ABSTRACT
Nsp1 of SARS-CoV-2 regulates the translation of host and viral mRNAs in cells. Nsp1 inhibits host translation initiation by occluding the entry channel of the 40S ribosome subunit. The structural study of the Nsp1-ribosomal complexes reported post-termination 80S complex containing Nsp1, eRF1 and ABCE1. Considering the presence of Nsp1 in the post-termination 80S ribosomal complex, we hypothesized that Nsp1 may be involved in translation termination. Using a cell-free translation system and reconstituted in vitro translation system, we show that Nsp1 stimulates peptide release and formation of termination complexes. Detailed analysis of Nsp1 activity during translation termination stages reveals that Nsp1 facilitates stop codon recognition. We demonstrate that Nsp1 stimulation targets eRF1 and does not affect eRF3. Moreover, Nsp1 increases amount of the termination complexes at all three stop codons. The activity of Nsp1 in translation termination is provided by its N-terminal domain and the minimal required part of eRF1 is NM domain. We assume that the biological meaning of Nsp1 activity in translation termination is binding with the 80S ribosomes translating host mRNAs and remove them from the pool of the active ribosomes.
KEYWORDS:
Introduction
Covid-19 is continuing to spread around the world. The causative agent of COVID-19 is Severe Acute Respiratory Syndrome Coronavirus 2 (SARS-CoV-2), which belongs to the beta-coronavirus group [1]. Its genome consists of a single-stranded (+) RNA, approximately 30,000 nt long, containing 14 open reading frames (ORFs) encoding 27 proteins. The two overlapping 5ʹ-end frames ORF1a and ORF1b encode large polypeptides (490 and 794 kDa, respectively), which are subsequently proteolytically cleaved by viral proteases (Nsp3 and Nsp5), generating the unstructured proteins Nsp1-Nsp16. The ORF1b polypeptide synthesis occurs via a − 1 frameshift just before the ORF1a stop codon. The RNA-dependent RNA polymerase NSP12 encoded within the ORF1b ensures the replication of the viral genome, also producing additional truncated RNA products with open reading frames for the synthesis of the remaining SARS-CoV-2 proteins [Citation2–4]. mRNA coding polyproteins contains a full-sized 265 nt long 5ʹUTR of SARS-CoV-2, and the additional mRNAs contain 70–75 distal nt of 5ʹUTR [Citation1Citation2]. It has been shown that among all SARS-CoV-2 proteins, Nsp1 is a major viral factor that affects cellular viability [Citation5].
Nsp1 is the first protein produced by a virus. It affects the vital activity of the infected cell and is necessary for the development of viral particles. Nsp1 induces apoptosis, and alters the transcriptional profile of cells by blocking the transcription of genes responsible for cell metabolism, regulation of the cell cycle, mitochondrial function, antigen presentation, ubiquitin/proteasome pathways, and protein synthesis [Citation5]. Moreover, Nsp1 directly inhibits translation both in vitro in the cell-free translation systems and in vivo in the cells [Citation5–9], although it does not affect elongation of protein synthesis [Citation10–12]. Nsp1-mediated inhibition of translation leads to suppression of the host interferon response, which is one of the main mechanisms of cellular defence against viral replication [Citation6,Citation13,Citation14]. Additionally, Nsp1 suppresses mRNA export from the nucleus via binding to the receptor of mRNA export NXF1-NXT1 [Citation15]. It was shown also that Nsp1 causes rapid depletion of the host mRNA pool in cells due to a significant reduction in their half-life [Citation10,Citation12,Citation16].
Therewith, viral mRNAs are less susceptible to translation inhibition due to the presence of an SL1 hairpin in their 5ʹUTR [Citation6,Citation8,Citation9]. SL1 interacts with Nsp1 and significantly increases translation of viral mRNA. Recently, it was revealed that secondary structure of SL1 hairpin does not prevent translation suppression by Nsp1 [Citation12,Citation17]. It was shown that certain motifs in the primary SL1 structure (presence of seven pyrimidines in positions 15–21 and absence of guanosine in positions 9–22) are important for the significant translation activation of viral mRNA by Nsp1. These sequences become available after melting of SL1 by helicases [Citation12]. Interestingly, the translation of the host mRNAs enriched in their 5ʹ-leaders by polypyrimidine tracts is less inhibited by Nsp1. Among them there are mRNA encoding components of the translation apparatus, RNA-binding proteins and other, important for viral pathogenicity [Citation11]. Additionally, the full-sized 5ʹUTR of the SARS-CoV-2 mRNA has IRES-like activity increasing in the presence of Nsp1 [Citation12]. SL1 region of the SARS-CoV-2 mRNA is considered as promising for COVID-19 therapy, for example, using the appropriate locked nucleic acid antisense oligonucleotides (LNA ASOs) [Citation18,Citation19].
Nsp1 of the SARS-CoV-2 virus is a small protein of 180 amino acid residues, consisting of N- and C-terminal domains connected by an unstructured linker [Citation3,Citation8]. The N-domain of this protein interacts with the 5ʹUTR of SARS-CoV-2 mRNA, which is necessary for its efficient translation [Citation8]. Mutations in the N-domain also lead to a decrease in the level of apoptosis of infected cells [Citation5]. The C-domain of Nsp1 binds to the entry channel of the 40S ribosome subunit. In this case, the 40S subunit associated with Nsp1 is unable to form a native translation initiation complex and bind mRNA. At the same time, it was shown that Nsp1 is unable to bind to the entry channel of the 40S ribosome subunit bound with mRNA [Citation20]. Thus, Nsp1 inhibits host mRNA translation [Citation5–9,Citation13,Citation20]. In addition to free 40S subunits, Nsp1 SARS-CoV-2 is also found in aberrant 48S initiator complexes and 80S ribosomes [Citation5,Citation7,Citation13,Citation20]. It should be noted that binding to the 40S subunit of homologous Nsp1 in closely related SARS-CoV does not block the formation of the 48S initiator complex but inhibits the attachment of the 60S subunit to it and causes inhibition of the translation initiation [Citation21]. Some of the 80S ribosomes with Nsp1 SARS-CoV-2 contain additional proteins involved in translation termination and ribosome recycling, i.e. the eukaryotic release factor 1 (eRF1) and ATP-binding cassette sub-family E member 1 (ABCE1) [Citation13]. This prompted us to address the possible role of Nsp1 in translation termination.
Translation termination is the final stage of protein biosynthesis, in which the nascent polypeptide releases from the ribosome. Translation termination begins when one of the three stop codons appears in the A site of the ribosome [Citation22]. The ribosome then binds eRF1 that recognizes all three stop codons [Citation23–27]. A partner protein of eRF1 is eRF3, the GTPase that activates eRF1 [Citation28–32]. Binding of the eRF1-eRF3-GTP complex with the ribosome stimulates GTPase activity of eRF3, which, in turn, causes a conformational change of eRF1 [Citation29]. As a result, the catalytic GGQ motif of eRF1 is positioned in the peptidyl transferase centre of the ribosome. This induces the hydrolysis of peptidyl-tRNA and the release of the synthesized protein [Citation22,Citation23,Citation26–28,Citation33]. Termination of translation is one of the critical stages of protein biosynthesis because its suppression inhibits both the release of peptides from the ribosomes and the transition of the ribosomes to recycling, preventing new rounds of translation.
