ABSTRACT
Lysine methylation is a frequent post-translational protein modification, which has been intensively studied in the case of histone proteins. Lysine methylations are also found on many non-histone proteins, and one prominent example is eukaryotic elongation factor 1 alpha (eEF1A). Besides its essential role in the protein synthesis machinery, a number of non-canonical functions have also been described for eEF1A, such as regulation of the actin cytoskeleton and the promotion of viral replication. The functional significance of the extensive lysine methylations on eEF1A, as well as the identity of the responsible lysine methyltransferases (KMTs), have until recently remained largely elusive. However, recent discoveries and characterizations of human eEF1A-specific KMTs indicate that lysine methylation of eEF1A can be dynamic and inducible, and modulates mRNA translation in a codon-specific fashion. Here, we give a general overview of eEF1A lysine methylation and discuss its possible functional and regulatory significance, with particular emphasis on newly discovered human KMTs.
Eukaryotic elongation factor 1 alpha (eEF1A)
Cellular protein synthesis consists of three distinct stages: initiation, elongation and termination, and all stages depend on distinct translation factors, which are typically highly conserved throughout evolution. Eukaryotic elongation factor 1 alpha (eEF1A) is a GTP-binding protein and a key component of the translational machinery (reviewed in [Citation1,Citation2]). In its GTP-bound state, eEF1A binds to aminoacylated tRNA, and mediates its transport to the A-site of the translating ribosome, thereby performing a crucial function during the elongation phase of translation (). Binding of eEF1A to the ribosome triggers hydrolysis of eEF1A-bound GTP, and the inactive GDP-bound form of eEF1A then leaves the ribosome. Recycling of the inactive eEF1A:GDP complex back to the active GTP-bound state is mediated by the eEF1B complex, which acts as a guanine nucleotide exchange factor (GEF) (). In simple eukaryotes, such as the yeast Saccharomyces cerevisiae, eEF1B consists of two subunits, α and γ, where eEF1Bα possesses the GEF activity. In higher eukaryotes, eEF1B usually comprises additional subunits [Citation2].
Figure 1. Canonical and non-canonical functions of eEF1A. During the elongation step of translation, ternary complexes of eEF1A, GTP and the different aminoacyl-tRNAs (aa-tRNA) are sampled by the ribosome. Upon productive base-pairing, the aa-tRNA is accommodated in the ribosome A-site while the eEF1A-bound GTP is hydrolyzed. eEF1A-GTP is regenerated through the guanosine exchange factor eEF1B. Non-canonical functions of eEF1A are indicated in the dashed box.
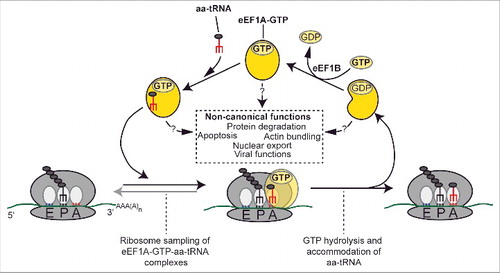
Structural studies have revealed that eEF1A consists of three domains, denoted I, II and III, which have been associated with distinct aspects of eEF1A function. Domain I binds GTP, and, together with domain II, mediates association with the GEF eEF1Bα [Citation3,Citation4]. The binding of tRNA has been primarily ascribed to Domain II, but comparison with the bacterial eEF1A orthologue EF-Tu suggests that all three domains are somewhat involved in tRNA binding [Citation5]. Mammals, as well as several other vertebrates, possess two eEF1A paralogues, denoted eEF1A1 and eEF1A2, which share a high degree of sequence similarity (92% identity in humans) [Citation6].
