ABSTRACT
Clostridioides difficile is the main cause of nosocomial antibiotic-associated diarrhoea. There is a need for new antimicrobials to tackle this pathogen. Guanine riboswitches have been proposed as promising new antimicrobial targets, but experimental evidence of their importance in C. difficile is missing. The genome of C. difficile encodes four distinct guanine riboswitches, each controlling a single gene involved in purine metabolism and transport. One of them controls the expression of guaA, encoding a guanosine monophosphate (GMP) synthase. Here, using in-line probing and GusA reporter assays, we show that these riboswitches are functional in C. difficile and cause premature transcription termination upon binding of guanine. All riboswitches exhibit a high affinity for guanine characterized by Kd values in the low nanomolar range. Xanthine and guanosine also bind the guanine riboswitches, although with less affinity. Inactivating the GMP synthase (guaA) in C. difficile strain 630 led to cell death in minimal growth conditions, but not in rich medium. Importantly, the capacity of a guaA mutant to colonize the mouse gut was significantly reduced. Together, these results demonstrate the importance of de novo GMP biosynthesis in C. difficile during infection, suggesting that targeting guanine riboswitches with analogues could be a viable therapeutic strategy.
Introduction
Clostridioides difficile (formerly known as Clostridium difficile) is a Gram-positive pathogen causing intestinal infections in humans and animals. It is currently the most important cause of healthcare-associated post-antibiotic infections, but it also causes an increasing number of community-acquired infections [Citation1]. The current therapy consists in oral administration of antibiotics, such as vancomycin or fidaxomicin [Citation2]. Despite a relatively good efficacy of these antibiotics against C. difficile, recurrent infections are highly problematic. Non-conventional approaches such as faecal microbiota transplantation (FMT) have proved to be effective to treat recurrent infections, but FMT has its own limitations and cannot be applied to a large population or for milder cases [Citation3]. Thus, it is important to develop new drugs to fight this important pathogen.
Riboswitches are interesting new targets for the development of novel antibiotics to fight emerging multidrug resistant bacteria [Citation4,Citation5]. Riboswitches are cis-acting RNA elements located in the 5ʹ untranslated region (5ʹ-UTR) of several bacterial mRNA [Citation6]. They often control the expression of genes involved in the biosynthesis of important metabolites such as vitamins and cofactors, and new classes have recently been identified [Citation7,Citation8]. Riboswitches are typically composed of two functional domains: the aptamer, which selectively binds with high affinity to a target metabolite, and the expression platform that controls the expression of the downstream gene(s) [Citation9,Citation10]. In case of transcriptional switches, the binding of a metabolite results in the formation of a transcriptional terminator that consists of a stable stem-loop followed by a stretch of uracil residues () [Citation11].
Figure 1. Genetic regulation of the guanine riboswitch. A) Schematic representation of the guanine riboswitch in the ON (left) and OFF (right) conformation. The binding of guanine in the OFF conformation induces the formation of a transcription terminator that is characterized by the formation of a stem-loop structure followed by a stretch of uracils. B) Simplified metabolic view of the metabolism of purines and GMP biosynthesis in C. difficile. Solid arrows indicate that C. difficile encodes the enzymes necessary to perform the indicated substrate conversions (only the relevant genes are indicated). Dotted grey lines indicate reactions for which a corresponding gene product was not found in C. difficile (based on the Kegg database). The four genes that are controlled by a guanine riboswitch (guaA, xpt, 21070 and 27040) are shown in blue circles or rectangles. See the text for details about these genes
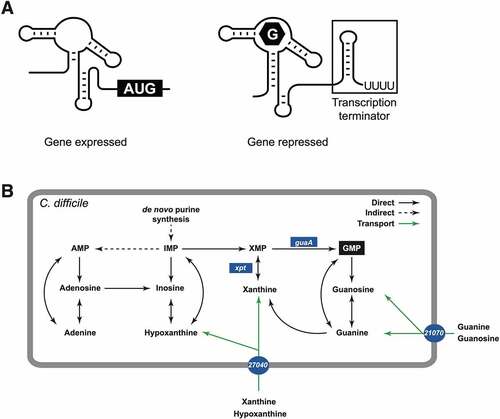
Guanosine monophosphate (GMP) is an important cellular metabolite for signalling (e.g. cyclic di-GMP), and for bacterial virulence and survival [Citation12]. GMP can be generated directly from guanine and guanosine, following simple enzymatic reactions (). In the absence of guanine or guanosine in the growth environment, C. difficile can use adenine and other purine analogs such as xanthine and hypoxanthine for conversion into inosine monophosphate (IMP) that can be further converted into xanthine monophosphate (XMP), and ultimately GMP through the action of the GMP synthase (guaA). If these purine precursors are unavailable, bacteria can use nucleotide salvation pathways, or proceed to de novo biosynthesis of IMP through a multistep pathway involving genes from the pur operon () [Citation13–16]. Inactivation of guaA generally leads to guanine auxotrophy and therefore, guaA is crucial for de novo biosynthesis of GMP [Citation17–19]. In Staphylococcus aureus, guaA is part of an operon containing xpt (xanthine phosphoribosyl transferase), pbuX (purine permease) and guaB (IMP dehydrogenase). Riboswitch-independent alternative promoters were also identified upstream of guaA and guaB [Citation19]. The 4-gene operon is under the control of a single guanine riboswitch that inhibits transcription elongation upon guanine binding [Citation20]. The purine metabolic pathways in S. aureus suggest a less versatile conversion system in which pools of AMP cannot be directly converted to IMP through inosine (Supplementary Figure S1). However, GMP may be directly converted to IMP, thus providing a rationale for S. aureus to supply IMP through different pathways. In C. difficile, four different guanine riboswitches are predicted (Supplementary Figure S2A), and each of them is expected to control the expression of a single gene in GMP synthesis and purine transport. This suggests an independent regulation of the four genes depending on the intracellular levels of guanine and/or related metabolites [Citation21]. In C. difficile strain 630, the gene CD630_23300 encodes a predicted xanthine phosphoribosyl transferase (Xpt) and CD630_01980 encodes a predicted GMP synthase (GuaA). The products of these genes would catalyse the conversion of xanthine into XMP, and XMP into GMP, respectively (). The genes CD630_21070 and CD630_27040 (hereafter simply 21070 and 27040) encode proteins with conserved domains found in NCS2 (nucleobase-cation symporter-2) permease family similar to Escherichia coli adenine permeases (AdeP and AdeQ) and guanine/hypoxanthine permeases (GhxP and GhxQ), as well as xanthine/uracil/vitamin C permeases of the AzgA family (IPR026033, COG2252 and pfam00860). While the exact nature of the substrate(s) transported by these permeases is unknown, bioinformatics analyses suggest that possible substrates could include xanthine, uracil, vitamin C, and possibly others.