The simultaneous presence of the eRF1 and Nsp1 in the 80S ribosomal complexes [Citation13] implies a possible role of Nsp1 in translation termination, which has not been elucidated yet. To study an effect of Nsp1 on the termination of host translation, we used the reconstituted mammalian translation system [Citation28] as well as pre-termination complexes (preTCs) purified from rabbit reticulocyte lysate (RRL) [Citation34]. We demonstrate that Nsp1 of SARS-CoV-2 stimulates translation termination. Moreover, we determine the stage of termination at which Nsp1 works. Point mutations allowed to find region of Nsp1 involved in translation termination. On the basis of the obtained data we propose a possible model of regulation of translation by SARS-CoV-2 Nsp1.
Results
Nsp1 stimulates peptide release
To study the activity of SARS-CoV-2 Nsp1 in translation termination, we first verified the ability of the recombinant Nsp1 to inhibit translation. For this purpose, Nluc mRNA was translated in RRL in the presence of Nsp1 (). We confirmed that our preparation of the recombinant tag-free Nsp1 significantly inhibited the translation of Nluc mRNA in the cell-free system.
Figure 1. Nsp1 activates peptidyl-tRNA hydrolysis with the release factors. (A) In vitro Nluc mRNA translation in RRL in the presence/absence of Nsp1. (B) Termi-Luc peptide release assay in the presence/absence of Nsp1 and the release factors. GST was added to the reaction as a negative control. Time progress curves showing luminescence (in relative luminescence units, rlu) with NLuc released from the ribosome complex upon treatment with the proteins of interest, number of repeats, n = 3, mean±SD
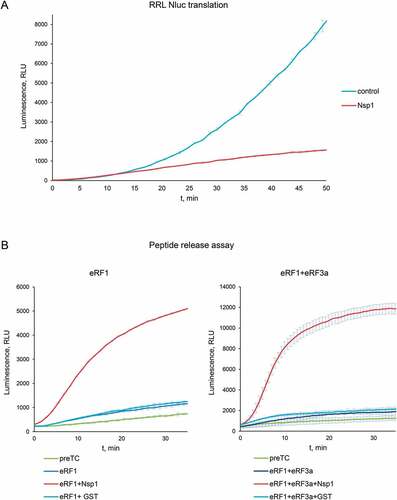
Next, we evaluated a possible effect of Nsp1 on translation termination. The result of translation termination is the hydrolysis of peptidyl-tRNA and the release of the nascent peptide from the ribosome. We performed an analysis of a peptide release efficiency using the Termi-Luc approach (Fig. S1) developed recently by Susorov et al. [Citation34]. This system synthesizes NanoLuc luciferase (Nluc) but does not release it from the ribosome those preventing its activity. The level of release in the assay allows complete NLuc folding and the appearance of NLuc activity as a function of the efficiency of peptide release. Pretermination complexes (PreTCs), containing Nluc located at the P site of the ribosome in the peptidyl-tRNA form and UAA stop codon at the A site, were purified from the RRL. The addition of the release factors to the purified preTC triggers the peptidyl-tRNA hydrolysis and induces the release of Nluc from the ribosome. Human eRF1 and eRF3a, together with Nsp1, were added to the preTC, and luminescent signal was measured over time. We observed that Nsp1 was unable to perform peptide release by itself, however it significantly stimulates translation termination induced by eRF1 alone and by the complex of eRF1 and eRF3a (, S2). As a negative control we used Glutathione-S-transferase (GST), which is of a similar size and does not involved in any translation stages.
To study the effect of Nsp1 on luciferase activity per se, we added it to the peptide release reaction twice at the log and stationary phases of the growth curve. To conduct this experiment, we used saturating concentrations of release factors to maximize the amount of the Nluc release and to neutralize the effect of Nsp1 on translation termination (Fig. S3A). We observed that the addition of Nsp1 did not change the luminescent signal and consequently did not affect the activity of Nluc.
Obtained results of Nsp1 activity in peptide release allowed us to conclude that the presence of Nsp1 in the 80S ribosomal complexes shown earlier [Citation13] reflects its functional involvement in translation termination.
Nsp1 stimulates the termination complexes formation
To study a molecular mechanism of the stimulation of translation termination by Nsp1, we tested its activity on the purified preTCs obtained in the reconstituted translation system [Citation28]. We assembled preTCs from individual components on the model MVHL mRNAs with the UAA stop codon and purified them by centrifugation in a sucrose density gradient. These complexes were then used to test the effect of Nsp1 on the formation of termination complexes (TCs) by fluorescent toe-print analysis, which shows the position of ribosomal complexes on mRNA. When stop codon is recognized by eRF1, the ribosome changes conformation of the +4 nucleotide following the stop codon, which is then detected as a nucleotide shift of the toe-print signal [Citation28,Citation35]. It should be noted that in each experiment we compared the relative amounts of cDNAs as areas of the preTC and the TC peaks according to the following formula TC formation efficiency = TC/(TC + preTC), which is presented in the histograms (). The examples of raw data obtained in this experiment are shown in Figure S4.
Figure 2. Nsp1 increases TC formation. Examples of raw toe-printing data and relative quantitative analysis of the TC formation efficiency induced by the release factors in the presence of Nsp1 or GST as a negative control. TC formation efficiency induced by release factors was taken as 1. (A) Toe-print analysis of TCs formed by addition to the preTCs of eRF1 and Nsp1. (B) Toe-print analysis of TCs formed by addition to the preTCs of eRF1(AGQ) and Nsp1. (C) Toe-print analysis of TCs formed by addition to the preTCs of eRF1+eRF3a+GTP and Nsp1. (D) Toe-print analysis of TCs formed by addition to the preTCs of eRF1(AGQ)+eRF3a+GTP and Nsp1. r.u. – relative units. Positions of preTCs and TCs are labelled by white and black triangles, respectively. Red stars indicate the increased quantity of ribosomal complexes, shifted from the preTC to the TC state. The error bars represent the standard error of mean, stars (**) mark a significant difference from the respective control P < 0.01 (n = 3)
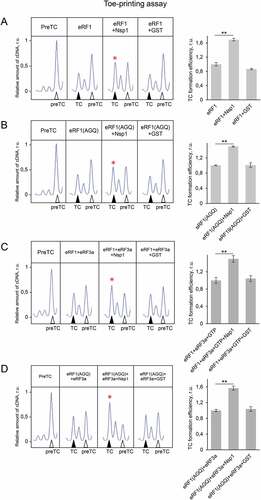
Toe-print analysis showed that the addition of Nsp1 to the preTC does not induce any shift of the preTC peak both in 0.5 and 2.5 µM concentration (Fig. S5A, S6B). However, addition of Nsp1 to the preTC with the release factor eRF1 increases the amount of the formed TC by 1,6 times (). As a negative control, we used GST. To determine the stage of termination during which Nsp1 activates TC formation, we tested the activity of the eRF1(AGQ) mutant, which is unable to induce hydrolysis of peptidyl-tRNA. Efficiency of the TC formation in the presence of eRF1(AGQ) reflects the efficiency of eRF1 binding with the stop codon irrespective of peptidyl-tRNA hydrolysis. We observed that TC formation performed by eRF1(AGQ) was similarly stimulated by Nsp1 ().