In addition to its well established and much studied function in protein synthesis, a number of non-canonical functions have been reported for eEF1A. These "moonlighting functions" are typically exerted by eEF1A alone, independent of the eEF1B complex. The most studied of these is the ability of eEF1A to interact with and modulate the cytoskeleton (reviewed in [Citation7]). eEF1A has been shown to be an actin-binding protein, capable of inducing actin bundling in vitro [Citation8,Citation9]. Correspondingly, eEF1A over-expression in S. cerevisiae causes disorganisation of the actin cytoskeleton [Citation10]. Several eEF1A mutants that were unable to interact with actin were still able to support protein synthesis, indicating that eEF1A's ability to interact with and modulate actin is a feature distinct from its canonical role in protein synthesis [Citation11]. A number of studies have reported that eEF1A, as well as its bacterial orthologue EF-Tu, promote and regulate the propagation of various RNA viruses. Many of these studies describe the binding of eEF1A to tRNA-like RNA structures in the 3' untranslated region (UTR) of the viral genomes, but eEF1A has also been shown to interact directly with viral proteins (reviewed in [Citation12]). Furthermore, several additional non-canonical roles for eEF1A have been indicated, e.g. in protein degradation, apoptosis and in nuclear transport [Citation4,Citation6].
eEF1A lysine methylation
eEF1A carries an abundance of post-translational modifications (PTMs), several of which are rather unusual. Ethanolamine-phosphoglycerol moieties are attached to two glutamic acid residues in mammalian eEF1A [Citation13,Citation14], whereas S. cerevisiae eEF1A carries a glutaminyl modification at a glutamic acid residue [Citation15]. Also, numerous phosphorylations have been reported, and some of these have been implicated in the regulation of eEF1A activity or stability [Citation16-18]. In addition, eEF1A has been reported to be methylated both at the N- and C-terminal ends [Citation19,Citation20]. However, the most striking feature of the PTM landscape of eEF1A is arguably the abundance of lysine methylation.
Many proteins carry methylated lysine residues. Each lysine can accept up to three methyl groups introduced by various lysine (K)-specific methyltransferases (KMTs) that use S-adenosylmethionine (AdoMet) as methyl donor, resulting in three possible methylation states: monomethylation (Kme1), dimethylation (Kme2) and trimethylation (Kme3) (). Lysine methylation of eEF1A was discovered in the dimorphic fungus Mucor racemosus already in 1982 [Citation21], and has since been studied in a variety of different organisms. In early studies that were primarily based on peptide sequencing, mammalian eEF1A was found to be methylated on five lysines, i.e. Lys-36, Lys-55, Lys-79, Lys-165 and Lys-318 () [Citation14]. Similarly, S. cerevisiae eEF1A was found to be methylated on four sites which partially overlapped with those present in mammals, i.e. Lys-30, Lys-79, Lys-316 (corresponding to Lys-318 in mammals) and Lys-390 [Citation22]. More recent studies using mass spectrometry (MS) have confirmed these methylation sites, and also revealed an additional site, Lys-3, in yeast eEF1A [Citation16,Citation19].
Figure 2. KMT-mediated lysine methylation. A lysine can accept up to three methyl groups through successive, KMT-mediated methylation. KMTs use S-adenosylmethionine (AdoMet), as methyl donor (not shown). Some KMT-mediated methylations can be reversed by lysine-specific demethylases (KDMs).
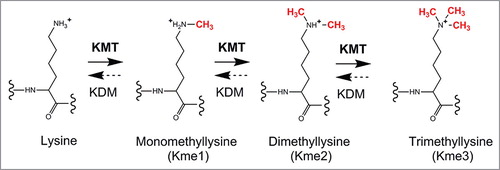
Figure 3. Methylated lysines and corresponding KMTs in yeast and human eEF1A. The three domains (I, II, III) of eEF1A are indicated in different shades of green colouring on a cartoon representation of the eEF1A structure. Methylated lysines in human and yeast eEF1A are indicated by red and blue labels, respectively, and also shown in stick representation, where methylation sites that are unique to human or yeast eEF1A are indicated in red and blue, respectively, whereas sites that are found in both proteins are shown in purple. The shown structure represents S. cerevisiae eEF1A (PDB 1F60), but since one of the methylated Lys residues in human eEF1A, Lys-165, is not conserved in yeast eEF1A, the corresponding residue (Ser-163) was, for the purpose of this illustration replaced by lysine (and indicated as human Lys-165). The KMTs responsible for lysine methylation in yeast and human (Efms and eEF1A-KMTs, respectively) are indicated. Asterisks indicate methylation sites that tend to display a mixture of different methylation states.
