Abstract
2,3,7,8 Tetrachlorodibenzo-p-dioxin (TCDD) suppresses adaptive immune responses and modulates the function of numerous cells involved in these responses. Our laboratory has shown that dendritic cells (DCs), which are important for the initiation of T-cell-dependent immunity, from TCDD-exposed mice exhibited reduced cell numbers and had altered expression of costimulatory molecules that are critical for the activation of T-cells. To further characterize the effects of TCDD on DCs and to elucidate a potential mechanism of toxicity, we investigated the direct effects of TCDD on DC maturation and survival in vitro. DCs, derived from bone marrow cells, were exposed to TCDD and then treated with TNFα to induced maturation. Apoptosis of bone marrow derived DCs (bmDCs) was induced by activating CD95 on the surface of the cells and was measured by annexin V staining. The TCDD-mediated changes in the expression of genes associated with apoptosis were examined using a pathway-specific c-DNA microarray. The results demonstrate that TCDD-treatment of bmDCs enhanced TNFα-induced maturation, measured as an increase in the expression of major histocompatibility complex class II, CD86, CD40, and CD54. In addition, TCDD exposure significantly augmented CD95-mediated death of bmDCs and altered the transcription of several genes involved in apoptosis. These findings confirm and extend the in vivo effects of TCDD on DC activation, and suggest that TCDD induces these changes, at least in part, via direct effects on the DC.
INTRODUCTION
Low doses of TCDD disrupt a broad spectrum of immune functions ranging from suppression of cytotoxic-T-lymphocyte activity (Kerkvliet et al., Citation1996; Clark et al., Citation1983), to reduced antibody production (Kerkvliet et al., Citation1990). In addition to these immunotoxic effects, our laboratory has recently established the dendritic cell (DC) as a target of TCDD toxicity in vivo. Exposure to TCDD significantly increased expression of costimulatory and accessory proteins on splenic DCs, followed by a significant reduction in the number of DCs found in the spleen (Shepherd et al., Citation2001; Vorderstrasse and Kerkvliet, 2001). These TCDD-induced changes in the activation of splenic DCs appeared to be biologically significant as they were associated with an enhanced proliferation of T-cells and followed by a premature termination of T-cell responses (Vorderstrasse and Kerkvliet, 2001; Vorderstrasse et al., Citation2003; Shepherd et al., Citation2000; Funatake et al., Citation2004). DCs originate from hematopoietic stem cells found in the bone marrow and differentiate in the presence of a variety of growth factors (reviewed by Banchereau et al., Citation2000). These immature DCs are proficient at taking up antigen and have a low surface expression of major histocompatibility complex class II (MHC class II) and costimulatory molecules. Maturation of DCs can be mediated by uptake of antigen, interactions with T-cells, and exposure to inflammatory cytokines (Banchereau et al., Citation2000; Winzler et al., Citation1997). DC maturation is characterized by a decrease in phagocytic activity and increased expression of proteins that aid in the presentation of antigen and costimulation of T-cells. Simultaneously, the down regulation of chemokine receptors associated with tissue retention allows mature DCs to migrate to the spleen or to the draining lymph nodes where they activate antigen-specific T-cells (Cyster, Citation1999; Sallusto and Lanzavecchia, 2000).
Previous studies have shown that TCDD increases DC activation and suppresses activation of the transcription factor NF-κB in a dendritic cell line (Ruby et al., Citation2002). NF-κB is a transcription factor involved in the maturation and survival of DCs (Oyama et al., Citation1998; Verhasselt et al., Citation1999; Rescigno et al., Citation1998; Miga et al., Citation2001). Thus, TCDD-mediated suppression of NF-κB may in fact mediate alterations in DC maturation or survival. In the present studies we sought to determine the effects of TCDD on DCs, using an in vitro system to generate large numbers of DCs from cultured bone marrow cells (Inaba et al., Citation1992). The results from this in vitro system show that TCDD-enhanced TNFα-induced maturation and increased CD95-mediated apoptosis and death. These findings demonstrate that TCDD directly targets DCs, potentially playing a role in the suppression of adaptive immune responses seen in TCDD-exposed mice.
MATERIALS AND METHODS
Reagents and Antibodies
TCDD (≥99% pure) was purchased from Cambridge Isotope Laboratories Inc. (Woburn, MA) and dimethyl sulfoxide (DMSO), was used as a vehicle for TCDD. Fetal bovine serum (FBS) was purchased from Hyclone (Logan, UT). All other reagents and cell culture supplies were purchased from Invitrogen (Carlsbad, CA). Dexamethasone and DMSO were purchased from Sigma (St. Louis, MO). Murine recombinant GM-CSF (specific activity 1.0 × 104 Uμ g), anti-CD95 (Jo-2) and anti-hamster IgG were obtained from BD Pharmingen (San Diego, CA). Murine recombinant TNFα (specific activity 1.1× 104 Uμ g) was obtained from PeproTech (London, U.K.). FITC-Annexin V was purchased from R&D Systems (Minneapolis, MN) and 7-amino-actinomycin D (7-AAD) was purchased from Calbiochem (San Diego, CA).