Despite the presence of several genes predicted to participate in purine metabolism and de novo GMP biosynthesis in C. difficile, little is known about these pathways and their importance for cell survival. However, available data suggest that GuaA is important for C. difficile survival since guanine analogs targeting the guanine riboswitch controlling guaA expression leads to growth inhibition [Citation21]. Still, direct experimental evidence for the importance of GuaA and GMP metabolism are missing for C. difficile, in particular in the context of gut infection. Here, we show the functionality of the four C. difficile guanine riboswitches controlling guaA and three other genes (xpt, 21070 and 27040) associated with purine transport and metabolism. Importantly, we demonstrate that the inactivation of guaA in C. difficile strain 630Δerm leads to guanine/GMP auxotrophy and affects bacterial growth and survival in vitro. We also show that a guaA mutant is less virulent and competitive in a mouse model of C. difficile infection. Our study further supports the central importance of guaA expression and the potential of guanine riboswitches as therapeutic targets for the treatment of C. difficile infections.
Results
Genomic organization of guanine riboswitch-regulated genes.
Comparative genomic analyses revealed that in contrast to S. aureus that employs a single guanine riboswitch to control the xpt-pbuX-guaB-guaA operon [Citation21], C. difficile uses four different riboswitches to modulate the expression of each gene (). Such a difference in the organization of riboswitch-controlled genes between S. aureus and C. difficile suggests that both bacteria regulate gene expression using different molecular mechanisms. As a way to determine the diversity of guanine riboswitch genomic arrangements, all occurrences of guanine aptamers were searched for in the Bacteria domain using the Rfam database. The riboswitch downstream genes were identified (see Materials and Methods) using defined keywords corresponding to xpt, pbuX, guaA or guaB. The purE riboswitch controlling a twelve-gene operon [Citation14] was included in the analysis as an internal control for our bioinformatics analysis. We did not include 21070 and 27040 in our search since their exact function is still unclear. Across the database, a proportion of 20% of riboswitches had no annotated gene within the 300 nt downstream of the riboswitch locus. These sequences were therefore excluded, leaving 2,023 candidates exhibiting both a riboswitch and at least one downstream regulated gene. Similarly to C. difficile, the majority of riboswitches appeared to perform regulation of single genes (~64%), while the rest of regulated operons contained a variable number of genes (). Among all the riboswitches, 42% were predicted to regulate at least one of the investigated genes. The observed frequency of these genes downstream of the riboswitches was unevenly distributed in such a way that xpt > pbuX > guaA > guaB (, see inset). These observations suggest that genes involved in xanthine metabolism (xpt and pbuX) may be more dependent on riboswitch regulation than those involved in GMP regulation (guaA and guaB) (). Furthermore, according to an intersectional genomic analysis, of the riboswitches regulating operons containing at least one of the four interesting genes, 52% were found to control either xpt or pbuX individually, where 15% were controlling these two genes in a tandem arrangement (, inset). Other regulated tandem arrangements were observed to a lesser degree, such as guaA and guaB. Whereas 25% of riboswitches were found before either guaA or guaB, another 5% were found before both genes, therefore sharing the same riboswitch. Another commonly encountered configuration consists in the large twelve-genes purE operon (). Also, only ~1% of the riboswitch-associated operons were organized as four-gene operons containing each gene of interest, thus poorly representing the S. aureus genomic arrangement. Surprisingly, operons containing three genes did not featured one of the tandem (xpt-pbuX or guaA-guaB) with a single gene from the other tandem, thus reinforcing the idea that xanthine and GMP metabolism are mostly regulated using different guanine riboswitches. The three-gene operons encountered were rather constituted of a combination of unannotated genes. Further analyses showed that these two arrangements, xpt-pbuX and guaA-guaB, were respectively overrepresented in bacterial classes Bacilli and Clostridia (data not shown). The examination of riboswitch distribution revealed that Bacilli and Clostridia are the most represented classes in the dataset, respectively making 61% and 26% (). The other purine riboswitches are vastly distributed among numerous bacterial phyla, mostly in Firmicutes and the related Tenericutes (). The purine riboswitches are also observed in a significant proportion of Gram-negative bacteria, such as the Gammaproteobacteria, Fusobacteria and Oligoflexia (). Overall, our findings indicate that guanine riboswitch-regulated genes are occurring mainly through small operons or single gene, which would allow to specifically regulate the expression of XMP or GMP, which contrasts with a more coordinated regulation using a single riboswitch as found in S. aureus.
Figure 2. Guanine riboswitch-controlled genes. A) Schematic representation of the genes under the control of a guanine riboswitch in S. aureus and C. difficile. (B) Proportion of riboswitch-controlled operons. The number of genes for each operon are indicated below the graph. The inset shows the proportion of the combination of each pair of indicated genes among the 854 operons containing at least one of them. (C) Distribution of purine riboswitches among 2562 bacterial classes. Riboswitches have been identified in Bacilli (61%) and Clostridia (26%), and the rest is shown as Others (13%). (D) Distribution of purine riboswitches from the Others category represented at the phylum taxonomic level (Fig. 2C). The largest represented phyla are composed of Firmicutes, Gammaproteo bacteria, Tenericutes and Fusobacteria. A variety of smaller bacterial phyla is also shown
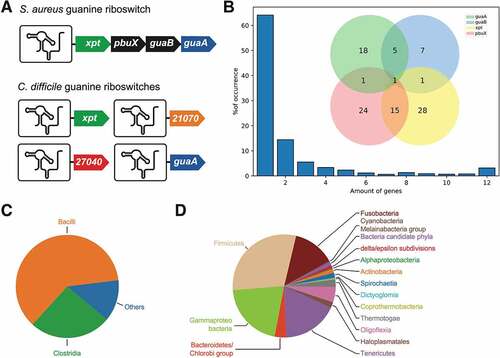
A multiple sequence alignment obtained with the consensus sequence of the purine aptamers available in the Rfam database shows that each sequence is different but that the predicted secondary structures and the conserved nucleotides in the core region are consistent with the expected aptamer structure (Supplementary Figures S2A and S2B). Furthermore, sequence analysis of C. difficile guanine riboswitches suggests that they all function as transcriptional attenuators upon guanine binding since an anti-terminator stem is predicted to disrupt a terminator structure in each case (Supplementary Figure S3). To determine whether C. difficile guanine riboswitches were capable of binding guanine, we first performed in-line probing assays with the corresponding aptamer of each riboswitch [Citation22]. These assays exploit the inherent chemical instability of RNA molecules under physiological conditions, which is increased in unstructured regions due to their ability to adopt an ‘in-line’ conformation [Citation22]. When performing in-line probing assays with the C. difficile guaA aptamer, we observed that the aptamer core region (positions 38–44) was cleaved at low concentrations of guanine and became protected as the concentrations increased (Supplementary Figure S4A). This indicated that the guaA aptamer is able to bind guanine and fitting analysis revealed a dissociation constants (Kd) for the ligand of 21 ± 2 nM (Supplementary Figure S4A), which is ~4-fold higher than the Bacillus subtilis xpt riboswitch [Citation20]. We also performed in-line probing assays using the xpt, 21070 and 27040 aptamers (Supplementary Figure S4B). Constant dissociation values of 1.2 ± 0.2, 4.1 ± 1.1, and 6.4 ± 1.6 nM were measured, respectively, suggesting that all C. difficile riboswitches exhibit high affinity towards guanine. Together, our data show that the four riboswitches can bind guanine with high affinity in vitro.