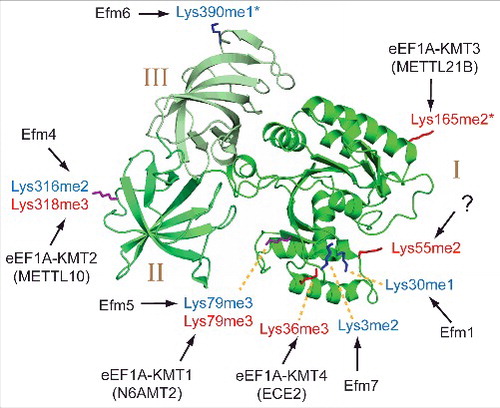
eEF1A-specific KMTs
The KMT enzymes belong to two structurally distinct methyltransferase (MTase) families: the so-called SET-domain MTases and the seven-β-strand (7BS) MTases. The SET-domain MTases exclusively comprise KMTs, and are responsible for the bulk of lysine methylations on histones [Citation23]. In contrast, the 7BS MTases collectively target a wide range of substrates (e.g. nucleic acids, metabolites, and proteins), and it has recently become evident that this group comprises many KMTs that target non-histone proteins, including eEF1A [Citation24].
Although lysine methylation on eEF1A was first reported more than three decades ago, the responsible KMTs have been discovered and characterized only during the last decade, pioneered by studies in S. cerevisiae. An overview of the currently known eEF1A-specific KMTs in yeast and humans is found in . The first two eEF1A-specific KMTs were discovered by Clarke and co-workers through assessing the lysine methylation status of eEF1A in various yeast knock-out (KO) strains with inactivated MTase genes [Citation25]. A subsequent study firmly established that these enzymes, now denoted Efm1 and Efm4, monomethylated Lys-30 and dimethylated Lys-316, respectively [Citation26]. Somewhat surprisingly, a putative (based on sequence homology) RNA MTase was found to be responsible for trimethylation of Lys-79, and this KMT was denoted Efm527. Also, the KMT responsible for monomethylation at Lys-390, denoted Efm6, was recently identified [Citation5]. Finally and interestingly, a KMT mediating dimethylation at Lys-3, denoted Efm7, was shown to have a dual activity, also methylating the N-terminus of eEF1A [Citation19].
Methylation at Lys-79 and Lys-316 in yeast eEF1A is conserved to humans, and the human genome was found to encode putative orthologues of the Efm4 and Efm5 proteins. Accordingly, the putative Efm4 orthologue, METTL10, was found to mediate trimethylation at Lys-318 in human eEF1A, and was therefore denoted eEF1A-KMT2 (gene name: EEF1AKMT2) whereas the N6AMT2 protein, a putative orthologue of Efm5, was found to catalyse methylation of Lys-79, and denoted eEF1A-KMT1 (gene name: EEF1AKMT1) [Citation19,Citation28].
The majority of KMTs that target eEF1A in yeast and humans have now apparently been identified, but the functional significance and dynamics of these modifications have remained elusive. However, some very recent studies on two novel eEF1A-specific KMTs in humans, eEF1A-KMT3 (METTL21B) and eEF1A-KMT4 (ECE2) have shed some light on these aspects of eEF1A lysine methylation, and some of these findings are presented in the subsequent sections.
Recent progress on human eEF1A-specific KMTs
eEF1A-KMT3. We and others previously discovered a novel KMT family (denoted Methyltransferase Family 16), consisting of 10 human members [Citation29,Citation30]. To investigate the activity of one of these MTases, METTL21B, we tested its ability to methylate proteins in a cell extract in the presence of [3H]-labelled AdoMet. A single [3H]-labelled substrate of ∼ 50 kDa was observed, and enrichment of this substrate by ion exchange chromatography enabled its identification, by MS, as eEF1A [Citation31]. In vitro studies of METTL21B activity revealed that the enzyme targets Lys-165 in both eEF1A paralogues (eEF1A1 and eEF1A2), and requires the full eEF1 complex, including bound GTP and aminoacyl-tRNA, for efficient methylation [Citation31]. The latter is different from most other eEF1A-specific KMTs, which are capable of efficiently methylating non-complexed, recombinant eEF1A.