Generation of DCs from Bone Marrow
Cultures of bone marrow-derived DCs (bmDCs) were prepared using the basic protocol originally described by Inaba et. al. (1992). Briefly, femurs from C57Bl/6 mice were flushed with bmDC media (DMEM, 20% FBS, 100 μg/mL gentamicin and 2 mM L-glutamine). The bone marrow cells were centrifuged briefly at low speed to pellet any debris and were transferred to a fresh tube and washed. The red blood cells and dead cells were removed by gradient centrifugation using Lympholyte M (Cedar Lane, Hornby, Canada). The enriched bone marrow cells were washed twice and plated at a density of 5 × 105 cells/mL in six- or 24-well tissue culture plates (Costar, Cambridge, MA). Cells were cultured in bmDC media with 10 ng/mL GM-CSF for three days at 37ˆC in 5% CO2; cultures were then swirled to dislodge non-adherent-cells, and the media was aspirated off and replaced with fresh media. Two days later, the culture media was replaced, and on day six 10 ng/mL TNFα was added to the cultures to induce maturation. The 10 ng/mL dose of TNFα was determined following a dose response experiment, measuring expression of MHC class II and CD86 (data not shown). After a total of seven days in culture, the bmDCs were visible as clumps of loosely adherent-cells and were harvested by vigorous. The resulting bmDCs were analyzed by flow cytometry for the expression of the murine DC marker CD11c.
Cell Staining and Flow Cytometry
BmDCs were incubated in a 96-well plate on ice with saturating concentrations of mAb. Nonspecific binding of mAb was blocked by pre-incubating the cells with rat, mouse or hamster IgG (Jackson ImmunoResearch, West Grove, PA). All cell preparations were stained with antibodies to CD11c for the selective analysis of DC. In various experiments, bmDC were stained with mAb to MHC class II (IAb), CD86, CD40, CD54, LFA-1, and CD95 (BD Pharmingen, San Diego, CA). Appropriately labeled isotype controls were used to determine nonspecific fluorescence. Listmode data were collected on a Coulter Epics XL flow cytometer and analyzed using WinList software (Verity Software House, Inc., Topsham, ME). Cell viability was determined by propidium iodide exclusion. For phenotypic evaluation, 20,000 viable cells were analyzed.
Gene Array
BmDC cultures were treated on day 6 with 10 ng/mL of TNFα and incubated for 24 hours. The media was then replaced with fresh media containing 5% FBS and 10−8 M TCDD or vehicle control. After another 24-hour incubation, cells were harvested and stained using a biotin-conjugated anti-CD11c antibody (BD Pharmingen, San Diego, CA) followed by streptavidin-spectral red (Southern Biotechnology Associates, Birmingham, AL). CD11c positive cells were sorted on a high-speed cell sorter (MoFlo, Cytomation, Ft. Collins, CO) to a purity of 94%. Total RNA was isolated from the sorted cells using an RNeasy Mini Kit (Qiagen, Valencia, CA) and stored at −80ˆC. RNA was quantitated by measuring the absorbance at 260 nm. The purity of the RNA was assessed by both the 260/280 absorbance ratio (> 1.8) and by 1.5% agarose gel with 0.5 μ g/mL of ethidium bromide. The Q series mouse apoptosis gene array from Superarray, Inc. (Bethesda, MD) was used to analyze the differential expression of 96 genes associated with signaling for apoptosis. Preparation of labeled samples and hybridization were performed following the manufacturer's instructions. The arrays were analyzed using Personal Densitometer and ImageQuant software from Molecular Dynamics (Amersham Biosciences, Sunnyvale, CA). All genes were normalized to the housekeeping gene, cyclophilin A. Of the four housekeeping genes on the array (GAPDH, cyclophilin A, RPL13A, and β-actin), cyclophilin A was chosen because the individual spot densities were distinct and similar in intensity on each array. A value of 1.0% of the cyclophilin A gene was used to identify those genes that were expressed (> 1.0%) or not expressed < 1.0%).
