Abstract
Understanding the effects of nanoparticles (NP) on immune cell functions is essential in designing safe and effective NP-based in vivo drug delivery systems. The immunomodulatory potential of gold nanoparticles (GNP) and silver nanoparticles (SNP) was investigated in vitro using murine splenic and human peripheral blood lymphocytes (PBL) in terms of effects on viability and mitogen-induced proliferation. Hydrodynamic size and number of NP were characterized using NP tracking analysis (NTA); modal diameters of GNP and SNP were 28 (±1.5) and 66 (± 2.7) nm, respectively, with a unimodal distribution. Lymphocytes were incubated with GNP or SNP in the presence/absence of B- or T-cell mitogens and proliferative responses then determined using [3H]-thymidine incorporation. Concanavalin A (T-cell-specific) and lipopolysaccharide- (B-cell-specific) stimulated responses of murine splenic lymphocytes, as well as phytohemagglutinin (T-cell-specific) and pokeweed mitogen- (B-and T-cell specific) induced responses of human lymphocytes, were significantly inhibited by GNP (25–200 μg/ml) and SNP (12.5–50 μg/ml). However, [3H]-thymidine incorporation by unstimulated lymphocytes was unaffected in the presence of GNP or SNP. Viability of lymphocytes was determined using trypan blue dye exclusion and was significantly inhibited only at 200 μg GNP/ml and 25 or 50 μg SNP/ml. As mitogen responses are most useful to provide supportive mechanistic information on primary immunotoxicologic functional observations, and so far more comprehensive data (in vivo and in vitro) is still needed, the results nevertheless suggest to us that GNP and SNP might potentially be able to modulate immune responses by impacting on lymphocyte activation.
Introduction
Nanoparticles (NP) have unique characteristics such as a small size, large surface area per unit mass, surface charge and an ability to exist in different stable geometric forms. These properties have been exploited for industrial and biomedical applications (Corot et al. Citation2006). Use of NP in consumer products has also been steadily growing; this has led to rising concern regarding their overall biosafety (Mironava et al. Citation2010; Vance et al. Citation2015).
Gold nanoparticles (GNP) have been used in many areas of medicine that include diagnostics, imaging, biosensing, drug delivery and cancer therapy. An amenability to functionalization with bioactive ligands, internalization by cells, a presence of a high electron density, size-dependent optical properties, thermal stability, etc. are some of the important features of GNP that have been exploited in biomedical applications (Mironava et al. Citation2010; Tomic et al. Citation2014). Silver nanoparticles (SNP) have been predominantly used in a variety of consumer and medical products primarily due to their apparent anti-bacterial properties (Rai et al. Citation2009; Vance et al. Citation2015; Vergallo et al. Citation2014).
Although there are potential beneficial uses of GNP and SNP, there are also untoward effects from exposure to these agents. Effects of GNP on dendritic cells and malignant B- and T-cells has been reported (Bhattacharya et al. Citation2007; Mukherjee et al. Citation2007; Villiers et al. Citation2010). Interestingly, GNP selectively inhibited the proliferation of myeloma cells, but had no effect on the proliferation of human peripheral blood lymphocytes (PBL) (Bhattacharya et al. Citation2007). Similarly, exposure of experimental animals to SNP has been shown to cause anemia, cardiac enlargement, growth retardation and degenerative changes in the liver (Butler et al. Citation2015). With regard to the immune system, the viability of human PBL was decreased in time- and dose-dependent manners upon exposure to SNP in vitro (Ghosh et al. Citation2012; Vergallo et al. Citation2014; Zhornik et al. Citation2014).
To better understand the adverse effects of NP in general, information on the response of different cell types—including immune cells—in terms of NP concentration, size, shape, surface composition and time is needed (Liu et al. Citation2013). Studies of the effects of NP on immune cells are generally scant (Becker et al. Citation2014; Hussain et al. Citation2012). Hence, there remains a pressing need to evaluate effects of various types of NP on immune cell function (Dobrovolskaia & McNeil Citation2007; Dobrovolskaia et al. Citation2008; Elsabahy & Wooley Citation2013; Zolnik et al. Citation2010). Accordingly, the present study was designed to evaluate effects of GNP and SNP on lymphocyte activation by mitogens in vitro to begin to provide some information (note: mitogen responses are most useful to provide supportive mechanistic information on primary immunotoxicologic functional observations) to better define potential immunotoxicities of these types of particles.
Materials and methods
Chemicals
RPMI 1640, penicillin G, streptomycin, pokeweed mitogen (PWM), phytohemagglutinin (PHA), concanavalin A (ConA), lipopolysaccharide (LPS, type 055:B5 from Escherichia coli), heparin and histopaque (d = 1.077 g/ml) were procured from Sigma (Hyderabad, India). [3H]-Thymidine (specific activity =6.5 Ci/mmol) was purchased from BRIT (Mumbai, India). Fetal calf serum (FCS) was obtained from Biological Industries (Kibbutz Beit-Haemek, Israel). All other chemicals used were obtained from SRL Chemicals (Mumbai) and were analytical grade.