Since METTL21B was the third human KMT found to target eEF1A, it was redubbed eEF1A-KMT3 (gene name: EEF1AKMT3) [Citation31]. Importantly, CRISPR/Cas9 mediated KO of the eEF1A-KMT3 function in mammalian cells abolished Lys-165 methylation, and also caused interesting effects on protein synthesis (see next section) [Citation31]. Similar findings were reported by Hamey et al. in an independent study [Citation32]. Interestingly, Lys-165 displayed a mixture of methylation states (Kme1, Kme2 (frequently the most abundant), and Kme3) in mammalian cells, and subjecting cells to certain types of stress, such as growth factor withdrawal or treatment with the ER stress inducers tunicamycin or brefeldin A, led to increased levels of eEF1A methylation at Lys-165 [Citation31]. Importantly, the observed changes in Lys-165 methylation were mirrored by corresponding changes in the expression of EEF1AKMT3 mRNA, suggesting an active mechanism, rather than an indirect effect resulting from e.g. alterations in cellular AdoMet levels. Thus, eEF1A-KMT3-dependent methylation of Lys-165 in eEF1A is dynamic and inducible, suggesting that it has a regulatory function and is part of a stress-induced cellular response. Interestingly, subcellular localisation studies indicated that eEF1A-KMT3, in addition to its expected cytosolic localisation, was also present at the centrosomes, suggesting that eEF1A-KMT3 and the Lys165-methylated eEF1A may play a role in centrosome function and thus exert a non-canonical function of eEF1A [Citation31].
eEF1A-KMT4. The gene encoding human eEF1A-KMT4 was previously incorrectly annotated as being part of the ECE2 gene (encoding Endothelin Converting Enzyme 2). Based on transcriptomics data, we concluded that the cluster of exons corresponding to eEF1A-KMT4 should be considered a separate gene [Citation33], and we set out to characterize the corresponding recombinant enzyme. Using an experimental strategy similar to that described above for eEF1A-KMT3, we found that the enzyme targets one of the orphan lysine methylation sites – Lys-36 – in eEF1A, and this novel MTase was consequently renamed eEF1A-KMT4 (gene name: EEF1AKMT4). Notably, eEF1A-KMT4 was equally capable of methylating the two eEF1A paralogues, eEF1A1 and eEF1A2, and its in vitro activity was GTP-dependent.
To establish that eEF1A-KMT4 catalyses the corresponding methylation reaction in cells, the EEF1AKMT4 gene was knocked out in HAP-1 cells using the CRISPR/Cas9 technique, and the methylation status of Lys-36 was analysed by MS. This residue was found to be predominately trimethylated in wild type HAP-1 cells, but exclusively unmodified in the KO cells. Taken together, it was, using a combination of in vitro and in vivo methods, firmly established that eEF1A-KMT4 catalyses trimethylation of Lys-36 in eEF1A. Moreover, KO of eEF1A-KMT4 had striking, codon-specific effects on mRNA translation (further discussed in next section).
eEF1A-KMT4 belongs to a subfamily of four related 7BS MTases, and one of these is eEF1A-KMT2 (METTL10) which was previously shown to target Lys-318 in eEF1A [Citation28,33]. A third member, METTL12, was recently identified as the long-sought KMT responsible for lysine methylation of citrate synthase in mitochondria [Citation34,Citation35]. The last of these enzymes, METTL13, remains uncharacterized, but it is reasonable to speculate that it is a KMT.
Functional significance of eEF1A lysine methylation
Lysine methylation of histone proteins is generally highly dynamic with a strong impact on chromatin structure and gene activity, and the underlying molecular mechanisms have now been largely established. The dynamic nature of histone lysine methylation results from the action of several specific lysine demethylase enzymes (KDMs) capable of reversing these methylations [Citation36]. Also, a number of so-called "reader" domains recognize specific methylation states at the various lysine residues in histones, and these readers are usually fused to enzymatic domains that modify or remodel chromatin, thereby converting lysine methylation to functional output [Citation37]. In contrast, no unifying model or mechanism has been established for eEF1A when it comes to the functional significance of lysine methylations. However, the abundance of lysine methylations on eEF1A and the existence of several eEF1A-specific KMTs, some of which appear to be conserved throughout the eukaryotic kingdom, clearly indicates that eEF1A lysine methylation is functionally important.