Determination of Apoptosis and Death of BmDCs
BmDCs were cultured as previously described and treated on day 6 with 10 ng/mL TNFα. One day later the media was replaced with media containing a reduced amount of serum (5%) and no GM-CSF or TNFα. TCDD or DMSO, as vehicle control, was then added to the cultures and 24 hours later the cells were treated with anti-CD95 antibody, to induce apoptosis, or a hamster IgG control. One day later, bmDCs were harvested on ice and stained with mAb to CD11c as described above. The staining media was replaced with annexin V binding buffer (0.1 M HEPES, M NaCl, 2.5 mM CaCl2), and the cells were then stained with 0.1 μg FITC-conjugated annexin V and 2 μg 7-AAD. Cells were incubated for 10 minutes at room temperature in the dark. Samples (20,000 cells) were immediately analyzed by flow cytometry.
Staining of Nuclei for Apoptotic Phenotype
BmDCs (5 × 104 cells) were deposited onto slides using a Cytospin centrifuge (Shandon Southern Products, Cheshire, UK). After drying, slides were fixed in 3.7% formaldehyde and then stained with DAPI (Sigma, St. Louis, MO) according to Shin et al. (Citation2000). Slides were analyzed by fluorescence microscopy for nuclear condensation.
Statistical Analysis
For all the experiments, a Student's t test was used to compare means of vehicle-treated groups to TCDD-exposed groups. For analysis, values of p ≤ 0.05 were considered significant and expressed as follows: *p ≤ 0.05 and ** p ≤ 0.01. Experimental replication is indicated in figure and table legends.
RESULTS
TCDD Alters the Expression of Costimulatory and Accessory Molecules on TNFα Treated BmDCs
TNFα treatment induces the up-regulation of MHC class II and a number of important costimulatory molecules on the surface of the DC (Winzler et al., Citation1997; Zhang et al., Citation1997). We investigated the effects of TCDD exposure on TNFα-mediated up-regulation of MHC class II, CD86, CD40, CD54, and LFA-1 on CD11c positive (CD11c+) bmDCs. Untreated CD11c+ bmDCs expressed low or constitutive levels of CD40, CD54, and LFA-1 (data not shown). In contrast, expression of MHC class II and CD86 appeared to consist of two separate populations expressing high and low levels of these molecules (, solid gray lines). Following a 24-hour treatment with TNFα, an increased percentage of CD11c+ bmDCs expressed high levels of MHC class II and CD86 (, gated regions). As shown in , addition of 10−9 M and 10−8 M TCDD to bmDC cultures one day prior to TNFα treatment further increased the percentage of CD11c+ bmDCs expressing high levels of MHC class II and CD86. However, the level of expression on a per cell basis, as measured by median channel fluorescence (MCF) was not altered by treatment with TCDD. Moreover, TCDD did not alter the total number of CD11c+ bmDC, indicating that the increased percentage of bmDCs expressing these two molecules was a consequence of enhanced maturation. To confirm that maturation of these bmDCs could be suppressed, dexamethasone, a known suppressive agent (Matyszak et al., Citation2000; Piemonti et al., Citation1999), was added to bmDC cultures prior to TNFα treatment. As expected, dexamethasone effectively decreased the percent of bmDCs expressing high levels of CD86 when compared to untreated controls (data not shown).
Figure 1 Effect of TNFα on the expression of MHC class II and CD86 on bmDCs. Day 6 bmDC cultures were treated with 10 ng/mL TNFα and harvested following 24 hour incubation. BmDC were analyzed by flow cytometry. Representative histograms depict MHC class II and CD86 expression on CD11c+ cells. Vehicle (solid grey line), TNFα (solid black line), isotype control (dotted line). Regions were set based on high levels of MHC class II and CD86 staining.
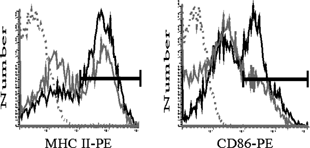
TABLE 1 Percent and expression of MHC IIhigh and CD86high on TNFα -treated bone marrow-derived DCsFootnotea
Expression of the costimulatory and accessory molecules CD40, CD54, and LFA-1 on TNFα-treated bmDCs was also altered by TCDD exposure (). TCDD increased the percentage of TNFα-treated CD11c+ bmDCs that expressed CD40, a costimulatory molecule found predominantly on mature DCs that mediates survival signals and is critical in priming DCs (Banchereau et al., Citation2000) (). Additionally, TCDD-exposure increased the expression level of the constitutively expressed adhesion molecule CD54, when measured as MCF (). In contrast to elevated expression of MHC class II, CD86, CD40, and CD54, the percentage of bmDCs expressing LFA-1, an adhesion molecule, was significantly reduced following TCDD treatment compared to vehicle controls (). Interestingly, this same selective reduction in expression of LFA-1 on DCs was seen in TCDD-exposed mice (Vorderstrasse and Kerkvliet, 2001).