Preparation and characterization of GNP and SNP
Both GNP and SNP were synthesized using a chemical reduction method with trisodium citrate (Lee & Meisel Citation1982; Basu et al. Citation2007). GNP and SNP formed in the suspension were sedimented by centrifugation (11 000g, 20 min) to remove excess citrate. Each NP pellet was then suspended in ultrapure water and stored at 4 °C. Yields of each NP were calculated gravimetrically (typical yields of GNP and SNP were 73 and 60%, respectively). Characterizations (e.g. size, number and distribution) of each preparation were done using nanoparticle tracking analysis (NTA) (NanoSight, Malvern Instruments, Malvern, UK) as per protocols supplied by the manufacturer. For use in the assays outlined below, NP suspensions were sterile filtered using a 0.45-μm pore diameter membrane filter before use in cell culture.
Mouse splenic lymphocytes
Naïve C57BL/6J mice (female, 8–12-week-of-age) were obtained from the National Center for Laboratory Animal Sciences (National Institute of Nutrition, Hyderabad, India). All mice were housed under specific pathogen-free conditions in the University of Hyderabad Animal Facility maintained at 25 ± 2 °C with a 50% relative humidity and 12 h light–dark cycle. Mice were provided ad libitum access to a standard rodent chow pellet diet and filtered water. The Institutional Animal Ethics Committee of the University of Hyderabad approved all of these studies.
As a source of splenic lymphocytes for these studies, each mouse was euthanized by ether anesthesia and their spleen aseptically removed at necropsy. The organ was placed in RPMI 1640 containing 5% FCS (complete medium) and single-cell suspensions of splenic lymphocytes then prepared as described in Zimmerman et al. (Citation1977).
Human blood lymphocytes
Blood was drawn from consenting healthy volunteers at the University Health Center clinic. Lymphocytes from each heparinized sample were isolated by density gradient separation over Histopaque (Boyum Citation1964). After isolation, enumeration of cell number and viability were carried out and the samples were used in the assays below. The Human Ethics Committee of the University of Hyderabad approved all of these protocols prior to any blood draw.
Lymphocyte proliferation assay
Lymphocytes (0.2 × 106 in 200 μl complete medium) from the mice or humans were placed into dedicated wells of 96-well flat-bottomed microtiter plates (Corning, NY). Triplicate wells then received different concentrations of each NP and also either medium or mitogen (ConA, LPS, PHA or PWM) at their optimal concentrations (Kasyapa & Ramanadham Citation1992). After being cultured for 48 h at 37 °C in a 5% CO2 incubator, the cultures were pulsed with 0.5 μCi [3H]-thymidine and incubated a further 24 h. The cultures were then processed using an automatic cell harvester (Skatron, Oslo, Norway) and radioactivity in the harvested materials was measured in a scintillation counter (Beckman Coulter, Indianapolis, IN). The obtained cpm (counts per minute) for each sample was corrected for percentage of viable cells present. Proliferative responses were expressed in terms of stimulation index (SI) = [average cpm in presence of mitogen (or mitogen + NP)/average cpm with cells only]. Taking the SI of control cells stimulated with mitogen as 100%, proliferative responses for experimental samples treated with mitogen and NP were then calculated and expressed as % of control.
Determination of lymphocyte viability
Viability of the lymphocytes treated with different concentrations of each NP for 72 h (in absence of any mitogen) was determined using trypan blue dye exclusion. A minimum of 300 cells were evaluated/sample and data recorded for total cell number and dead cells (dye-stained). Viability was then expressed as % live of total cell number.
Statistical analysis
Data were expressed as means ± standard error of mean (SEM). Statistical analysis and significance were each evaluated using a one-way analysis of variance (ANOVA) followed by a post-hoc Tukey test. All analyzes were performed using Sigma Plot 11 software (Systat, San Jose, CA). Differences between control and treated samples were considered significant at p < .05.
Results
NP characterization
NTA data from a representative experiment are presented in ; pooled data from four individual NP preparations are presented (). The GNP showed a unimodal distribution () with mean and modal diameters of 50 and 28 nm, respectively. The average number of particles in the GNP suspension was 0.59 × 109/μg (). The SNP also showed a unimodal distribution () with mean and modal diameters of 76 and 66 nm, respectively. The average number of particles in the SNP suspension was 0.48 × 109/μg ().
Figure 1. Size distribution and particle concentration. Measurements obtained using NTA. (A) GNP. (B) SNP. Graph presented is representative of four independent experiments. x-axis: diameter (nm); y-axis: particle number (×106/ml).