Except for the Efm1 enzyme, which is a SET-domain protein, all the identified eEF1A-specific KMTs in yeast and human belong to the 7BS family of MTases. Many recently identified mammalian 7BS KMTs appear to provide complete trimethylation of their respective substrates, and this also seems to be the case for some of the eEF1A-specific KMTs. For example, the human eEF1A-KMT4 enzyme can install up to three methyl moieties on Lys-36 in vitro, and the trimethylated species was found to be predominant in various mammalian cells and tissues [Citation33]. In such cases, one may speculate that methylation represents an editing event that provides a general improvement of protein function, rather than a dynamic, regulatory signal. However, it should also be kept in mind that lysine methylation has generally been studied in model systems under conditions (rich cell culture media, well-fed animals) very different from those experienced by the corresponding organisms in their natural habitats under evolutionary selection pressure. Thus, it is not unlikely that lysine methylations that appear static when studied in these model systems will be more dynamic in a natural setting, reflecting their partaking in adaptive or regulatory processes.
Indeed, some recent findings indicate that eEF1A lysine methylation can be highly dynamic. Lys-390 in yeast eEF1A, which is methylated by Efm6, displayed very low methylation levels in vivo, corresponding to only ∼0.2 methyl groups per eEF1A molecule, but, notably, overexpression of Efm6 dramatically increased Lys-390 methylation, to ∼2 methyl groups per eEF1A [Citation5]. Moreover, Efm6 was recently reported to be strongly upregulated by hypoxia, suggesting that Efm6-mediated Lys-390 methylation may represent a hypoxia-inducible modulator of protein synthesis [Citation38]. Human eEF1A-KMT3 (described previously) represents a close human homologue of Efm6, and, interestingly, the EEF1AKMT3 gene was found to be induced by various stressors and perturbations, concomitant with increased methylation at the eEF1A-KMT3 target site, Lys-165 [Citation31]. Also, Lys-165 methylation levels varied considerably between various mammalian cells and tissues. Moreover, for the dimorphic fungus M. racemosus, where eEF1A lysine methylation was first reported, eEF1A methylation showed a high methylation level in actively growing fungi, but was almost completely unmethylated in spores [Citation21]. In summary, these findings demonstrate that eEF1A lysine methylation, at least in certain cases, is dynamic and inducible, which is suggestive of a regulatory role.
Although eEF1A lysine methylation was first reported several decades ago, evidence linking such methylation to the process of mRNA translation has been absent. However, recent studies of human KO cells lacking specific eEF1A-KMTs have indeed provided indications that eEF1A methylation influences the translational output from the ribosome. We found, through ribosomal profiling (RP) experiments, that eEF1A-KMT3 KO cells which lack methylation at Lys-165, showed altered translation of specific genes, compared with their wild-type counterpart [Citation31]. Similarly, Hamey et al. assessed eEF1A-KMT3 KO cells by proteomics experiments, and found that several proteins showed altered abundance [Citation32]. Reassuringly, the two studies gave similar results; genes related to the ribosome, RNA processing, and translation were up-regulated, whereas genes/proteins associated with the endoplasmic reticulum were down-regulated. Moreover, KO of eEF1A-KMT4, which is responsible for methylation at Lys-36, gave specific effects at the level of individual codons, as observed in RP experiments. It was found that codons associated with certain amino acids, such as Asn, showed a reduced occupancy at the A-site, reflecting a faster translation rate. In contrast, other codons, such as those encoding His, showed increased occupancy. Taken together, the above findings indicate that eEF1A lysine methylation influences the ability of eEF1A to interact with the various aminoacyl-tRNAs and/or mediate their interaction with the translating ribosome, thus modulating protein synthesis.
More than two decades ago, Cavallius et al. generated mutant yeast strains carrying a single copy of eEF1A gene, where methylated lysines had been replaced by arginine, and thus assessed the importance of these lysines for yeast viability [Citation39]. Notably, even the yeast strain representing the combined quadruple mutant, where all four methylated lysines had been replaced (K30R, K79R, K316R, K390R), was viable and without any obvious phenotype, and eEF1A protein isolated from this strain showed properties indistinguishable from the corresponding wild-type protein. Clearly, it may be discussed whether the replacement of lysine by arginine is a suitable approach for investigating the significance of lysine methylation; the point has been made that a function for lysine methylation may be to protect proteins against ubiquitin-mediated degradation (since ubiquitin cannot be attached to a methylated lysine), and that such protection would also be accomplished by replacing lysine with arginine [Citation39]. Nonetheless, it may be concluded that the tolerance for substitutions at the methylation sites appears to be rather high when it comes to maintaining the basic, essential function of eEF1A, suggesting that the effects of methylation per se are likely modest, at least during near-optimal growth in the laboratory. Anyway, now that the KMTs responsible for all lysine methylations on eEF1A have been identified in yeast, it may be of great interest to study yeast strains defective in one or several of these KMTs for their ability to support efficient and accurate protein synthesis, e.g. by RP or quantitative MS.