Figure 2 Effect of TCDD on the expression of CD40, CD54, and LFA-1 on TNFα-treated bmDCs. Day 5 bmDC cultures were exposed to TCDD (10− 9 M and 10− 8 M) or vehicle control (0.01% DMSO) and on day 6 treated with 10 ng/mL TNFα. After seven days total in culture, bmDC were harvested and analyzed by flow cytometry. Representative histograms depict CD40, CD54, and LFA-1 expression on CD11c+ cells. Vehicle (solid grey line), 10− 8 M TCDD (solid black line), isotype control (dotted line). Results are representative of three independent experiments.
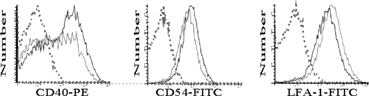
TABLE 2 Percent and expression of CD40, CD54 and LFA-1 on TNFα -treated bone marrow-derived DCsFootnotea
TCDD Exposure Increases Anti-CD95-Mediated Apoptosis and Death of TNFα-Treated bmDCs
Next we investigated the potential pro-apoptotic effects of TCDD on cultured bmDCs. Previous findings from our laboratory and others have suggested that TCDD influences the survival of a number of immune cells from thymocytes to DCs (Laiosa et al., Citation2003; Vorderstrasse and Kerkvliet, 2001). As previously noted, the study by Vorderstrasse and colleagues demonstrated a significant loss of splenic DCs in TCDD-treated mice, however this study was unable to determine if the loss of DCs was due to cell death or efflux from the spleen. In the current studies, the effect of TCDD on bmDC survival was assessed by binding of annexin V to phosphatidyl serine residues on apoptotic cells and uptake of the vital stain 7-AAD in dead cells. To resolve the phenotypic difference between apoptosis and dead cells in our model, bmDCs were treated with H2O2 to induce apoptosis (Gardner et al., Citation1997) or exposed to heat (65ˆC) to produce necrotic death. Based on the differential expression of annexin V and 7-AAD in H2O2 and heat treated bmDCs, apoptotic and dead populations were defined ().
Figure 3 Effect of TCDD on anti-CD95-mediated apoptosis and death of TNFα -treated bmDCs. (A) BmDC cultures were treated with 0.1 mM H2O2 for six hours to induce apoptosis, or heated for two minutes at 65ˆC to induce necrosis to define apoptosis and death. BmDCs were harvested, stained with annexin V, 7-AAD, and antibodies to CD11c to identify bmDCs, and analyzed by flow cytometry. Regions were established from these controls to identify apoptotic cells “A” and dead cells “D.” (B) Day 6 bmDC cultures were treated with 10 ng/mL TNFα. On day 7 media was replaced with reduced serum (5%) and without GM-CSF and cultures exposed to TCDD (10− 8 or 10−9 M) or vehicle control (0.01% DMSO) for one day. Anti-CD95 (1 μ g/mL) or hamster IgG, as a control, was added and then cultures were treated with 2 μ g/mL crosslinking antibody. After a total of nine days in culture bmDCs were harvested and stained with annexin V, 7-AAD, and antibodies to CD11c to identify bmDCs. BmDCs were analyzed by flow cytometry, and apoptotic and dead DC were defined by regions set previously using necrosis and H2O2 controls. (C) Graphical representation of the effect of TCDD on anti-CD95-mediated deletion (apoptosis plus death) of TNFα -treated DC. Labeled as vehicle (white bars), 10− 9 M TCDD (grey bars), and 10− 8 M TCDD (dark grey bars). See Materials and Methods for statistical analysis. Results are representative of three independent experiments.
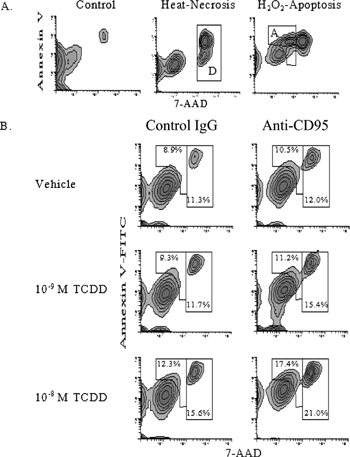
TNFα-matured bmDCs were treated with TCDD or vehicle, followed by treatment with anti-CD95 (Jo-2), an agonistic antibody known to induce apoptosis in lymphocytes and DCs (Ogasawara et al., Citation1995; Nagata et al., Citation1995; Nagata, Citation1999; Matsue et al., Citation1999). BmDCs were analyzed for apoptosis and death 24 hours after treatment with anti-CD95 antibody, as preliminary time course experiments established the 24-hour time point as optimal for measuring apoptosis (data not shown). In addition, an increase in nuclear condensation, a microscopic indicator of apoptosis, was also observed in bmDCs following treatment with anti-CD95 antibody validating our annexin V staining results (data not shown). As shown in , TCDD, in the presence of anti-CD95 antibody, significantly increased the percentage of bmDCs that were annexin V+ (region A, apoptotic) and increased the percentage of bmDCs that stained with high levels of 7-AAD (region D, dead), when compared to vehicle controls. As seen in when both apoptotic and dead phenotypes were combined, TCDD caused a concentration-dependent increase in bmDC deletion. It should be noted that in the absences of anti-CD95 antibody TCDD at the 10−8 M induced a small, but significant increase in both in apoptosis and death, when compared to vehicle control. However these increases were not statistically significant in all experiments.