Guanine and related metabolites trigger riboswitch-mediated differential gene expression in C. difficile
We next investigated the regulatory activity of C. difficile guanine riboswitches. To do so, we constructed gusA transcriptional fusions that were expressed using the constitutive promoter Pcwp2 [Citation23]. Each construct contained a purine riboswitch and the first 10 codons of its associated downstream gene that were fused to gusA, therefore acting as a genetic reporter of riboswitch activity (Supplementary Figure S5). Cells were incubated for 2 h in MDM supplemented with increasing concentrations of either guanine or the analogs xanthine or guanosine, and the ß-glucuronidase activity was determined on whole bacterial lysates. The results obtained for guaA show that the reporter activity was downregulated with increasing concentrations of guanine, with ~45% reduction in GusA activity using 50 mg/L guanine (). While a comparable inhibition was obtained with 50 mg/L xanthine, a weaker decrease (~20%) in GusA activity was observed when using 50 mg/L guanosine and high concentrations (3000 mg/L) had to be used to get a ~ 40% decrease (). When assessing the xpt and 21070 riboswitches, the GusA activity was decreased in the presence of guanine, xanthine and guanosine (). However, the 21070 riboswitch showed a stronger inhibition of GusA activity (~50%) in the presence of guanosine. Finally, the GusA activity assays revealed that while the expression of the 27040 riboswitch was not significantly decreased by guanine, it was strongly affected (~60% inhibition) by xanthine (), and both metabolites seem to have different regulatory effects on the riboswitch. These results suggest some level of specificity between the four guanine riboswitches in their natural context. It also suggests that depending on the intracellular levels of guanine and related metabolites, the activity of these riboswitches and the expression of their cognate genes are modulated differently. A control experiment, in which the gusA gene was placed under the control of the Pcwp2 promoter, in the absence of the intervening riboswitch, did not show any difference in gene regulation in the presence of guanine, suggesting that the reduction in GusA activity was directly due to an effect on the riboswitch and not on the promoter (Data not shown).
Figure 3. Functionality of the guaA riboswitches determined by reporter assays in C. difficile. GusA reporter assays were conducted with pSMI1, pSMI2, pSMI3 and pSMI4, carrying transcriptional fusions of the gusA gene with the guaA, xpt, 27040 and 21070 riboswitches, respectively. The activity was determined after 2 h of incubation in MDM broth supplemented with different concentrations of the indicated metabolites. The results shown represent the mean and standard deviations from 3 independent experiments
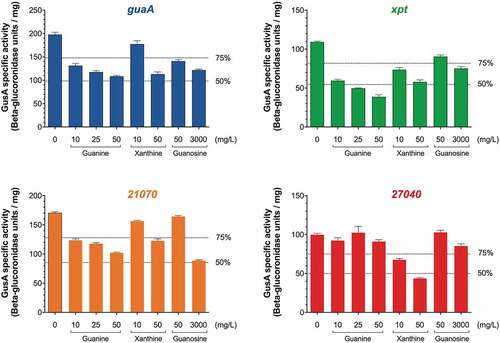
In summary, our results confirm that the four predicted guanine riboswitches are functional and that C. difficile regulates GMP synthesis by sensing the presence of guanine and related metabolites in its environment and controlling their transport and metabolism accordingly.
Inactivation of the GMP synthase GuaA in C. difficile leads to growth defects and auxotrophy
To determine the importance of the guanine riboswitch-controlled genes in C. difficile, we inactivated the four associated genes in the strain 630Δerm and assessed their importance by monitoring cell growth. The ClosTron gene inactivation system [Citation24] was used to disrupt the guaA, xpt, 21070, and 27040 genes. Mutants were readily isolated on brain heart infusion agar plates, suggesting that the product of these genes is non-essential in rich growth conditions. We hypothesized that C. difficile was able to generate GMP directly from guanine or guanosine, which are likely present in a rich medium such as BHIS. When cultured in BHIS broth (), the guaA mutant grew a little slower than the WT and the other mutants but reached a similar final bacterial density after 15 h. All mutants were able to grow on BHIS agar plates (), in agreement with a rich medium allowing efficient growth.