The effects of eEF1A lysine methylation on mRNA translation are apparently rather modest, and one should also consider the possibility that lysine methylation primarily affects one (or several) of the non-canonical functions of eEFA1. This remains largely unexplored, but a few studies provide some indications in this respect. It was found that KO or knock-down of the Efm4 function caused a strong reduction in the formation of plant viral RNAs and proteins, both in Nicotiana benthamiana plants and in S. cerevisiae (surrogate host) indicating that Lys-316 methylation of eEF1A is important for viral replication [Citation40]. Also, the observation that mammalian eEF1A-KMT3 is partially localized to the centrosome may suggest a non-canonical function for this enzyme and the corresponding methylation at Lys-165 in eEF1A [Citation31].
Conclusions and further perspectives
Besides histones, eEF1A is one of the few proteins where several highly specific KMTs install methylations at distinct lysine residues. During recent years several of the KMTs responsible for lysine methylation of eEF1A have been identified and characterised, and there are indications that eEF1A methylation can be inducible, dynamic and of regulatory importance. Lysine methylation and other dynamic PTMs on histones have been proposed to constitute a "histone code" that regulates transcription. By analogy, and based on the recent findings described herein, we suggested the existence of an "eEF1A code", with lysine methylation as a key component, contributing to shaping the proteome by regulating mRNA translation [Citation33].
However, despite considerable recent progress, the functional significance of eEF1A lysine methylation remains largely elusive. Nonetheless, with most of the eEF1A-specific KMTs now identified in humans and yeast, taken together with the recent technological advances, regarding e.g. RP, CRISPR/Cas9-mediated gene KO and MS-based proteomics, the stage is now set for investigating how both canonical and non-canonical functions of eEF1A are affected by lysine methylation.
Disclosure of potential conflicts of interest
No potential conflicts of interest were disclosed.
Acknowledgments
We are grateful to Rita Pinto and Erna Davydova for critical reading of the manuscript.
Additional information
Funding
References
- Negrutskii BS, El'skaya AV. Eukaryotic translation elongation factor 1 alpha: structure, expression, functions, and possible role in aminoacyl-tRNA channeling. Prog Nucleic Acid Res Mol Biol. 1998;60:47–78. doi:10.1016/S0079-6603(08)60889-2
- Sasikumar AN, Perez WB, Kinzy TG. The many roles of the eukaryotic elongation factor 1 complex. Wiley Interdiscip Rev RNA. 2012;3:543–555. doi:10.1002/wrna.1118
- Andersen GR, Pedersen L, Valente L, et al. Structural basis for nucleotide exchange and competition with tRNA in the yeast elongation factor complex eEF1A:eEF1Balpha. Mol Cell. 2000;6:1261–1266. doi:10.1016/S1097-2765(00)00122-2
- Mateyak MK, Kinzy TG. eEF1A: thinking outside the ribosome. J Biol Chem. 2010;285:21209–21213. doi:10.1074/jbc.R110.113795
- Jakobsson ME, Davydova E, Malecki J, et al. Saccharomyces cerevisiae Eukaryotic Elongation Factor 1A (eEF1A) Is Methylated at Lys-390 by a METTL21-Like Methyltransferase. PLoS ONE. 2015;10:e0131426. doi:10.1371/journal.pone.0131426
- Abbas W, Kumar A, Herbein G. The eEF1A Proteins: At the Crossroads of Oncogenesis, Apoptosis, and Viral Infections. Front Oncol. 2015;5:75. doi:10.3389/fonc.2015.00075
- Kim S, Coulombe PA. Emerging role for the cytoskeleton as an organizer and regulator of translation. Nat Rev Mol Cell Biol. 2010;11:75–81. doi:10.1038/nrm2818
- Yang F, Demma M, Warren V, et al. Identification of an actin-binding protein from Dictyostelium as elongation factor 1a. Nature. 1990;347:494–496. doi:10.1038/347494a0
- Demma M, Warren V, Hock R, et al. Isolation of an abundant 50,000-dalton actin filament bundling protein from Dictyostelium amoebae. J Biol Chem. 1990;265:2286–2291.