Additionally, Nsp1 stimulated the formation of the TC in the presence of both release factors (eRF1 and eRF3a) and GTP (). Likewise, in the presence of the eRF1(AGQ)-eRF3a complex, we observed significant stimulation of TC formation by Nsp1 (). Thus, we concluded that Nsp1 stimulates translation termination during TC formation before peptide release.
Nsp1 does not influence the GTPase activity of eRF3a
One of the stages of translation termination is the GTP hydrolysis performed by eRF3, which occurs after the binding of eRF1-eRF3 complex to the stop codon in the ribosome. This is followed by a conformational change of the TC and positioning of eRF1 in the peptidyl-transferase centre of the ribosome [Citation29]. To exclude effect of Nsp1 on the enzymatic activity of eRF3, we analysed the GTPase activity of eRF3a in the presence of eRF1, 80S ribosome, and Nsp1. We demonstrated no effect of Nsp1 on the hydrolysis of GTP (). The GTPase activity of eRF3a was determined at the concentrations of the components corresponding to the linear part of the saturation curve (Fig. S5B). We confirmed this finding by analysing the efficiency of TC formation in the presence of a non-hydrolysable GTP analogue, GDPCP, using toe-printing assay. The addition of the GDPCP to eRF1 and eRF3a did not prevent stimulation of TC formation by Nsp1 similar to that observed in the presence of GTP (, S6A). Therefore, Nsp1 stimulation activity in translation termination appears before and independently of GTP hydrolysis catalysed by eRF3.
Figure 3. Nsp1 stimulates translation termination independently of GTP hydrolysis. (A) GTPase activity of eRF3a in the presence of the ribosome subunits, eRF1 and Nsp1, number of repeats, n = 4, mean±SE. (B) Nsp1 stimulation of TC formation does not depend on GTP hydrolysis. TC formation was induced by the addition to preTC of eRF1+eRF3a+GDPCP and Nsp1 or GST as a negative control. TC formation efficiency induced by eRF1+eRF3a+GDPCP was taken as 1. The TC corresponds to the black triangle, and the preTC corresponds to the white triangle, r.u. – relative units. Red star indicates the increased quantity of ribosomal complexes, shifted from the preTC to the TC state. The error bars represent the standard error of mean, stars (**) mark a significant difference from the respective control P < 0.01 (n = 3). (C) Termi-Luc peptide release assay in the presence/absence of Nsp1 and NM-eRF1 or eRF1. Time progress curves showing luminescence (in relative luminescence units, rlu) with NLuc released from the ribosome complex upon treatment with the proteins of interest, number of repeats, n = 3, mean±SD
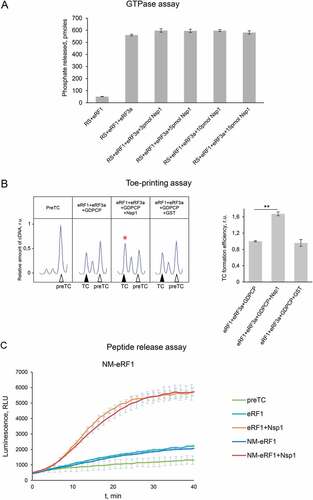
eRF1 interacts with eRF3 via its C-terminal domain, and its N-terminal part, NM domain, is active in translation termination similarly to full-sized eRF1 [Citation25]. To study effect of the C-terminal domain of eRF1 on Nsp1 activity in termination, we performed peptide release experiment induced by NM-eRF1 in the presence of Nsp1. We observed that Nsp1 stimulates activity of NM-eRF1 similarly to full-sized eRF1 (). This once again confirms our conclusion that Nsp1 does not affect eRF3, and the stimulation of translation termination by Nsp1 occurs on the stage of stop codon recognition by eRF1.
Effects of mutations of Nsp1 on its activity in translation termination
To find critical for termination activity regions of Nsp1, we obtained five mutant forms of Nsp1: K164A/H165A (KH/AA), Y154A/F157A (YF/AA), R171E/R175E (RR/EE), R124S/K125E (RK/SE), and N128S/K129E (NK/SE), described previously as mutants that lack an activity in translation or mutants that disrupt cell apoptosis () [Citation5,Citation7,Citation13]. For KH/AA, YF/AA and RR/EE it was shown that these mutants disrupt interaction of Nsp1 with 40S [Citation5,Citation7,Citation13]. Mutants RK/SE and NK/SE were described as significantly disrupting suppressive effect of Nsp1 to interferon response.
Figure 4. Activity of the Nsp1 mutants in translation termination. (A) Scheme of the Nsp1 forms used in the experiments. (B) In vitro Nluc mRNA translation in RRL in the presence/absence of Nsp1 mutants. (C) Termi-Luc peptide release assay in the presence/absence of Nsp1 mutants and the release factors. Time progress curves showing luminescence (in relative luminescence units, rlu), number of repeats, n = 3, mean±SD
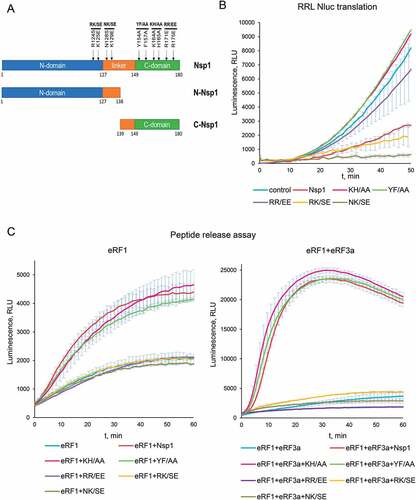
In our experiments the mutant proteins KH/AA, YF/AA, and RR/EE were unable to suppress translation in the cell-free system (), in agreement with the previous studies [Citation7,Citation13]. Moreover, the mutants KH/AA and YF/AA slightly activated cell-free translation. In contrast, the mutants RK/SE, NK/SE decreased the translation of the Nluc in the cell-free system similarly to the wild-type Nsp1 (). In peptide release assay we observed that the mutants KH/AA and YF/AA stimulated peptide release in the presence of eRF1 alone and the eRF1-eRF3a complex similarly to Nsp1 (). However, the mutants RR/EE, RK/SE and NK/SE lost the ability to stimulate peptide release ().