Figure 4. Growth of the wild type (WT) and mutant C. difficile strains in different conditions. The WT strain 630Δerm and the indicated mutants (guaA, xpt, 21070 and 27040) were grown in BHIS broth (A) or MDM broth (C and E). Bacterial counts are reported in CFU/mL in the corresponding graphs. The strains were also streaked and grown on BHIS agar (B), or MHBCA agar (D and F). Where indicated, the guaA mutant was complemented with the pSMI5 plasmid carrying a copy of the WT guaA gene under the control of the constitutive Pcwp2 promoter. The empty plasmid pRPF144E was used as a control. The broth cultures were repeated three times and values represent the mean and standard deviation
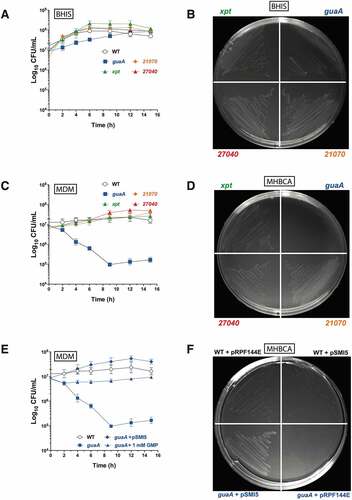
We next assessed growth of the mutants in poorer media such as cation-adjusted Mueller–Hinton broth (MHBCA) and C. difficile minimal-defined medium (MDM) [Citation25]. In the MDM broth and MHBCA agar plates, only the guaA mutant was affected ( ). The guaA mutant showed a 2-log reduction in colony forming units (CFU)/mL in MDM broth over the first 8 h (), indicating significant mortality. Complementation of the guaA mutant with a plasmid copy of the guaA gene restored the growth phenotype in MDM broth (), as well as on MHBCA agar plates (). Control experiments performed in rich media (BHIS agar) showed that both the mutant and complemented strains grew well (Supplementary Figure S6).
The above results strongly suggest the essentiality of guaA in poor growth conditions in which GMP de novo biosynthesis is required. As a confirmation of the importance of guaA for GMP synthesis, we were able to rescue guaA mutant cells in MDM by the addition of 1 mM GMP to the medium (). The slight growth recovery observed with the guaA mutant after 8 h in the absence of GMP was probably caused by the release of cellular metabolites upon cell lysis, thereby enabling cells to grow using readily available guanine, guanosine and/or GMP. Taken together, our data suggest that only the product of guaA is essential under laboratory conditions.
Inactivation of guaA is associated with poor survival and infectivity in a mouse model of infection
Our in vitro growth assays showed that under nutrient-limiting conditions, the activity of guaA becomes essential for bacterial survival. To determine whether guaA activity is important for C. difficile infection, we assessed the capacity of guaA mutant cells to colonize mice and cause disease. For these assays, we used a co-infection strategy whereby equal numbers (i.e. 105 CFU) of C. difficile spores of the wild-type and guaA mutant were co-administered simultaneously in a mouse model of infection (). As shown in , the wild-type strain 630Δerm readily colonized mice and reached high faecal titres (>108 total CFU/g faeces) as soon as 1-day post-infection. Under the same conditions, the colonization of the guaA mutant remained low with ~5×103 CFU/g faeces. After 2 days of infection, the mutant reached a maximum titre of ~106 CFU/g faeces, but was still 2-log below the wild-type strain. At 6 days post-infection, bacterial counts of the guaA mutant were below the detection limit of 103 CFU/g faeces. The total counts of the wild-type strain started to decrease after 7 days but were still over 106 CFU/g faeces at day 9. When counting spores only, a similar trend was observed, although in this case the counts of the guaA mutant were very low 1-day post-infection and fell below the limit of detection at day 2 (). Moreover, compared with the wild-type strain for which spores represented between 1% and 10%, spore counts for the guaA mutant were below the level of detection after 48 h, suggesting impaired sporulation in vivo. Spores are mainly responsible for the persistence of C. difficile in the gut and in the environment, leading to recurrent disease, and thus represent an important aspect of the life cycle of the bacterium. Taken together, these results show that inactivation of guaA leads to severe colonization defects, poor infectivity and competitiveness and sporulation defects in the mouse model.
Figure 5. Impact of guaA inactivation in the mouse model of C. difficile infection. (A) Schematic view of the infection protocol. The timeline is represented by a horizontal black arrow and the numbers below represent time in days. (B and C) C57BL/6 mice were co-infected with 105 spores each of wild type strain 630Δerm (empty dots) and guaA mutant (blue triangles). Total bacterial counts (B) and spore counts (C) are shown at different time points post-infection and are reported as CFU/g faeces. Each dot/triangle represents a single mouse, and because severe diarrhoea prevented faeces to be collected from some mice, some points are missing. The limit of detection was set at 103 CFU/g faeces and is indicated by a dotted line. The experiment has been repeated twice and representative results from one experiment are shown
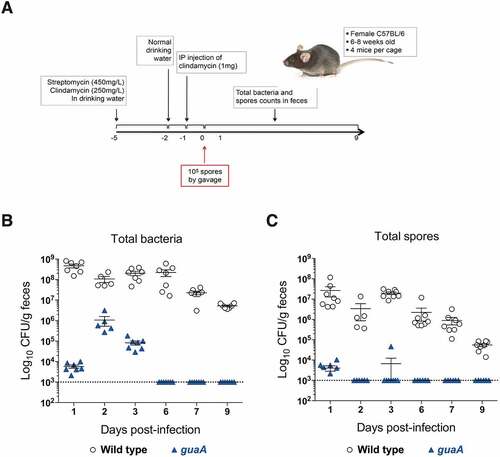
Discussion
In this study, we demonstrated the functionality of four guanine riboswitches identified in C. difficile using in-line probing, RT-qPCR and reporter gene assays. These riboswitches control the expression of guaA, xpt, CD630_21070 and CD630_27040, four genes associated with GMP biosynthesis and transport of purine metabolites. These riboswitches bind guanine in the low nanomolar range, and we expect these riboswitches to participate actively in the fine regulation of the expression of the corresponding genes. We also provide experimental evidence showing the importance of de novo GMP biosynthesis in C. difficile. Indeed, inactivation of guaA encoding a GMP synthase led to severe growth defects under nutrient-limiting growth conditions, while growth was relatively unaffected under rich conditions. In vivo infection assays further demonstrated that guaA inactivation caused a drastic reduction in competitiveness and colonization capacity of C. difficile in a mouse model.