TCDD Increases the Expression of Several Genes Associated with Apoptosis
TNFα-treated bmDCs were exposed to TCDD and the expression of pro- and anti-apoptotic genes were analyzed by gene array. Sorted CD11c+ bmDCs pretreated with TNFα and exposed to TCDD or vehicle control (n = 2) were lysed and mRNA was hybridized to a gene array. Of the 96 genes analyzed, only 14 genes displayed consistent changes following TCDD exposure. The remaining 82 genes were either not detected in either vehicle- or TCDD-treated samples, or expressed in all samples but were not changed following exposure to TCDD, or were inconsistently expressed between the two samples. As shown in A, 11 genes were expressed only in the TCDD-treated bmDC. Three other genes, caspase 2, Dffa, and Hus 1, were detected in at least one vehicle-treated sample and the expression of these genes was increased at least 2-fold in both of the TCDD-treated samples (B). Interestingly, the TCDD-mediated increase reflected in CD95 transcription was not reflected CD95 surface expression, which was unaltered by TCDD treatment (). Thus, it appears that changes in CD95 mRNA cannot explain the sensitivity of TCDD-exposed bmDCs to anti-CD95-mediated apoptosis, suggesting that TCDD may be enhancing apoptosis by altering the signal transduction pathways involved in apoptosis.
Figure 4 Effect of TCDD on the expression of CD95 on TNFα -treated bmDCs. Day 6 bmDC cultures were treated with 10 ng/mL TNFα and exposed to TCDD (10− 8 M) or vehicle control (0.01% DMSO). After seven days total in culture, bmDCs were harvested and CD95 expression analyzed by flow cytometry. Representative histograms depict CD95 expression on CD11c+ cells. Vehicle (Solid grey line), 10− 8 M TCDD (solid black line), and isotype control (dotted line). Results are representative of two independent experiments.
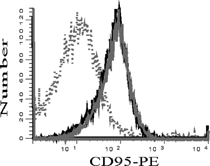
TABLE 3 TCDD exposure increases the expression of several genes associated with apoptosisFootnotea
DISCUSSION
In this study, the direct effects of TCDD on the maturation and survival of DCs were examined. The results of this study demonstrate that TCDD directly enhances TNFα-mediated DC maturation and decreases DC survival in response to CD95 activation. The direct effects produced by TCDD on bmDCs are consistent with the findings of previous studies wherein treatment of mice with TCDD resulted in an activation-like phenotype of splenic DCs and a subsequent decrease in their numbers compared to vehicle-treated mice (Vorderstrasse and Kerkvliet, 2001). Interestingly, the ability of TCDD to activate DCs in vivo occurred in the absence of any intentional exogenous stimulation, whereas the effects of TCDD on DCs in vitro occurred only in the presence of TNFα. It is possible that exposure to TCDD in vivo initially enhanced TNFα production, which then facilitated the effects of TCDD on DCs. Prior studies have shown that TCDD enhances TNFα production under a variety of exposure conditions (Kern et al., Citation2002; Moos et al., Citation1997; Rier et al., Citation2001). This interpretation could explain the reported absence of TCDD effects on human monocyte-derived DCs, since no maturation stimulus such as TNFα was used (Laupeze et al., 2002). Taken together, these results suggest that TCDD may selectively act on DCs during the process of maturation.
Although the ability of TCDD to enhance the maturation of DCs appears contradictory to the immunosuppressive activities associated with TCDD, the increased maturation of DCs could, in fact, compromise their function. Indeed, mature DCs die in the absence of survival signals that are usually provided by activated T-cells (De Smedt et al., Citation1998; Winzler et al., Citation1997). If TCDD alone activates DCs in the absence of antigen, then survival signals would be lacking and the DC would die by neglect. This could contribute to a decline in DC numbers and reduce immune responsiveness. Alternatively, or in addition, TCDD may actively induce the death of DCs by promoting death-inducing signaling processes such as apoptosis. Our results support this theory as TCDD exposure appeared to sensitize TNFα-matured DCs to CD95-mediated apoptosis and death, and altered the transcription of several genes involved in this pathway.