TC formation analysis of the same mutants showed that the proteins KH/AA and YF/AA demonstrated the same activity as wild-type Nsp1 (Fig. S7, S10). On the contrary, the mutants RR/EE, RK/SE and NK/SE did not stimulate the formation of TC (Fig. S7, S10). Therefore, TC formation analysis confirmed the results obtained by the peptide release assay.
To test the effect of Nsp1 mutants on luciferase activity per se, we added them to the peptide release reaction at the log and stationary phases of the growth curve (Fig. S3A). We demonstrated that the addition of all obtained Nsp1 mutants did not affect or affected insignificantly the activity of Nluc.
We believe that activation of the cell-free translation () and stimulation of peptide release () by the two mutant proteins KH/AA and YF/AA reflect their positive effect on translation termination in combination with the loss of the ability to inhibit initiation. On the other hand, the mutant RR/EE was inactive in all performed tests, so we concluded that this mutation reduces the whole activity of the protein, probably disrupting its structure. Indeed, the most part of expressed RR/EE protein precipitated during isolation from the lysate and further purifications, which indicates a violation of its structure.
Summarizing the obtained results, we can conclude that different parts of Nsp1 are responsible for different activities of this protein in translation. The analysis of mutant activities indicated that the C domain of Nsp1 is involved in translation repression via binding with the 40S subunit, which was shown by structural analysis [Citation5,Citation7,Citation13], but the N domain of Nsp1 is responsible for the activation of translation termination.
We also tested 3xFLAG-tagged Nsp1 (3xFLAG-Nsp1) in peptide release and TC formation analysis, as this form of Nsp1 has been used in different structural and biochemical studies [Citation7,Citation13]. Interestingly, similar to untagged Nsp1 amount of 3xFLAG-Nsp1 slightly suppressed translation of Nluc mRNA in the cell-free system (Fig. S8A). However, in the presence of either eRF1 alone, or the eRF1-eRF3a-GTP complex, 3xFLAG-Nsp1 stimulated TC formation and peptidyl-tRNA hydrolysis, like the wild-type Nsp1 (Fig. S8, S10). The effect of FLAG-Nsp1 on luciferase activity during peptide release was also tested (Fig. S3A). We showed that the addition of FLAG-Nsp1 affected insignificantly the efficiency of Nluc luminescence.
Thus, the 3xFLAG does not interfere with the functioning of Nsp1 in translation termination but decreases suppression of protein translation in the cell-free system. We did not find any information about influence of 3xFLAG-Nsp1 on translation in cell-free systems. All published data describe translation activity of 3xFLAG-Nsp1 or its mutants during overexpression in cell lines. Although 3xFLAG-Nsp1 partially suppresses translation in cell lines, overexpression of this protein in the cells could give inaccurate results due to the high amount of low activity protein. As far as we have found nobody compared activities in translation of untagged Nsp1 and 3xFLAG-Nsp1. Probably an extremely high negative charge from 3xFLAG-tag (at pH 7.5 charge is −7.17) interferes with the interaction of this protein with the ribosomal RNA. This in turn can cause an ineffective translational suppression and inefficient purification of ribosomal complexes from cell lysates via FLAG antibodies.
Individual N and C domains of Nsp1 are inactive in the initiation and termination of translation
It would be interesting for further research to determine whether it is possible to work with individual Nsp1 domains. Therefore, we decided to see if we can localize the activities in initiation and termination in separate domains of Nsp1. We obtained the N domain that contained 1–138 amino acid residues, and the C domain that contained 139–180 amino acid residues (). Then we tested the effect of the individual Nsp1 domains on the cell-free translation and peptide release. Unfortunately, both domains lost their function in suppressing NLuc cell-free translation () and did not stimulate hydrolysis of peptidyl-tRNA in the presence of eRF1 or the eRF1-eRF3a complex (). Only the N domain of Nsp1 slightly improved the peptide release in the presence of eRF1 with eRF3a (). The effect of Nsp1 domains on luciferase activity per se was also tested (Fig. S3A,B). We demonstrated that the addition of Nsp1 domains did not affect the efficiency of Nluc luminescence.
Figure 5. Activity of the Nsp1 domains in translation termination. (А) In vitro Nluc mRNA translation in RRL in the presence/absence of N or C domains of Nsp1, n = 3, mean±SD. (B) Termi-Luc peptide release assay in the presence/absence of N or C domains of Nsp1 and the release factors. Time progress curves showing luminescence (in relative luminescence units, rlu) with NLuc released from the ribosome complex upon treatment with the proteins of interest, n = 3, mean±SD
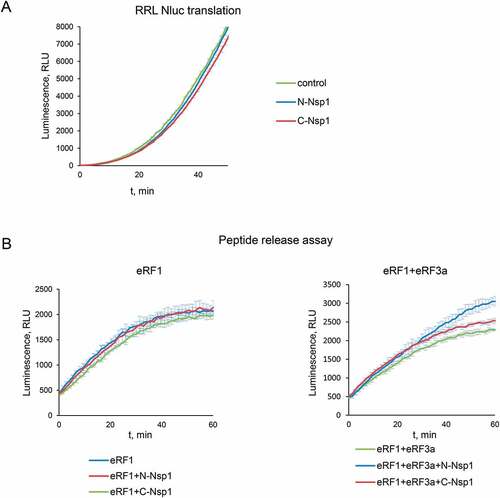
Activities of the N and the C domains of Nsp1 were also studied by the TC formation analysis. The activities of the domains clearly follow the pattern of their activities in the peptide release. The C domain of Nsp1 was unable to stimulate TC formation, and the N domain slightly stimulated TC formation in the presence of eRF1 (Fig. S9, S10). Thus, we have shown that the functioning of Nsp1, both in the translation termination and cell-free translation, requires the presence of the full-length protein.
Nsp1 stimulates translation termination at all three stop codons
As we obtained results of stimulation of peptide release and TC formation by Nsp1 only at UAA stop codon, we studied its activity at other codons. First, we determined the efficiency of TC formation on the model mRNAs with UAA, UAG and UGA stop codons (, S11). We revealed that at all three stop codons, Nsp1 equally stimulated TC formation in the presence of eRF1(AGQ) () and the eRF1(AGQ)-eRF3-GTP complex (). Second, we tested whether in the presence of Nsp1 appears a capability of eRF1 to recognize sense codons. We determined the efficiency of TC formation on the model mRNAs with UGG and GCU codons (Fig. S12, S13). We revealed that at the sense codons, Nsp1 was unable to induce TC formation in the presence of the eRF1(AGQ)-eRF3-GTP complex. Therefore, Nsp1 is equally effective in stimulating of translation termination at all three stop codons and does not work on the sense codons in the used in vitro system. This confirms the specific effect of Nsp1 on translation termination.