Until now, the importance of guaA in C. difficile was predicted from experiments in which non-metabolizable guanine analogues inhibited growth of the bacterium in vitro [Citation21]. Similar observations were also made with S. aureus, and in the latter case demonstration of the efficacy of guanine analogs in treating mastitis in mice and cows was made [Citation21,Citation26]. The overall conclusion was that guanine analogues could block the expression of guaA through the associated riboswitch, thereby forcing bacteria to exhaust their GMP pool, leading to cell death [Citation21]. However, this conclusion was later challenged by another study reporting that riboswitch-independent transcription of the guaA and guaB genes was also possible through alternative promoters. The antibacterial activity of guanine analogues was therefore attributed to off-target effects rather than the direct inhibition of the guanine riboswitch [Citation19]. Inactivation of guaA had never been done in C. difficile and our study thus provides the first direct experimental demonstration of the importance of guaA and GMP biosynthesis for growth of C. difficile under limiting conditions, as well as during infection. Previous studies have shown that inactivation of guaA encoding the GMP synthase results in reduced virulence of Salmonella in a mouse model of infection [Citation27]. Likewise, inactivation of guaA in E. coli is associated with guanine auxotrophy [Citation17] and the inability of the bacterium to grow in urine in vitro, with a 2–3 log loss of viability after 6 h. In addition, a guaA mutant was significantly less virulent in a mouse model of urinary tract infection [Citation28]. Similar conclusions were also reported for S. aureus, in which inactivation of guaA or guaB led to guanine auxotrophy, failure to grow in human serum, caused cellular morphology defects and was associated with avirulence in mice models of infection [Citation19]. Interestingly, inactivation of the salvation pathway genes xpt or pbuX did not cause any of these defects. Hence, de novo guanine biosynthesis seems to be more important than the salvation pathways during S. aureus infection, due to limited guanine availability in vivo. It thus appears that de novo biosynthesis of GMP is also crucial for full virulence of C. difficile in the gut.
One interesting aspect of guanine riboswitches in C. difficile is that each of them is associated with only one gene predicted to participate to GMP biosynthesis and transport of purine precursors such as xanthine and hypoxanthine. In other clostridia such as Clostridium acetobutylicum, Clostridium beijerinckii and Clostridium botulinum, guanine riboswitches control an xpt-pbuX operon associated with xanthine metabolism and transport on the one hand, and a guaB-guaA operon associated with GMP biosynthesis from purine IMP and XMP on the other hand. This gene organization suggests co-regulation of transporters and enzymes associated with specific metabolic pathways by guanine-responsive riboswitches. In B. subtilis, four guanine riboswitches control either single genes (e.g. pbuG or yxjA) or transcriptional units associated with purine metabolism, like the xpt-pbuX and pur operons [Citation20]. In staphylococci, a simplified regulatory network for the control of GMP biosynthesis is observed, with a single guanine riboswitch controlling an operon comprising xpt, pbuX, guaB and guaA [Citation21]. However, in S. aureus, the identification of alternative promoters driving the transcription of guaB and guaA, independent from the guanine riboswitch, suggests a more complex regulation than initially reported [Citation19].
The inactivation mutants in C. difficile showed that only guaA seems essential under poor growth conditions. Inactivation of the xanthine phosphoribosyl transferase (Xpt) or the two transporters (21070 and 27040) did not impact growth of C. difficile in vitro under the conditions tested. In these mutants, other alternative routes such as the purine salvation pathway are probably available to provide XMP to feed the GMP synthase (). We did not test double or triple mutants, so we cannot predict the consequence of the inactivation of one or the two transporters and xpt altogether, but the net result would possibly be similar to single mutants; salvation pathways would probably take the relay. The genome of C. difficile 630 also contains several other putative transporters of purine and related metabolites that are not associated with riboswitches, like CD630_20750 (pbuX, a putative xanthine permease), CD630_20900 and CD630_20910 (two putative xanthine/uracil permeases), CD630_25940 (uraA, putative uracil permease), and CD630_31800 (putative purine permease). Although the functional characterization of these transporters is not available, we can expect that they participate in the transport of these metabolites. It is therefore not surprising that inactivation of 21070 and 27040 did not lead to growth defects in vitro. In S. aureus, only guaA and guaB seem to be essential for growth and survival in vitro and in vivo, and inactivation of the salvation pathway genes xpt and pbuX has no significant impact [Citation19].
The in-line probing data showed that the guanine riboswitches in C. difficile bind guanine and purine analogs in the low nanomolar range, which is consistent with values reported for other bacteria like B. subtilis, in which the guanine riboswitch controlling the xpt-pbuX operon has an apparent Kd value of ≤ 5 nM [Citation20]. Regions of the guanine aptamer, the so-called ‘Tune-Box’, can accommodate small differences in the unstructured and ligand-free form [Citation29]. Variations in the nucleotide composition of the tune-box can modify the affinity of a guanine riboswitch aptamer for its ligand. In fact, one of the hallmarks of riboswitches is their ability to discriminate between closely related metabolites, to avoid regulatory crosstalk between different metabolic pathways. For example, the TPP riboswitch from E. coli binds thiamine pyrophosphate (TPP) with a ~ 1,000-fold greater affinity than thiamine phosphate (TP) or thiamine [Citation30]. In B. subtilis, the Kd value of the xpt-pbuX guanine riboswitch aptamer towards xanthine and hypoxanthine is ~ 50 nM, which is also 10 times higher than for guanine [Citation20]. We expected that the guanine riboswitches in C. difficile would have different affinities towards guanine and purine analogs due to the differences in their aptamer sequences. Although the differences in Kd values were small, we did observe some differences in the affinity towards guanine, xanthine and guanosine. In particular, the riboswitches controlling the expression of the two enzymes GuaA and Xpt were sensitive to the presence of guanine, whereas the riboswitch controlling the transporter 27040 did not respond to guanine but was sensitive to xanthine mainly. The apparent discrepancy between in-line probing and reporter assays with guanine might be explained by the fact that only the aptamer was analysed by in-line probing, whereas the full riboswitch (aptamer + expression platform) was included in our reporter assays. Ligand binding to the aptamer is not determined by the same characteristics than that of the riboswitch. For example, ligand binding to the riboswitch may depend on the cotranscriptional context that has been shown to impose a ligand binding window, thereby changing the behaviour of the riboswitch in bacteria [Citation31–33]. Also, the addition of guanine in our reporter assays did not lead to complete repression of the riboswitch, and a ≈ twofold repression was observed with guaA and xpt. Different reasons could explain this. For example, the reporter gene was expressed from a multi-copy plasmid, as opposed to studies conducted in B. subtilis and S. aureus where only one copy of the reporter gene is generally integrated into the chromosome [Citation19]. The concentration of guanine required to reach saturation of the riboswitches is therefore expected to be higher. The exogenously added guanine might also be rapidly incorporated into metabolic pathways to sustain growth, thereby limiting the interaction with the guanine riboswitches. Finally, endogenous guanine levels might be already high enough in the minimal growth medium used to partially repress the riboswitches.