In an analysis of the expression profile of 96 genes involved in the regulation of apoptosis, we observed a TCDD-mediated increase in the transcription of 14 genes including CD95, yet surface expression of CD95 was not altered by TCDD. Of the remaining 14 genes increased by TCDD, several are involved in CD95-mediated cell death. For example, an increase in transcription of the pro-apoptotic protein FADD (Tibbetts et al., Citation2003; Zhang et al., Citation1998) could facilitate the formation of death-inducing signaling complexes, and lead to increased cleavage of pro-caspase 8. Activated caspase 8 initiates a cascade of enzymatic cleavages of effector caspases (Nicholson et al., 1997). Interestingly, two effector caspases, caspases 9 and 2, were up-regulated by TCDD. Further experiments will be undertaken to fully ascertain the effect of TCDD on CD95 signaling in DCs and other apoptotic pathways. In addition to the potential increases in CD95 signaling, TCDD has been previously been shown to suppress NF-κB transcription factor signaling in DCs (Ruby et al., Citation2002). NF-κB is an important redox sensitive transcription factor that is important in survival (Wang et al., Citation1998). Thus, the combination of these two TCDD-mediated events, increased transcription of CD95 signaling proteins and decreased NF-κB signaling, may help to explain the sensitivity of TCDD exposed DCs to CD95-mediated apoptosis in the absences of increased CD95 expression.
In conclusion, the present data establish the DC as a direct target of TCDD-mediated toxicity. TCDD-induced DC toxicity includes enhanced maturation and increased apoptosis. The increase in apoptosis and death of TCDD-exposed DCs also represents a mechanism that could initiate or exacerbate TCDD-induced immune suppression. DC survival is crucial in the maintenance and strength of T-cell-mediated immune responses (Josien et al., Citation2000; Miga et al., Citation2001). Moreover, recent data have determined that the duration of the interaction between DCs and T-cells is critical for T-cell “fitness,” characterized by survival and cytokine responsiveness (Gett et al., Citation2003; van Stipdonk et al., Citation2003). Gett and colleagues demonstrated that T-cells receiving a short stimulation were capable of proliferating, but failed to respond to survival signals and died by neglect. Hence, a TCDD-mediated increase in apoptosis and death of DCs, as presented here, could diminish the duration of DC-T-cell interaction thereby reducing T-cell survival and leading to premature termination of immune responses, both hallmarks of TCDD-toxicity.
ACKNOWLEDGMENTS
We would like to thank Julie Oughton for assistance with flow cytometric analysis and data interpretation; Linda Steppan for technical assistance and the Environmental Health Sciences Center for the use of the flow cytometer. This work was supported by EHS Center Grant ES00210 and Program Project Grant ES00040.
REFERENCES
- Banchereau J., Briere F., Caux C., Davoust J., Lebecque S., Liu Y. J., Pulendran B., Palucka K. Immunobiology of dendritic cells. Annual Review of Immunology 2000; 18: 767–811
- Clark D. A., Sweeney G., Safe S., Hancock E., Kilburn D. G., Gauldie J. Cellular and genetic basis for suppression of cytotoxic T-cell generation by haloaromatic hydrocarbons. Immunopharmacology 1983; 6: 143–153
- Cyster J. G. Chemokines and the homing of dendritic cells to the T-cell areas of lymphoid organs. Journal of Experimental Medicine 1999; 189: 447–450
- De Smedt T., Pajak B., Klaus G. G. B., Noelle R. J., Urbain J., Leo O., Moser M. Antigen-specific T lymphocytes regulate lipopolysaccharide-induced apoptosis of dendritic cells in vivo. Journal of Immunology 1998; 161: 4476–4479
- Funatake C. J., Dearstyne E. A., Steppan L. B., Shepherd D. M., Spanjaard E. S., Marchak-Rothstein A., Kerkvliet N. I. Early consequences of 2,3,7,8-Tetrachlorodibenzo-p-dioxin exposure on the activation and survival of antigen-specific T-cells. Toxicol. Sci. 2004; 82: 129–142