Figure 6. NSP1 increases TC formation at all three stop codons. (А) Toe-print analysis of the TCs formed by addition to the preTCs of eRF1 and Nsp1 and relative quantitative analysis of the TC formation efficiency at different stop codons (UAA, UAG, UGA) in the presence of eRF1 and Nsp1. TC formation efficiency induced by eRF1 alone was taken as 1. (B) Toe-print analysis of TCs formed by addition to the preTCs at different stop codons (UAA, UAG, UGA) of eRF1+ eRF3a+GTP and Nsp1 and relative quantitative analysis of the TC formation efficiency on different stop codons (UAA, UAG, UGA) by eRF1+eRF3a+GTP in the presence of Nsp1. TC formation efficiency induced by eRF1+ eRF3a+GTP was taken as 1. r.u. – relative units. Positions of preTCs and TCs are labelled by white and black triangles, respectively. Red stars indicate the increased quantity of ribosomal complexes, shifted from the preTC to the TC state. The error bars represent the standard error of mean, stars (**) mark a significant difference from the respective control P < 0.01 (n = 3)
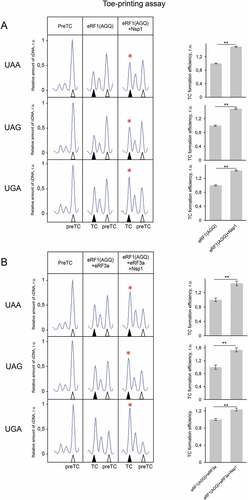
Discussion
In the present study, we show that Nsp1 promotes translation termination, which is compatible with the finding that Nsp1 binds the 80S ribosome simultaneously with eRF1 and ABCE1 [Citation13]. We show the stimulation of eRF1 stop codon recognition activity by Nsp1 during translation termination (–3) and identify the amino acid residues of Nsp1 important for its functioning in translation termination (, S7). Analysing of activities of mutated and truncated forms of these proteins, we localize the required regions for such activity – NM domain of eRF1 and N domain of Nsp1 (, 4 C). Additionally, we demonstrate that Nsp1 stimulates termination at all three stop codons ().
It should be noted that Nsp1 can not bind entry channel of the 40S ribosomal subunit, which is already bound with mRNA [Citation20]. mRNA was also absent in the structure of the 80S ribosomal complex containing Nsp1 [Citation13]. PreTCs in our experiments contained mRNA, consequently Nsp1 could not interact with the entry channel of the preTCs. Besides, it was shown that Nsp1 mutants KH/AA, YF/AA and RR/EE did not bind with the ribosome in contrast to wild-type Nsp1 [Citation7,Citation13]. In peptide release KH/AA and YF/AA mutants are able to stimulate eRF1. It confirms that Nsp1 does not require binding to the mRNA channel of the ribosome to perform its function in translation termination.
Overall, we propose the following mechanism of translation termination activation by Nsp1 (). Nsp1 interacts with the preTC together with eRF1 or with the eRF1-eRF3 complex. It promotes binding of eRF1 to the stop codon, which increases the overall efficiency of translation termination. After GTP hydrolysis and peptide release Nsp1 probably remains bound with the post-termination complexes, which were observed by Cryo-EM [Citation13]. This in turn prevents new rounds of translation initiation.
Figure 7. Model for Nsp1 activity in translation. (A) Translation termination in the presence of Nsp1. Nsp1 facilitates eRF1binding to the stop codon. After GTP hydrolysis and peptide release Nsp1 remains bound with the postTC. (B) Suppression of translation by Nsp1. Nsp1 binds to the 40S ribosomal subunits, which suppresses initiation of host translation. Additionally Nsp1 stimulates translation termination on the currently translating host mRNAs and displaces the 40S ribosomal subunits from the pool of the actively translating ribosomes. This prevents new rounds of host translation. Created with BioRender.com
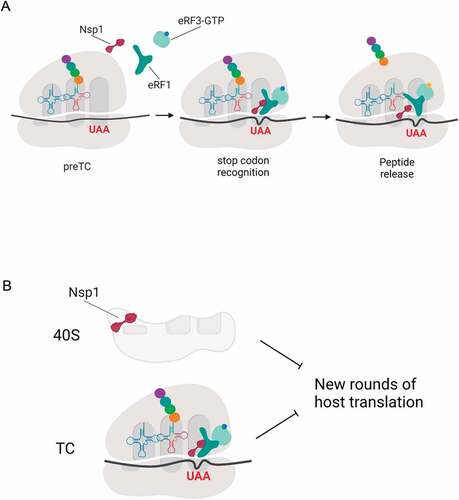
Nsp1 suppresses cell translation at the initiation stage via binding to the 40S ribosome subunit to transfer components of protein biosynthesis into the translation of virus proteins [Citation5–9,Citation13,Citation20]. But at the same time, Nsp1 probably stimulates expression of host genes via increasing translation termination rate. One can conclude that improving translation termination leads to better gene expression, unless this increased translation termination takes place at sense codons. We tested this possibility and showed that termination is stimulated by Nsp1 specifically at the stop codons at least in vitro (, S12). What are the advantages for the virus to stimulate translation termination? Ribosomes that are in this step will terminate translation and dissociate even without Nsp1, however stimulation of Nsp1 intensifies this process and accelerates cell switching to viral translation. It’s clear that the entire process of translation cannot be stopped by only suppressing initiation because those mRNAs that have already passed the initiation stage continue to be translated at the stages of elongation and termination. To disrupt the translation of such mRNAs, it would be reasonable to stimulate termination at the stop codons and remove the 40S ribosome subunits from the pool of active components via binding to the mRNA channel after the termination stage. We assume that Nsp1 is involved in this process ().
Furthermore, it should be noted that the SARS-CoV-2 mRNA contains two long ORFs for the translation of 16 proteins. They contain only two stop codons, so the elimination of the 40S subunits by Nsp1 after translation termination preferentially involves ribosomes that translate host mRNAs and do not affect the translation of the viral proteins encoded in the first two ORFs.