- Munshi R, Kandl KA, Carr-Schmid A, et al. Overexpression of translation elongation factor 1A affects the organization and function of the actin cytoskeleton in yeast. Genetics. 2001;157:1425–1436.
- Gross SR, Kinzy TG. Translation elongation factor 1A is essential for regulation of the actin cytoskeleton and cell morphology. Nat Struct Mol Biol. 2005;12:772–778. doi:10.1038/nsmb979
- Li D, Wei T, Abbott CM, et al. The unexpected roles of eukaryotic translation elongation factors in RNA virus replication and pathogenesis. Microbiol Mol Biol Rev. 2013;77:253–266. doi:10.1128/MMBR.00059-12
- Whiteheart SW, Shenbagamurthi P, Chen L, et al. Murine elongation factor 1 alpha (EF-1 alpha) is posttranslationally modified by novel amide-linked ethanolamine-phosphoglycerol moieties. Addition of ethanolamine-phosphoglycerol to specific glutamic acid residues on EF-1 alpha. J Biol Chem. 1989;264:14334–14341.
- Dever TE, Costello CE, Owens CL, et al. Location of seven post-translational modifications in rabbit elongation factor 1 alpha including dimethyllysine, trimethyllysine, and glycerylphosphorylethanolamine. J Biol Chem. 1989;264:20518–20525.
- Jank T, Belyi Y, Wirth C, et al. Protein glutaminylation is a yeast-specific posttranslational modification of elongation factor 1A. J Biol Chem. 2017;292:16014–16023. doi:10.1074/jbc.M117.801035
- Hornbeck PV, Zhang B, Murray B, et al. PhosphoSitePlus, 2014: mutations, PTMs and recalibrations. Nucleic Acids Res. 2015;43:D512–D520. doi:10.1093/nar/gku1267
- Lin KW, Yakymovych I, Jia M, et al. Phosphorylation of eEF1A1 at Ser300 by TbetaR-I results in inhibition of mRNA translation. Curr Biol. 2010;20:1615–1625. doi:10.1016/j.cub.2010.08.017
- Sanges C, Scheuermann C, Zahedi RP, et al. Raf kinases mediate the phosphorylation of eukaryotic translation elongation factor 1A and regulate its stability in eukaryotic cells. Cell Death Dis. 2012;3:e276. doi:10.1038/cddis.2012.16
- Hamey JJ, Winter DL, Yagoub D, et al. Novel N-terminal and lysine methyltransferases that target translation elongation factor 1A in yeast and human. Mol Cell Proteomics. 2015;15:164–176. doi:10.1074/mcp.M115.052449
- Zobel-Thropp P, Yang MC, Machado L, et al. A novel post-translational modification of yeast elongation factor 1A. Methylesterification at the C terminus. J Biol Chem. 2000;275:37150–37158. doi:10.1074/jbc.M001005200
- Hiatt WR, Garcia R, Merrick WC, et al. Methylation of elongation factor 1 alpha from the fungus Mucor. Proc Natl Acad Sci U S A. 1982;79:3433–3437. doi:10.1073/pnas.79.11.3433
- Cavallius J, Zoll W, Chakraburtty K, et al. Characterization of yeast EF-1 alpha: non-conservation of post-translational modifications. Biochim Biophys Acta. 1993;1163:75–80. doi:10.1016/0167-4838(93)90281-U
- Herz HM, Garruss A, Shilatifard A. SET for life: biochemical activities and biological functions of SET domain-containing proteins. Trends Biochem Sci. 2013;38:621–639. doi:10.1016/j.tibs.2013.09.004
- Falnes PO, Jakobsson ME, Davydova E, et al. Protein lysine methylation by seven-β-strand methyltransferases. Biochem J. 2016;473:1995–2009. doi:10.1042/BCJ20160117
- Lipson RS, Webb KJ, Clarke SG. Two novel methyltransferases acting upon eukaryotic elongation factor 1A in Saccharomyces cerevisiae. Arch Biochem Biophys. 2010;500:137–143. doi:10.1016/j.abb.2010.05.023