- Gardner A. M., Xu F. H., Fady C., Jacoby F. J., Duffey D. C., Tu Y., Lichtenstein A. Apoptotic vs. nonapoptotic cytotoxicity induced by hydrogen peroxide. Free Radical Biology and Medicine 1997; 22: 73–83
- Gett A. V., Sallusto F., Lanzavecchia A., Geginat J. T-Cell Fitness Determined by Signal Strength. Nature Immunology 2003; 4: 355–360
- Inaba K., Inaba M., Romani N., Aya H., Deguchi M., Ikehara S., Muramatsu S., Steinman R. M. Generation of large numbers of dendritic cells from mouse bone marrow cultures supplemented with granulocyte/macrophage colony-stimulating factor. Journal of Experimental Medicine 1992; 176: 1693–1702
- Josien B. R., Li H. L., Ingulli E., Sarma S., Wong R., Vologodskaia M., Steinman R. M., Choi Y. TRANCE, a tumor necrosis factor family member, enhances the longevity and adjuvant properties of dendritic cells In vivo. Journal of Experimental Medicine 2000; 191: 495–502
- Kerkvliet N. I., Baecher-Steppan L., Shepherd D. M., Oughton J. A., Vorderstrasse B. A., Dekrey G. K. Inhibition of TC-1 cytokine production, effector cytotoxic T lymphocyte development and alloantibody production by 2,3,7,8-tetrachlorodibenzo-p-dioxin. Journal of Immunology 1996; 157: 2310–2319
- Kerkvliet N. I., Steppan L. B., Brauner J. A., Deyo J. A., Henderson M. C., Tomar R. S., Buhler D. R. Influence of the Ah locus on the humoral immunotoxicity of 2,3,7, 8-tetrachlorodibenzo-p-dioxin: Evidence for Ah-receptor-dependent and Ah-receptor-independent mechanisms of immunosuppression. Toxicology and Applied Pharmacology 1990; 105: 26–36
- Kern P. A., Dicker-Brown A., Said S. T., Kennedy R., Fonseca V. A. The stimulation of tumor necrosis factor and inhibition of glucose transport and lipoprotein lipase in adipose cells by 2,3,7,8-tetrachlorodibenzo-p-dioxin. Metabolism 2002; 51: 65–68
- Laiosa M. D., Wyman A., Murante F. G., Fiore N. C., Staples J. E., Gasiewicz T. A., Silverstone A. E. Cell proliferation arrest within intrathymic lymphocyte progenitor cells causes thymic atrophy mediated by the aryl hydrocarbon receptor. Journal of Immunology 2003; 171: 4582–4591
- Laupeze B., Amiot L., Sparfel L., Le Ferrec E., Fauchet R., Fardel O. Polycyclic aromatic hydrocarbons affect functional differentiation and maturation of human monocyte-derived dendritic cells. Journal of Immunology, 168: 2652–2658
- Matsue H., Matsue K., Walters M., Okumura K., Yagita H., Takashima A. Induction of antigen-specific immunosuppression by CD95L cDNA-transfected ‘killer’ dendritic cells. Nature Med. 1999; 5: 930–937
- Matyszak M. K., Citterio S., Rescigno M., Ricciardi-Castagnoli P. Differential effects of corticosteroids during different stages of dendritic cell maturation. European Journal of Immunology 2000; 30: 1233–1242
- Miga A. J., Masters S. R., Durell B. G., Gonzalez M., Jenkins M. K., Maliszewski C. R., Kikutani H., Wade W. F., Noelle R. J. Dendritic cell longevity and T-cell persistence is controlled by CD154-CD40 interactions. European Journal of Immunology 2001; 31: 959–965
- Moos A. B., Oughton J. A., Kerkvliet N. I. The effects of 2,3,7,8-tetrachlorodibenzo-p-dioxin (TCDD) on tumor necrosis factor (TNF) production by peritoneal cells. Toxicology Letters 1997; 90: 145–153
- Nagata S. Fas ligand-induced apoptosis. Annual Review of Genetics 1999; 33: 29–55
- Nagata S., Golstein P. The Fas death factor. Science 1995; 267: 1449–1456
- Nicholson D. W., Thornberry N. A. Caspases: Killer proteases. Trends in Biolchemical Science 1997; 22: 299–306
- Ogasawara J., Suda T., Nagata S. Selective apoptosis of CD4+CD8+ thymocytes by the anti-Fas antibody. Journal of Experimental Medicine 1995; 181: 485–491
- Oyama T., Ran S, Ishida T., Nadaf S., Kerr L., Carbone D. P., Gabrilovich D I. Vascular endothelial growth factor affects dendritic cell maturation through the inhibition of nuclear factor-kappa B activation in hemopoietic progenitor cells. Journal of Immunology 1998; 160: 1224–1232