Material and methods
Nsp1 cloning and purification
The codon-optimized sequence of the SARS-CoV-2 Nsp1 was cloned into the petSUMO vector and expressed in E. coli BL21(DE3) cells. Noteworthy, the resulting Nsp1 lacked any tag. Nsp1 wt was amplified by PCR with Q5 polymerase (NEB) from the plasmid pDONR207 SARS-CoV-2 NSP1, which was a gift from Fritz Roth (Addgene plasmid # 141255; http://n2t.net/addgene:141255; RRID:Addgene_141255) [Citation36] using primers NSP1_F, NSP1_R. The SUMO sequence was amplified from the petSUMO plasmid (Invitrogen) by PCR with Q5 polymerase (NEB) using primers petSUMO_F, petSUMO_R. The petSUMO_Nsp1_WT construct was then obtained using PCR-products with Gibson Assembly® Master Mix (NEB), according to the manufacturer’s protocol. N (1–138 aa) and С (139–180 aa) Nsp1 domains were subcloned into petSUMO using primers NSP1_N_R and NSP1_C_F. Nsp1 mutants RK/SE, NK/SE, KH/AA, YF/AA, RR/EE were made with use of QuikChange Site-Directed Mutagenesis Kit (Agilent), according to the manufacturer’s protocol. 3xFLAG was amplified by PCR with Q5 polymerase (NEB) from our construct using primers 3xFLAG_F and 3xFLAG_R and cloned into petSUMO_NSP1_WT vector using Gibson Assembly® Master Mix (NEB). The resulting proteins were expressed in Escherichia coli BL21(DE3) cells (Invitrogen) after induction by 0.5 mM isopropyl β-D-1-thiogalactopyranoside at 18°C overnight, followed by purification using a Ni-NTA gravity flow column and elution by 20 mM Tris-HCl pH 7.5, 500 mM KCl, 7,5% glycerol, 300 mM imidazole, and 1 mM DTT. Obtained proteins were dialysed against cleavage buffer (CB), comprising 20 mM Tris-HCl (pH 7.5), 250 mM KCl, 7,5% glycerol, 1 mM DTT. Then peptide bond hydrolysis was carried out with 6xHis-tagged peptidase Ulp1 in CB. Hydrolysis was carried out during the night at +4 º. Then mix was incubated with Ni-NTA agarose, equilibrated in CB, and purified using gravity flow column. The product was dialysed in storage buffer (SB): 20 mM Tris-HCl (pH 7.5), 100 mM KCl, 10% glycerol, 1 mM DTT, frizzed in liquid nitrogen and stored at −70º. The composition of CB for Nsp1 С domain (~4,8 kDa) was identical with SB and the dialysis was not carried out.
In vitro translation assay
The linear template for Nluc was obtained by PCR with the primers: forward primer 5ʹ- CCA GTG CCA AGC TTA ATA CGA CTC ACT ATA G − 3ʹ, reverse primer 5ʹ- TTT TTT TTT TTT TTT TTT TTT TTT TTT TTT TTT TTT TTT TTT TTT TTT TTA AAC AGC TAT GAC CAT GAT T − 3ʹ. Reverse primers contained poly(T) to generate a polyadenylated 3ʹend of mRNAs. mRNAs were in vitro transcribed (T7 RiboMAX Express Large Scale RNA Production System, Promega, USA) from the Nluc PCR template.
The translation was performed in Flexi® Rabbit Reticulocyte Lysate System (Promega). Translation mixture contained 70% Flexi® RRL was supplemented with 50 mM KOAc, 1 mM Mg(OAc)2, 0.2 mM ATP, 0.2 GTP, 0.5 mM each of 20 amino acids (Promega), 2 mM DTT, 0.2 U/µl Ribolock (ThermoFisher), 1% NLuc substrate (Nano-Glo, Promega), 16 pg/µl NLuc-mRNA and 0.6 µM Nsp1 protein or its mutants/domains. Luminescence was measured at 30°C up to 1 h using a Tecan Infinite 200 Pro (Tecan, Männedorf, Switzerland). The data were presented as luminescence time progress curve with standards deviations, plotted in Microsoft Excel.
Termi-Luc peptide release assay
A peptide release with Nanoluciferase (NLuc) was performed as previously described [Citation34] with modifications. The assay allows measurement of the NLuc release from TCs assembled on NLuc mRNA in RRL. NLuc folds into the catalytically active form only after its release from the ribosome, which leads to luminescence in the presence of substrate. The NanoLuc mRNA was in vitro transcribed (T7 RiboMAX Express Large Scale RNA Production System, Promega, USA) from a template containing β-globin 5ʹ-UTR, NLuc CDS, 3ʹ-UTR derived from pNL1.1[Nluc] vector (Promega), and 50 nt poly(A) tail. 1 ml of RRL lysate (Green Hectares, USA) was preincubated in a mixture containing 1.5 u/µl Micrococcal nuclease (Fermentas) and 1 mM CaCl2 at 30°C for 10 min, followed by the addition of EGTA to a final concentration of 2 mM. A reaction mixture containing 70% nuclease-treated RRL (Green Hectares, USA) was supplemented with 20 mM Hepes-KOH (pH 7.5), 80 mM KOAc, 0.5 mM Mg(OAc)2, 0.36 mM ATP, 0.2 GTP, 0.05 mM each of 20 amino acids (Promega), 0.5 mM spermidine, 5 ng/µl total rabbit tRNAs, 10 mM creatine phosphate, 0.003 u/µl creatine kinase (Sigma), 2 mM DTT and 0.2 U/µl Ribolock (ThermoFisher) in a volume of 1 ml. The mixture was preincubated with 1 μM eRF1(AGQ) at 30°C for 10 min, followed by the addition of the NLuc mRNA to a final concentration of 8 µg/ml, resulting in the formation of TCs. Next, KOAc concentration was adjusted to 300 mM and the mixture was layered on 10–35% linear sucrose gradient in buffer containing 50 mM Hepes-KOH, pH 7.5, 7.5 mM Mg(OAc)2, 300 mM KOAc, 2 mM DTT. The gradients were centrifuged in a SW-41 Ti (Beckman Coulter) rotor at 18000 rpm for 14 h. Fractions enriched with preTCs were collected. PreTC was aliquoted, flash-frozen in liquid nitrogen, and stored at −80°C. Peptide release was performed in solution containing 8 nM preTC, 50 mM Hepes-KOH, pH 7.5, 0.25 mM spermidine, 2 mM DTT, 0.2 mM GTP, and 1% NLuc substrate (Nano-Glo, Promega) in the presence of release factors (4 nM eRF1 alone or with 4 nM eRF3a) and 80 µM Nsp1 protein or its mutants/domains or GST as negative control. Luminescence was measured up to 1 hour at 30°C using a Tecan Infinite 200Pro (Tecan, Männedorf, Switzerland). The peptide release kinetic curves or histogram with maximum values were generated and the standard deviation was calculated in Microsoft Excel.
Nanoluciferase activity analysis
Nanoluciferase luciferase activity analysis was conducted based on Termi-Luc assay with modifications. Peptide release was performed in solution containing 8 nM preTC, 50 mM Hepes-KOH, pH 7.5, 0.25 mM spermidine, 2 mM DTT, 0.2 mM GTP, and 1% NLuc substrate (Nano-Glo, Promega) in the presence of an excess of the release factors in order to achieve maximum NLuc release (300 nM eRF1 with 300 nM eRF3a) and 80 nM Nsp1 protein or its mutants/domains or GST. The measurements of NLuc luminescence were carried out using a Tecan Infinite 200Pro (Tecan, Männedorf, Switzerland) at 30°C. When an active growth or plateau of the luminescence curve was observed, the device was paused, the plate was removed and 1 µl of NSP1, NSP1 mutants, 3x-FLAG-NSP1, GST or SB buffer was added, then placed back into the device, where measurements were continued. The kinetic curves were generated and the standard deviation was calculated in Microsoft Excel.