- Couttas TA, Raftery MJ, Padula MP, et al. Methylation of translation-associated proteins in Saccharomyces cerevisiae: Identification of methylated lysines and their methyltransferases. Proteomics. 2012;12:960–972. doi:10.1002/pmic.201100570
- Dzialo MC, Travaglini KJ, Shen S, et al. A new type of protein lysine methyltransferase trimethylates Lys-79 of elongation factor 1A. Biochem Biophys Res Commun. 2014;455:382–389. doi:10.1016/j.bbrc.2014.11.022
- Shimazu T, Barjau J, Sohtome Y, et al. Selenium-based S-adenosylmethionine analog reveals the mammalian seven-beta-strand methyltransferase METTL10 to be an EF1A1 lysine methyltransferase. PLoS ONE. 2014;9:e105394. doi:10.1371/journal.pone.0105394
- Cloutier P, Lavallee-Adam M, Faubert D, et al. A newly uncovered group of distantly related lysine methyltransferases preferentially interact with molecular chaperones to regulate their activity. PLoS Genet. 2013;9:e1003210. doi:10.1371/journal.pgen.1003210
- Kernstock S, Davydova E, Jakobsson M, et al. Lysine methylation of VCP by a member of a novel human protein methyltransferase family. Nat Commun. 2012;3:1038. doi:10.1038/ncomms2041
- Malecki J, Aileni VK, Ho AY, et al. The novel lysine specific methyltransferase METTL21B affects mRNA translation through inducible and dynamic methylation of Lys-165 in human eukaryotic elongation factor 1 alpha (eEF1A). Nucleic Acids Res. 2017;45:4370–4389.
- Hamey JJ, Wienert B, Quinlan KGR, et al. METTL21B Is a Novel Human Lysine Methyltransferase of Translation Elongation Factor 1A: Discovery by CRISPR/Cas9 Knockout. Mol Cell Proteomics. 2017;16:2229–2242. doi:10.1074/mcp.M116.066308
- Jakobsson ME, Malecki J, Nilges BS, et al. Methylation of human eukaryotic elongation factor alpha (eEF1A) by a member of a novel protein lysine methyltransferase family modulates mRNA translation. Nucleic Acids Res. 2017;45:8239–8254. doi:10.1093/nar/gkx432
- Malecki J, Jakobsson ME, Ho AYY, et al. Uncovering human METTL12 as a mitochondrial methyltransferase that modulates citrate synthase activity through metabolite-sensitive lysine methylation. J Biol Chem. 2017;292:17950–17962. doi:10.1074/jbc.M117.808451
- Rhein VF, Carroll J, Ding S, et al. Human METTL12 is a mitochondrial methyltransferase that modifies citrate synthase. FEBS Lett. 2017;591:1641–1652. doi:10.1002/1873-3468.12649
- Dimitrova E, Turberfield AH, Klose RJ. Histone demethylases in chromatin biology and beyond. EMBO Rep. 2015;16:1620–1639. doi:10.15252/embr.201541113
- Taverna SD, Li H, Ruthenburg AJ, et al. How chromatin-binding modules interpret histone modifications: lessons from professional pocket pickers. Nat Struct Mol Biol. 2007;14:1025–1040. doi:10.1038/nsmb1338
- Bendjilali N, MacLeon S, Kalra G, et al. Time-Course Analysis of Gene Expression During the Saccharomyces cerevisiae Hypoxic Response. G3 (Bethesda ). 2017;7:221–231. doi:10.1534/g3.116.034991
- Cavallius J, Popkie AP, Merrick WC. Site-directed mutants of post-translationally modified sites of yeast eEF1A using a shuttle vector containing a chromogenic switch. Biochim Biophys Acta. 1997;1350:345–358. doi:10.1016/S0167-4781(96)00181-9
- Li Z, Gonzalez PA, Sasvari Z, et al. Methylation of translation elongation factor 1A by the METTL10-like See1 methyltransferase facilitates tombusvirus replication in yeast and plants. Virology. 2014;448:43–54. doi:10.1016/j.virol.2013.09.012