Targeting riboswitches with small molecules has great potential in the search of new antibacterial drugs [Citation4]. Our study suggests that disruption of guaA expression reduces virulence and competitiveness as well as sporulation of C. difficile in the gut. As such, targeting the riboswitch controlling guaA with non-metabolizable guanine analogues could be a promising therapeutic strategy. The metabolism of purines and GMP is a complex multistep process involving multiple genes and transporters and inactivation of guaA could also lead to multiple impacts that need to be further investigated, in particular in vivo. For instance, variations in intracellular pools of guanine metabolites like GTP could lead to pleiotropic effects mediated by the master transcriptional regulator CodY, which plays a central role in the metabolism and virulence of several Gram-positive bacteria [Citation34]. CodY regulates more than 500 genes in C. difficile [Citation34] and is also known to inhibit sporulation and toxin production under nutrient-rich conditions and during the exponential growth phase. Repression mediated by CodY is stimulated by branched-chain amino acids and GTP that act as corepressors [Citation34]. Under nutrient limiting conditions, or upon entry into stationary phase, GTP levels drop and CodY becomes gradually inactive, thereby releasing the expression of several genes and contributing to sporulation initiation and toxin production [Citation35]. Hence, in a guaA mutant grown under nutrient-limiting conditions, we would expect CodY to be partially inactive due to reduced GTP pools, and the expression of several genes would therefore be derepressed, including sporulation-associated genes [Citation36]. However, we did not observe increased sporulation of the guaA mutant during infection in mice, suggesting that the impacts of guaA inactivation possibly surpass the influence of CodY. In agreement with this, sporulation of a codY mutant in C. difficile strain 630Δerm (the same strain as the one we used here) was only twofold higher than the parent strain [Citation36], suggesting that the influence of CodY on sporulation in this strain is limited. We are still far from a clear picture of the system, but our study provides highly useful data on the importance of de novo GMP biosynthesis in C. difficile.
Materials and methods
Operon analysis
The 2,570 riboswitch sequences were acquired from Rfam database (http://rfam.xfam.org/) and the annotations of the genes following them were obtained from NCBI databases (https://www.ncbi.nlm.nih.gov/).
First, 20,000 nt following the riboswitch start position were fetched from the corresponding genomic sequence. A distance inferior to 200 nt was allowed between genes from the same orientation to be included as part of the same operon. To be considered as regulated by a riboswitch, a distance inferior to 300 nt was allowed between the 5ʹ end of the riboswitch and the AUG codon of the first downstream gene. The riboswitch-associated taxonomy was traced back using Entrez Direct tools to fetch accession numbers through the NCBI database. All other analyses and parsing were performed using homemade scripts (Python 3.7 and Bash), which are available upon request.
Bacterial strains and growth conditions
C. difficile strain 630Δerm and the Escherichia coli CA434 donor strain used for conjugation were kind gifts from Nigel Minton (Nottingham, UK). C. difficile was grown under anaerobic atmosphere (10% H2, 5% CO2 and 85% N2) at 37°C using a Coy Laboratory anaerobic chamber (Coy Laboratory). C. difficile was routinely grown in brain heart infusion (BHI) (BD Biosciences), BHIS (BHI supplemented with 2% yeast extract and 1% L-cysteine) or TY broth (3% tryptose, 2% yeast extract, pH 7.4). For agar plates, 1.5% agar was added to the above media. Mueller Hinton broth (cation-adjusted) (MHBCA; BD Biosciences) and a minimal defined medium (MDM) were also used in specific experiments [Citation25]. E. coli CA434 was routinely grown in Luria-Bertani (LB) broth or agar (BioShop). When needed, antibiotics were added to media at the following concentrations: Kanamycin 100 µg/mL, norfloxacin 12 µg/mL, thiamphenicol 15 µg/mL, or erythromycin 2.5 µg/mL. A list of the strains and plasmids used in this study can be found in Supplementary Table S1.
Gene inactivation and complementation in C. difficile
The guaA, xpt, CD630_21070 and CD630_27040 genes were inactivated in C. difficile 630Δerm using the ClosTron technology [Citation24]. ClosTron constructs were ordered through the DNA 2.0 Company (CA, USA) and transferred by conjugation into C. difficile as described elsewhere [Citation37]. Selection of integrants was done with erythromycin and confirmation of the interruption of the target gene was done by PCR with primers flanking the integration site (Supplementary Table S2). For complementation assays, the guaA gene was cloned into the SacI and BamHI sites of the shuttle plasmid pRPF144 [Citation23] in place of the gusA reporter gene, leading to creation of pSMI5 (guaA), and then transferred by conjugation from E. coli CA434 into the appropriate C. difficile mutant strain.
Growth curve experiments
A 5-mL tube of BHI broth was inoculated from an isolated colony grown on BHI agar and incubated O/N at 37°C anaerobically. The next morning, another 5-mL tube of fresh BHI was inoculated at 2% with the pre-culture and bacteria were grown until the optical density at 600 nm (OD600) reached ~0.5. Then, bacteria were centrifuged at 3,700 g for 10 min, washed in 5 mL of pre-reduced PBS and centrifuged again. Bacteria were then inoculated at an initial density of ~107 CFU/mL in the indicated broth and incubated at 37°C anaerobically. Samples were collected at various time points, and serial dilutions were plated on MHBCA plates. Colonies were counted after overnight incubation to determine the number of CFU/mL.
In-line probing assays
For the production of guanine riboswitch aptamers for in-line probing assays, DNA templates were prepared from partial duplexes and transcribed using T7 RNA polymerase as previously described [Citation38]. The aptamer sequences used in this study were based on the genomic sequence from strain 630Δerm (accession No. AM180355), to which a GCG sequence was added to the 5′ extremity to allow high transcription yield and to minimize the 5′ heterogeneity. In-line probing assays were done following standard procedures as previously described [Citation22]. Briefly, [5′-32P]-labelled RNA molecules were incubated for 48 h at 25°C in 50 mM Tris-HCl buffer, pH 8.5, 20 mM MgCl2 and 100 mM KCl in the absence or presence of the indicated ligand concentrations. The reactions were stopped with a 97% formamide solution containing 10 mM EDTA, xylene cyanol and bromophenol blue and samples were separated by electrophoresis in 8% polyacrylamide gels (acrylamide:bisacrylamide; 19:1) containing 8 M urea. Gels were dried, exposed to Phosphor Imager screens and scanned on a Typhoon FLA9500 (GE health care) and analysed with the ImageQuant software to measure the intensity of bands of interest.