Pre-termination complex assembly
The 40S and 60S ribosomal subunits as well as eukaryotic translation factors eIF2, eIF3, eEF1H, and eEF2 were purified from RRL or HeLa lysate, as previously described [Citation28]. The human translation factors eIF1, eIF1A, eIF4A, eIF4B, ∆eIF4G, ∆eIF5B, eIF5, PABP, and eRF1 were produced as recombinant proteins in E. coli strain BL21(DE3) with subsequent protein purification on Ni-NTA agarose and ion-exchange chromatography [Citation28]. The full-length human eRF3a was expressed in insect Sf21 cells and purified via affinity chromatography using a HisTrap HP column (GE Healthcare) followed by anion-exchange chromatography on a MonoQ column (GE Healthcare) [Citation37]. mRNAs were transcribed in vitro (T7 RiboMAX Express Large-Scale RNA Production System, Promega, USA) from the linear fragment of the pET28-MVHL-UAA plasmid containing the T7 promoter, four CAA repeats, β-globin 5ʹ-UTR, and ORF (coding for the peptide MVHL), followed by the stop codon UAA and 3ʹ-UTR, comprising the remaining natural β-globin coding sequence. Eukaryotic preTCs on MVHL-UAA mRNA were assembled and purified as previously described [Citation28,Citation35,Citation38]. Briefly, ribosomal complexes were assembled in a 500 μL solution containing 37 pmol of mRNA. They were incubated for 15 min in buffer A (20 mM Tris acetate (pH 7.5), 100 mM KAc, 2.5 mM MgCl2, 2 mM DTT) supplemented with 400 U RiboLock RNase inhibitor (Thermo Fisher Scientific, cat# EO0384), 1 mM ATP, 0.25 mM spermidine, 0.2 mM GTP, 75 μg calf liver total tRNA (acylated with all or individual amino acids; Sigma, cat# R7250), 75 pmol human 40S and 60S purified ribosomal subunits, 200 pmol eIF2, 60 pmol eIF3, 400 pmol eIF4A, 150 pmol eIF4B, and 125 pmol each of human eIF2, ΔeIF4G, eIF1, eIF1A, eIF5, and ΔeIF5B for the formation of the 80S initiation complex. After 15 min, 200 pmol of human eEF1H and 38 pmol of eEF2 were added to form the elongation complex. The ribosomal complexes were then purified via centrifugation in a Beckman SW55 rotor for 95 min at 4°C and 48,000 rpm in a 10–30% (w/w) linear sucrose density gradient (SDG) prepared in buffer A with 5 mM MgCl2. Fractions corresponding to the ribosomal complexes were diluted three-fold with buffer A containing 1.25 mM MgCl2 (to a final concentration of 2.5 mM Mg2+) and used in experiments.
Termination complex (TC) formation efficiency assay
First, 0.03 pmol preTCs incubated with release factors (0.5 pmol eRF1/eRF1(AGQ) alone or 0.1 pmol eRF1/eRF1(AGQ) with 0.1 pmol eRF3a) and optionally with 2.5 pmol Nsp1 or its mutants/domains or GST as negative control at 37°C for 15 min in the presence of 0.2 mM GTP or GDPCP, supplemented with equimolar amounts of MgCl2. Samples were analysed using the primer extension protocol. The toe-printing analysis was performed with AMV reverse transcriptase and 5ʹ-carboxyfluorescein-labelled primers complementary to 3ʹ-UTR sequences, as described previously [Citation35]. cDNAs were separated via electrophoresis using standard GeneScan® conditions on an ABI Prism® Genetic Analyser 3100 (Applera).
GTPase assay
Ribosomal 40S and 60S subunits (5 pmol each) with 5 pmol of eRF1 were associated at 37°C for 10 min. Then, other components were added. Final reaction volume was 10 μL and contained 23.5 mМ Tris-HCl pH 7.5, 35 mM NH4Cl, 18 mM MgCl2, 0.5 mM GTP, 3 pmol of eRF3a and 0, 3, 5, 10 or 15 pmol of Nsp1. After incubation for 15 min at 37°C the amount of released phosphate was estimated with Malachite Green Phosphate Assay (Sigma), according to the manufacturer’s protocol.
Quantification and statistical analysis
All experiments were carried out on at least three technical replicates (the specific number of replicates is shown in the description under figures). The data is presented as mean±standard deviation (SD), when analysing luminescence signals of lysates, or mean±standard error of mean (SE), when analysing parameters (translation). A two-tailed t-test was used to compare mean values between two groups. For multiple comparisons, the Bonferroni correction was used. One-way ANOVA was used to compare mean values between more than two groups. P-values were calculated in Microsoft Excel. The difference was considered significant when p was less than 0.05 [].
Author contributions
A.S., E.S., N.B., E.S., K.E., T.E. and E.A. designed the experiments. A.S., E.S., N.B., E.S., K.E., N.P., A.A., V.M. and T.E. performed the experiments. All authors discussed the results and contributed to the final manuscript. E.A. supervised the project. T.E. and E.A. wrote the manuscript.
Data availability
The data that support the findings of this study are contained within the article and the supporting information. All source data generated for this study are available from the corresponding author (Dr Elena Alkalaeva; [email protected]) upon reasonable request.
Supplemental Material
Download PDF (1.6 MB)Acknowledgments
We are grateful to Ludmila Frolova for providing plasmids encoding release factors, to Tatyana Pestova and Christopher Hellen for provided us with plasmids encoding initiation factors and Christiane Schaffitzel for providing the TEV-protease protein and plasmid for eRF3a baculovirus expression. pDONR207 SARS-CoV-2 NSP1 was a kind gift from Fritz Roth (Addgene plasmid # 141255; http://n2t.net/addgene:141255; RRID: Addgene_141255). Sequencing of plasmids, coding mutant proteins and cDNA fragment analyses were performed by the EIMB RAS “Genome” centre. We are thankful for the Centre for Precision Genome Editing and Genetic Technologies for Biomedicine for access to facilities necessary to conduct this study.
Disclosure statement
No potential conflict of interest was reported by the author(s).
Supplementary material
Supplemental data for this article can be accessed here
Additional information
Funding
References
- Zhu N, Zhang D, Wang W, et al. A novel coronavirus from patients with pneumonia in China, 2019. N Engl J Med. 2020;382:727–733.
- Kim D, Lee JY, Yang JS, et al. The architecture of SARS-CoV-2 transcriptome. Cell. 2020b;181:914–921.e10.
- Wu A, Peng Y, Huang B, et al. Genome composition and divergence of the novel coronavirus (2019-nCoV) originating in China. Cell Host Microbe. 2020;27:325–328.
- Zhou P, Lou YX, Wang XG, et al. A pneumonia outbreak associated with a new coronavirus of probable bat origin. Nature. 2020;579:270–273.