- Piemonti L., Monti P., Allavena P., Sironi M., Soldini L., Leone B. E., Socci C., Di C. V. Glucocorticoids affect human dendritic cell differentiation and maturation. Journal of Immunology 1999; 162: 6473–6481
- Rescigno M., Martino M., Sutherland C. L., Gold M. R., Ricciardi-Castagnoli P. Dendritic cell survival and maturation are regulated by different signalling pathways. Journal of Experimental Medicine 1998; 188: 2175–2180
- Rier S. E., Coe C. L., Lemieux A. M., Martin D. C., Morris R., Lucier G. W., Clark G. C. Increased tumor necrosis factor-α production in peripheral blood leukocytes from TCDD-exposed rhesus monkeys. Toxicolgical Sciences 2001; 60: 327–337
- Ruby C. E., Leid M., Kerkvliet N. I. 2,3,7,8-tetrachlorodibenzo-p-dioxin suppresses tumor necrosis factor-α and anti-CD40-induced activation of NF-κ B/Rel in dendritic cells: p50 homodimer activation is not affected. Molecular Pharmacology 2002; 62: 722–728
- Sallusto F., Lanzavecchia A. Understanding dendritic cell and T-lymphocyte traffic through the analysis of chemokine receptor expression. Immunological Reviews 2000; 177: 134–140
- Shepherd D. M., Dearstyne E. A., Kerkvliet N. I. The effects of TCDD on the activation of ovalbumin (OVA)-specific DO11.10 transgenic CD4(+) T-cells in adoptively transferred mice. Toxicol. Sci. 2000; 56: 340–350
- Shepherd D. M., Steppan L. B., Hedstrom O. L., Kerkvliet N. I. Anti-CD40 treatment of 2,3,7,8-tetrachlorodibenzo-p-dioxin (TCDD)-exposed C57Bl/6 mice induces activation of antigen presenting cells yet fails to overcome TCDD-induced suppression of allograft immunity. Toxicol. Appl. Pharmacol. 2001; 170: 10–22
- Shin K. J., Bae S. S., Hwang Y. A., Seo J. K., Ryu S. H., Suh P. G. 2,2′,4,6,6′-Pentachlorobiphenyl induces apoptosis in human monocytic cells. Toxicology and Applied Pharmacology 2000; 169: 1–7
- Tibbetts M. D., Zheng L., Lenardo M. J. The death effector domain protein family: Regulators of cellular homeostasis. Nature Immunology 2003; 4: 404–409
- van Stipdonk M. J. B., Hardenberg G., Bijker M. S., Lemmens E. E., Droin N. M., Green D. R., Schoenberger S. P. Dynamic programming of CD8+ T lymphocyte responses. Nature Immunology 2003; 4: 361–365
- Verhasselt V., Vanden Berghe W., Vanderheyde N., Willems F., Haegeman G., Goldman M. N-acetyl-L-cysteine inhibits primary human T-cell responses at the dendritic cell level: Association with NF-kappaB inhibition. Journal of Immunology 1999; 162: 2569–2574
- Vorderstrasse B. A., Dearstyne E. D., Kerkvliet N. I. Influence of 2,3,7,8-tetrachlorodibenzo-p-dioxin (TCDD) on the antigen-presenting activity of dendritic cells. Toxicology 2003; 72: 15–28
- Vorderstrasse B. A., Kerkvliet N. I. 2,3,7,8-tetrachlorodibenzo-p-dioxin affects the number and function of murine splenic dendritic cells and their expression of accessory molecules. Toxicol. Appl. Pharmacol. 2001; 171: 117–125
- Wang C. Y., Mayo M. W., Korneluk R. G., Goeddel D. V., Baldwin A. S., Jr. NF-κ B antiapoptosis: Induction of TRAF1 and TRAF2 and c-IAP1 and c-IAP2 to suppress caspase-8 activation. Science 1998; 281: 1680–1683
- Winzler C., Rovere P., Rescigno M., Granucci F., Penna G., Adorini L., Zimmerman V. S., Davoust J., Ricciardi-Castagnoli P. Maturation stages of mouse dendritic cells in growth factor-dependent long-term cultures. Journal of Experimental Medicine 1997; 185: 317–328
- Zhang J., Cado D., Chen A., Kabra M. H., Winoto A. Fas-mediated apoptosis and activation-induced T-cell proliferation are defective in mice lacking FADD/Mort1. Nature 1998; 392: 296–300
- Zhang Y., Mukaida N., Wang J., Harada A., Akiyama M., Matsushima K. Induction of dendritic cell differentiation by granulocyte-macrophage colony-stimulating factor, stem cell factor, and tumor necrosis factor alpha in vitro from lineage phenotypes-negative c-kit+ murine hematopoietic progenitor cells. Blood 1997; 90: 4842–4853