16. doi: 10.1080/15440478.2021.1961343.
- Poddar, M. K., and P. K. Dikshit. 2021. Recent development in bacterial cellulose production and synthesis of cellulose based conductive polymer nanocomposites. Nano Select 2 (9):1605–28. doi:10.1002/nano.202100044.
- Rathinamoorthy, R., T. Aarthi, C. A. Aksaya Shree, P. Haridharani, V. Shruthi, and R. L. Vaishnikka. 2021. Development and characterization of self-assembled bacterial cellulose nonwoven film. Journal of Natural Fibers 18 (11):1857–70. doi:10.1080/15440478.2019.1701609.
- Rathinamoorthy, R., and T. Kiruba. 2020. Bacterial cellulose-A potential material for sustainable eco-friendly fashion products. Journal of Natural Fibers 00 (00):1–13. doi:10.1080/15440478.2020.1842841.
- Rivas, B., A. B. Moldes, J. M. Domínguez, and J. C. Parajó. 2004. Development of culture media containing spent yeast cells of debaryomyces hansenii and corn steep liquor for lactic acid production with lactobacillus rhamnosus. International Journal of Food Microbiology 97 (1):93–98. doi:10.1016/j.ijfoodmicro.2004.05.006.
- Römling, U., and M. Y. Galperin. 2015. Bacterial cellulose biosynthesis: diversity of operons, subunits, products, and functions. Trends in Microbiology 23 (9):545–57. doi:10.1016/j.tim.2015.05.005.
- Ross P, Mayer R and Benziman M. 1991. Cellulose biosynthesis and function in bacteria. Microbiological Reviews, 55(1),35–58. doi:10.1128/MMBR.55.1.35-58.1991.
- Sacui, I. A., R. C. Nieuwendaal, D. J. Burnett, S. J. Stranick, M. Jorfi, C. Weder, E. J. Foster, R. T. Olsson, and J. W. Gilman. 2014. Comparison of the properties of cellulose nanocrystals and cellulose nanofibrils isolated from bacteria, tunicate, and wood processed using acid, enzymatic, mechanical, and oxidative methods. ACS Applied Materials & Interfaces 6 (9):6127–38. doi:10.1021/am500359f.
- Saito, T., and A. Isogai. 2004. TEMPO-mediated oxidation of native cellulose. the effect of oxidation conditions on chemical and crystal structures of the water-insoluble fractions. Biomacromolecules 5 (5):1983–89. doi:10.1021/bm0497769.
- Saito, T., S. Kimura, Y. Nishiyama, and A. Isogai. 2007. Cellulose nanofibers prepared by TEMPO-mediated oxidation of native cellulose. Biomacromolecules 8 (8):2485–91. doi:10.1021/bm0703970.
- Sai, H., L. Xing, J. Xiang, L. Cui, J. Jiao, C. Zhao, Z. Li, F. Li, and T. Zhang. 2014. Flexible aerogels with interpenetrating network structure of bacterial cellulose–silica composite from sodium silicate precursor via freeze drying process. RSC Advances 4 (57):30453. doi:10.1039/C4RA02752C.
- Schramm, M., and S. Hestrin. 1954. Factors affecting production of cellulose at the air/liquid interface of a culture of acetobacter xylinum. Journal of General Microbiology August. 11(1):123–29. doi: 10.1099/00221287-11-1-123.
- Sharma, A., M. Thakur, M. Bhattacharya, T. Mandal, and S. Goswami. 2019. Commercial application of cellulose nano-composites – a review. Biotechnology Reports 21:e00316. doi:10.1016/j.btre.2019.e00316.
- Takeshita, S., S. Zhao, W. J. Malfait, and M. M. Koebel. 2021. Chemistry of chitosan aerogels: Three-dimensional pore control for tailored applications. Angewandte Chemie International Edition 60 (18):9828–51. doi:10.1002/anie.202003053.
- Tanpichai, S., and E. Wimolmala. 2021. Facile single-step preparation of cellulose nanofibers by TEMPO-mediated oxidation and their nanocomposites. Journal of Natural Fibers (November 9):1–17. doi:10.1080/15440478.2021.1993483.
- Wang, Z., S. Wu, Y. Zhang, L. Miao, Y. Zhang, and A. Wu. 2020. Preparation of modified sodium alginate aerogel and its application in removing lead and cadmium ions in wastewater. International Journal of Biological Macromolecules 157:687–94. doi:10.1016/j.ijbiomac.2019.11.228.
- Wan, C., Y. Lu, Y. Jiao, C. Jin, Q. Sun, and J. Li. 2015. Ultralight and hydrophobic nanofibrillated cellulose aerogels from coconut shell with ultrastrong adsorption properties. Journal of Applied Polymer Science 132 (24):n/a–n/a. doi:10.1002/app.42037.
- Wu, C.-N., and K.-C. Cheng. 2017. Strong, thermal-stable, flexible, and transparent films by self-assembled TEMPO-oxidized bacterial cellulose nanofibers. Cellulose 24 (1):269–83. doi:10.1007/s10570-016-1114-8.
- Wu, C. N., S. C. Fuh, S. P. Lin, Y. Y. Lin, H. Y. Chen, J. M. Liu, and K. C. Cheng. 2018. TEMPO-oxidized bacterial cellulose pellicle with silver nanoparticles for wound dressing. Biomacromolecules 19 (2):544–54. doi:10.1021/acs.biomac.7b01660.
- Wu, Q., J. Hu, S. Cao, S. Yu, and L. Huang. 2020. Heteroatom-doped hierarchical porous carbon aerogels from chitosan for high performance supercapacitors. International Journal of Biological Macromolecules 155:131–41. doi:10.1016/j.ijbiomac.2020.03.202.
- Xiao, S., R. Gao, Y. Lu, J. Li, and Q. Sun. 2015. Fabrication and characterization of nanofibrillated cellulose and its aerogels from natural pine needles. Carbohydrate Polymers 119:202–09. doi:10.1016/j.carbpol.2014.11.041.
- Yousefi, H., M. Faezipour, S. Hedjazi, M. M. Mousavi, Y. Azusa, and A. H. Heidari. 2013. Comparative study of paper and nanopaper properties prepared from bacterial cellulose nanofibers and fibers/ground cellulose nanofibers of canola straw. Industrial Crops and Products 43 (1):732–37. doi:http://dx.doi.org/10.1016/j.indcrop.2012.08.030.
- Zhang, Z., G. Sèbe, D. Rentsch, T. Zimmermann, and P. Tingaut. 2014. Ultralightweight and flexible silylated nanocellulose sponges for the selective removal of oil from water. Chemistry of Materials 26 (8):2659–68. doi:10.1021/cm5004164.
- Zhang, Z., P. Tingaut, D. Rentsch, T. Zimmermann, and G. Sèbe. 2015. Controlled silylation of nanofibrillated cellulose in water: Reinforcement of a model polydimethylsiloxane network. ChemSuschem 8 (16):2681–90. doi:10.1002/cssc.201500525.