- Yuan S, Peng L, and Park JJ, et al. Nonstructural protein 1 of SARS-CoV-2 is a potent pathogenicity factor redirecting host protein synthesis machinery toward viral RNA. Mol Cell. 2020;80:1055–1066.e6 .
- Banerjee AK, Blanco MR, Bruce EA, et al. SARS-CoV-2 disrupts splicing, translation, and protein trafficking to suppress host defenses. Cell. 2020;183:1325–1339.e21.
- Schubert K, Karousis ED, and Jomaa A, et al. SARS-CoV-2 Nsp1 binds the ribosomal mRNA channel to inhibit translation. Nat Struct Mol Biol. 2020;27:959–966 .
- Shi M, Wang L, and Fontana P, et al. SARS-CoV-2 Nsp1 suppresses host but not viral translation through a bipartite mechanism. bioRxiv. 2020. ; doi:10.1101/2020.09.18.302901 .
- Tidu A, Janvier A, Schaeffer L, et al. The viral protein NSP1 acts as a ribosome gatekeeper for shutting down host translation and fostering SARS-CoV-2 translation. RNA. 2021;27:253–264.
- Finkel Y, Gluck A, Nachshon A, et al. SARS-CoV-2 uses a multipronged strategy to impede host protein synthesis. Nature. 2021;594:240–245.
- Rao S, Hoskins I, Tonn T, et al. Genes with 5′ terminal oligopyrimidine tracts preferentially escape global suppression of translation by the SARS-CoV-2 Nsp1 protein. RNA. 2021;27:1025–1045.
- Slobodin B, Sehrawat U, and Lev A, et al. Cap-independent translation and a precisely localized RNA sequence enable SARS-CoV-2 to control host translation and escape anti-viral response. bioRxiv.2021. https://doi.org/10.1101/2021.08.18.456855. .
- Thoms M, Buschauer R, Ameismeier M, et al. Structural basis for translational shutdown and immune evasion by the Nsp1 protein of SARS-CoV-2. Science. 2020;369:1249–1256.
- Xia H, Cao Z, Xie X, et al. Evasion of Type I Interferon by SARS-CoV-2. Cell Rep. 2020;33(1):108234.
- Zhang K, Miorin L, and Makio T, et al. Nsp1 protein of SARS-CoV-2 disrupts the mRNA export machinery to inhibit host gene expression. Sci Adv. 2021;7(6):eabe7386.
- Burke JM, St Clair LA, Perera R, et al. SARS-CoV-2 infection triggers widespread host mRNA decay leading to an mRNA export block. RNA. 2021;27:1318–1329.
- Bujanic L, Shevchuk O, and Kügelgen NV, et al. The key features of SARS-CoV-2 leader and NSP1 required for viral escape of NSP1-mediated repression. bioRxiv. 2021. doi:10.1101/2021.09.13.460054;.
- Vora SM, Fontana P, and Leger V, et al. Targeting Stem-loop 1 of the SARS-CoV-2 5ʹUTR to suppress viral translation and Nsp1 evasion. bioRxiv. 2021. doi:10.1101/2021.09.09.459641;.
- Zhu C, Lee JY, and Woo JZ, et al. An intranasal ASO therapeutic targeting SARS-CoV-2. bioRxiv. 2021. doi:10.1101/2021.05.17.444397.
- Lapointe CP, Grosely R, Johnson AG, et al. Dynamic competition between SARS-CoV-2 NSP1 and mRNA on the human ribosome inhibits translation initiation. Proc Natl Acad Sci. 2021;118:e2017715118.
- Kamitani W, Huang C, Narayanan K, et al. A two-pronged strategy to suppress host protein synthesis by SARS coronavirus Nsp1 protein. Nat Struct Mol Biol. 2009;16:1134–1140.
- Jackson RJ, Hellen CUT, and Pestova TV. Termination and post-termination events in eukaryotic translation. 2012;86: 45–93.
- Brown A, Shao S, Murray J, et al. Structural basis for stop codon recognition in eukaryotes. Nature. 2015;524:493–496.
- Frolova L, Le Goff X, Rasmussen HH, et al. A highly conserved eukaryotic protein family possessing properties of polypeptide chain release factor. Nature. 1994;372:701–703.
- Kryuchkova P, Grishin A, Eliseev B, et al. Two-step model of stop codon recognition by eukaryotic release factor eRF1. Nucleic Acids Res. 2013;41:4573–4586.
- Matheisl S, Berninghausen O, Becker T, et al. Structure of a human translation termination complex. Nucleic Acids Res. 2015;43:8615–8626.
- Song H, Mugnier P, Das AK, et al. The crystal structure of human eukaryotic release factor eRF1—mechanism of stop codon recognition and peptidyl-tRNA hydrolysis. Cell. 2000;100:311–321.
- Alkalaeva EZ, Pisarev AV, Frolova LY, et al. In vitro reconstitution of eukaryotic translation reveals cooperativity between release factors eRF1 and eRF3. Cell. 2006;125:1125–1136.
- Cheng Z, Saito K, Pisarev AV, et al. Structural insights into eRF3 and stop codon recognition by eRF1. Genes Dev. 2009;23:1106–1118.
- Frolova L, Le Goff X, Zhouravleva G, et al. Eukaryotic polypeptide chain release factor eRF3 is an eRF1- and ribosome-dependent guanosine triphosphatase. RNA. 1996;2:334–341.
- Shao S, Murray J, Brown A, et al. Decoding mammalian ribosome-mRNA states by translational GTPase complexes. Cell. 2016;167(1229–1240.e15). DOI:10.1016/j.cell.2016.10.046
- Taylor D, Unbehaun A, Li W, et al. Cryo-EM structure of the mammalian eukaryotic release factor eRF1-eRF3-associated termination complex. Proc Natl Acad Sci. 2012;109:18413–18418.
- Frolova LY, Tsivkovskii RY, Sivolobova GF, et al. Mutations in the highly conserved GGQ motif of class I polypeptide release factors abolish ability of human eRF1 to trigger peptidyl-tRNA hydrolysis. Rna. 1999;5:1014–1020.
- Susorov D, Egri S, Korostelev AA. Termi-Luc: a versatile assay to monitor full-protein release from ribosomes. RNA. 2020;26:2044–2050.
- Egorova T, Sokolova E, Shuvalova E, et al. Fluorescent toeprinting to study the dynamics of ribosomal complexes. Methods. 2019;162–163:54–59.
- Kim D-K, Knapp JJ, Kuang D, et al. A comprehensive, flexible collection of SARS-CoV-2 coding regions. G3 Genes|Genomes|Genetics. 2020a;10:3399–3402.
- Ivanov A, Mikhailova T, Eliseev B, et al. PABP enhances release factor recruitment and stop codon recognition during translation termination. Nucleic Acids Res. 2016;44:7766–7776.
- Mikhailova T, Shuvalova E, Ivanov A, et al. RNA helicase DDX19 stabilizes ribosomal elongation and termination complexes. Nucleic Acids Res. 2017;45:1307–1318.