GusA reporter assays
Transcriptional fusions of the guanine riboswitches and the gusA reporter gene were constructed as follows. The pRPF144 plasmid [Citation23] was first modified by introducing a multiple cloning site (MCS) amplified by PCR from the pMTL84153 plasmid [Citation39], using primers LCF837 and LCF838 (Supplementary Table S2). The MCS was then cloned in reverse orientation into the SacI site of pRPF144, creating the plasmid pLOS144. This plasmid was then used to clone the different riboswitch aptamers, transcriptionally fused to the gusA reporter gene. The aptamer region of each riboswitch was amplified by PCR using primers listed in Supplementary Table S2. The amplicons were then digested and cloned into the SacI site of pLOS144, creating plasmids pSMI1, pSMI2, pSMI3 and pSMI4 containing the riboswitch from guaA, xpt, 27040 and 21070, respectively (Supplementary Table S1). The GusA activity was measured in MDM supplemented with the indicated metabolites, using a modified version of the procedure described in Mani et al. [Citation40]. Briefly, a pre-culture of C. difficile carrying the various plasmids was done in MDM + thiamphenicol 15 µg/mL until the OD600 reached 0.7, at which point cells were centrifuged and washed in reduced PBS, followed by resuspension in MDM ± the metabolites. Cells were incubated for 2 h at 37°C under anaerobic conditions, and 1-mL samples were collected by centrifugation (10,000 g for 5 min), suspended in Z-buffer (60 mM Na2HPO4 · 7H2O [pH 7.0], 40 mM NaH2PO4 · H2O, 10 mM KCl, 1 mM MgSO4 · 7H2O, 50 mM 2-mercaptoethanol), treated for 1 h at 37°C with an endolysin preparation to disrupt the peptidoglycan cell wall, and lyzed by sonication. The determination of the GusA activity was then made by combining 0.1 mL of lysate with 0.1 mL of Z-buffer in a 96-well plate, and by adding 40 µL of a solution of 6 mM p-nitrophenyl-β-D-glucuronide [Citation40]. The specific activity was determined by reporting the β-glucuronidase units in function of the total protein concentration of each sample, as determined by Bradford quantification.
Determination of C. difficile competitiveness in a mouse model of infection
All experiments were performed with 6 to 8 weeks old C57BL/6 female mice (15.5 g to 19 g), purchased from Charles River Laboratories. Manipulations were done in accordance with the Canadian Council on Animal Care and the protocol was approved by the animal ethics review board of the faculty of medicine and health sciences of the Université de Sherbrooke. Mice were housed in sterile cages equipped with HEPA filters and sterile bedding, fed with sterile food and water ad libitum. Mice were made susceptible to C. difficile infection by the addition of 450 mg/L streptomycin and 250 mg/mL clindamycin in their drinking water for 3 days. Then, a single intraperitoneal dose of clindamycin (1 mg/mouse) was given 24 h before gavage with 105 spores of wild-type C. difficile 630Δerm and 105 spores of the guaA inactivated mutant strain, in 0.1 mL of water. Spores were prepared as described before [Citation41]. Clinical signs of infection were monitored twice a day (diarrhoea, hunched back, lethargy) and mice were weighed daily. Fresh faecal samples were collected directly from the animal anus at different time points, and quickly homogenized in reduced PBS under anaerobic conditions. During the acute infection period, some mice had diarrhoea and did not produce collectable faeces and thus some of the samples could not be analysed for bacterial counts. C. difficile total cell counts were determined by plating serial dilutions of the faecal suspensions on BHI agar plates containing 8 µg/mL cefoxitin + 12 µg/mL norfloxacin to select for C. difficile cells, as well as 0.1% taurocholic acid and 1 mM glycine to favour spore germination (BHI-CN-TAG) [Citation41]. To count spores, faecal suspensions were treated for 1 h with 50% ethanol and serial dilutions were plated on BHI-CN-TAG plates. To discriminate between wild-type and guaA mutant cells, erythromycin was added to the agar plates; only the mutant strain grew due to the presence of an erythromycin cassette introduced with the group II intron of the ClosTron. Bacterial counts were determined after overnight incubation at 37°C under anaerobic atmosphere and were reported as colony forming units (CFU) per gram of faeces.
Supplemental Material
Download PDF (1.6 MB)Acknowledgments
This research was enabled in part by support provided by Calcul Québec and Compute Canada. We would like to thank Audrey Bioteau for bioinformatics support and for the development of Entrez Fetcher allowing efficient fetching of taxonomic metadata from the NCBI database.
Data availability
Strains and plasmids used in this study are available upon request. The Entrez_fetch program is publicly available through GitLab (https://gitlab.com/celliern/entrez_fetch/-/archive/master/entrez_fetch-master.zip)
Supplementary material
Supplemental data for this article can be accessed here
Additional information
Funding
References
- Guery B, Galperine T, Barbut F. Clostridioides difficile: diagnosis and treatments. British Med J. 2019;366:l4609.
- Cho JM, Pardi DS, Khanna S. Update on Treatment of Clostridioides difficile Infection. Mayo Clin Proc. 2020;95:758–769.
- Rajasingham R, Enns EA, Khoruts A, et al. Cost-effectiveness of Treatment Regimens for Clostridioides difficile Infection: an Evaluation of the 2018 Infectious Diseases Society of America Guidelines. Clin Infect Dis. 2019;70:754–762.
- Lünse CE, Schüller A, Mayer G. The promise of riboswitches as potential antibacterial drug targets. Int J Med Microbiol. 2014;304:79–92.
- Blount KF, Breaker RR. Riboswitches as antibacterial drug targets. Nat Biotechnol. 2006;24:1558–1564.
- Sudarsan N, Barrick JE, Breaker RR. Metabolite-binding RNA domains are present in the genes of eukaryotes. RNA. 2003;9:644–647.
- Greenlee EB, Stav S, Atilho RM, et al. Challenges of ligand identification for the second wave of orphan riboswitch candidates. RNA Biol. 2018;15
- Weinberg Z, Lünse CE, Corbino KA, et al. Detection of 224 candidate structured RNAs by comparative analysis of specific subsets of intergenic regions. Nucleic Acids Res. 2017;45:10811–10823.
- Serganov A, Nudler E. A Decade of Riboswitches. Cell. 2013;152:17–24.
- McCown PJ, Corbino KA, Stav S, et al. Riboswitch diversity and distribution. RNA. 2017;23:995–1011.
- Bastet L, Dubé A, Massé E, et al. New insights into riboswitch regulation mechanisms. Mol Microbiol. 2011;80:1148–1154.
- Hall CL, Lee VT. Cyclic‐di‐GMP regulation of virulence in bacterial pathogens. Wiley Interdiscip Rev RNA. 2018;9:e1454.
- Christiansen LC, Schou S, Nygaard P, et al. Xanthine metabolism in Bacillus subtilis: characterization of the xpt-pbuX operon and evidence for purine- and nitrogen-controlled expression of genes involved in xanthine salvage and catabolism. J Bacteriol. 1997;179:2540–2550.
- Ebbole DJ, Zalkin H. Cloning and characterization of a 12-gene cluster from Bacillus subtilis encoding nine enzymes for de novo purine nucleotide synthesis. J Biological Chem. 1987;262:8274–8287.
- Xi H, Schneider BL, Reitzer L. Purine Catabolism in Escherichia coli and Function of Xanthine Dehydrogenase in Purine Salvage. J Bacteriol. 2000;182:5332–5341.
- Mäntsälä P, Zalkin H. Cloning and sequence of Bacillus subtilis purA and guaA, involved in the conversion of IMP to AMP and GMP. J Bacteriol. 1992;174:1883–1890.
- Shimaoka M, Takenaka Y, Mihara Y, et al. Effects of xapA and guaA Disruption on Inosine Accumulation in Escherichia coli. Biosci Biotechnol Biochem. 2014;70:3069–3072.
- Kotloff KL, Pasetti MF, Barry EM, Nataro JP, Wasserman SS, Sztein MB, Picking WD, Levine MM. Deletion in the Shigella Enterotoxin Genes Further Attenuates Shigella flexneri 2a Bearing Guanine Auxotrophy in a Phase 1 Trial of CVD 1204 and CVD 1208. J Infect Dis. 2004;190:1745–1754.
- Kofoed EM, Yan D, Katakam AK, et al. De Novo Guanine Biosynthesis but Not the Riboswitch-Regulated Purine Salvage Pathway Is Required for Staphylococcus aureus Infection In Vivo. J Bacteriol. 2016;198:2001–2015.
- Mandal M, Boese B, Barrick JE, et al. Riboswitches Control Fundamental Biochemical Pathways in Bacillus subtilis and Other Bacteria. Cell. 2003;113:577–586.
- Mulhbacher J, Brouillette E, Allard M, et al. Novel Riboswitch Ligand Analogs as Selective Inhibitors of Guanine-Related Metabolic Pathways. Plos Pathog. 2010;6:e1000865.
- Regulski EE, Breaker RR. In-line probing analysis of riboswitches. In: Wilusz J, editor. Post-Transcriptional Gene Regulation. Methods Mol Biol. Vol. 419. Humana Press; 2008. p. 53–67.
- Fagan RP, Fairweather NF. Clostridium difficile Has Two Parallel and Essential Sec Secretion Systems*. J Biol Chem. 2011;286:27483–27493.
- Heap JT, Pennington OJ, Cartman ST, et al. The ClosTron: a universal gene knock-out system for the genus Clostridium. J Microbiol Methods. 2007;70:452–464.
- Karlsson S, Burman LG, Åkerlund T. Suppression of toxin production in Clostridium difficile VPI 10463 by amino acids. Microbiology+. 1999;145:1683–1693.
- Ster C, Allard M, Boulanger S, et al. Experimental treatment of Staphylococcus aureus bovine intramammary infection using a guanine riboswitch ligand analog. J Dairy Sci. 2013;96:1000–1008.
- McFarland WC, Stocker BAD. Effect of different purine auxotrophic mutations on mouse-virulence of a Vi-positive strain of Salmonella dublin and of two strains of Salmonella typhimurium. Microbiol Pathogen. 1987;3:129–141.
- Russo TA, Jodush ST, Brown JJ, et al. Identification of two previously unrecognized genes (guaA and argC) important for uropathogenesis. Mol Microbiol. 1996;22:217–229.
- Stoddard CD, Widmann J, Trausch JJ, et al. Nucleotides Adjacent to the Ligand-Binding Pocket are Linked to Activity Tuning in the Purine Riboswitch. J Mol Biol. 2013;425:1596–1611.
- Winkler W, Nahvi A, Breaker RR. Thiamine derivatives bind messenger RNAs directly to regulate bacterial gene expression. Nature. 2002;419:952–956.
- Chauvier A, Picard-Jean F, J-c B-D, et al. Transcriptional pausing at the translation start site operates as a critical checkpoint for riboswitch regulation. Nat Commun. 2017;8:13892.
- Wickiser JK, Winkler WC, Breaker RR, et al. The Speed of RNA Transcription and Metabolite Binding Kinetics Operate an FMN Riboswitch. Mol Cell. 2005;18:49–60.
- Wickiser JK, Cheah MT, Breaker RR, et al. The Kinetics of Ligand Binding by an Adenine-Sensing Riboswitch. Biochemistry-US. 2005;44:13404–13414.
- Daou N, Wang Y, Levdikov VM, et al. Impact of CodY protein on metabolism, sporulation and virulence in Clostridioides difficile ribotype 027. Plos One. 2019;14:e0206896.
- Dineen SS, McBride SM, Sonenshein AL. Integration of Metabolism and Virulence by Clostridium difficile CodY. J Bacteriol. 2010;192:5350–5362.
- Nawrocki KL, Edwards AN, Daou N, et al. CodY-Dependent Regulation of Sporulation in Clostridium difficile. J Bacteriol. 2016;198:2113–2130.
- Sekulovic O, Fortier L-C. Global Transcriptional Response of Clostridium difficile Carrying the ϕCD38-2 Prophage. Appl Environ Microb. 2015;81:1364–1374.
- Mulhbacher J, Lafontaine DA. Ligand recognition determinants of guanine riboswitches. Nucleic Acids Res. 2007;35:5568–5580.
- Heap JT, Pennington OJ, Cartman ST, et al. A modular system for Clostridium shuttle plasmids. J Microbiol Methods. 2009;78:79–85.
- Mani N, Lyras D, Barroso L, et al. Environmental Response and Autoregulation of Clostridium difficile TxeR, a Sigma Factor for Toxin Gene Expression. J Bacteriol. 2002;184:5971–5978.
- Garneau JR, Valiquette L, Fortier L-C. Prevention of Clostridium difficile spore formation by sub-inhibitory concentrations of tigecycline and piperacillin/tazobactam. BMC Infect Dis. 2014;14:29.