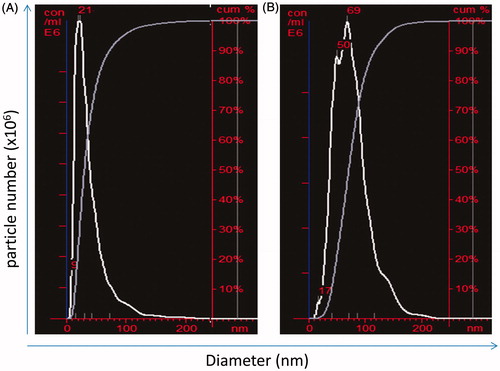
Table 1. Distribution of size and number determined by NTA.
Effect of GNP and SNP on murine splenic lymphocyte and human PBL viability
Murine splenic lymphocytes and human PBL were incubated with GNP or SNP for 72 h and their viability then determined. Viability of both populations was significantly reduced only by 200 μg GNP/ml (). In comparison, SNP inhibited viability of murine splenic lymphocytes at 25 and 50 μg/ml, whereas viability of human PBL was inhibited only at 50 μg/ml ().
Effect of GNP and SNP on [3H]-thymidine incorporation by unstimulated murine splenic lymphocytes and human PBL
[3H]-Thymidine incorporation by unstimulated lymphocytes in a presence of increasing levels of GNP and SNP is shown in . Incorporation was unaffected by GNP at all the levels tested and remained near that of the control cultures (). Interestingly, SNP appeared to somewhat stimulate the mouse cells at most of the test doses, but these effects were not significant (). At 50 μg SNP/ml, the effect on mouse cells led to a value significantly lower level of thymidine incorporation than that of the control cells (p < .05). In comparison, the human cells were moreover unaffected by the SNP, albeit that there again was significantly inhibited incorporation at 50 μg SNP/ml (p < .05, ).
Effect of GNP and SNP on mitogen-induced in vitro proliferative response of murine splenic lymphocytes and human PBL
[3H]-Thymidine incorporation for each sample was corrected for corresponding cell viability and the SI then calculated. Thus, data presented in represents proliferative responses of equal numbers of viable lymphocytes in each sample.
Figure 4. Effects of NP on mitogen-stimulated lymphocyte proliferative response of murine splenic lymphocytes. (A) GNP. (B) SNP. Mean stimulation indices with mitogens: LPS =23 (±1), ConA =25 (±1) are normalized to 100% value. Data shown are means (±SEM) of three experiments. *p < .05, NP treated versus control.
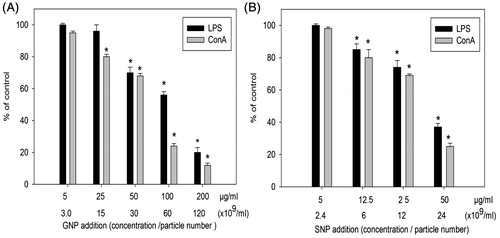
When murine lymphocytes were incubated with GNP at 5–200 μg/ml (3.0–120 × 109 particles/ml; NP:cell ratios of 3 × 103–120 × 103) in the presence of LPS or ConA, the ConA-stimulated proliferation was significantly inhibited starting at 25 μg GNP/ml (p < .05, ). The LPS-stimulated proliferation was inhibited starting at 50 μg GNP/ml (p < .05, ). When the mouse lymphocytes were treated with SNP at 5–50 μg/ml (2.4–24 × 109 particles/ml; NP:cell ratios of 2.4 × 103–24 × 103) in the presence of LPS or ConA, responses induced by each were significantly inhibited starting at 12.5 μg SNP/ml (p < .05, ). In both cases, the outcomes appeared dose-related but the effects as a function of specific mitogen did not differ.
With human cells, proliferative responses of PBL stimulated with PHA or PWM were significantly inhibited starting again at 25 μg GNP/ml (p < .05, ) and at 12.5 μg SNP/ml (p < .05, ). Again, in both cases, the outcomes appeared dose-related. However, in this set of studies, the effects as a function of specific mitogen did seem to differ, with the effects on induction by PHA being less impacted than that by PWM.
Figure 5. Effects of NP on mitogen-stimulated proliferative response of human PBL. (A) GNP. (B) SNP. Mean stimulation indices with mitogens: PHA =58 (±2) and PWM =24 (±1) are normalized to 100% value. Data shown are means (±SEM) of three experiments. *p < .05, NP treated versus control.
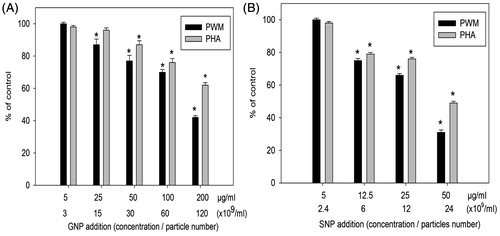
To evaluate whether free gold and silver ions released from NP could play a role in the observed effects, particle-free supernatants were collected from GNP and SNP incubated in culture medium. Specifically, GNP (50 and 200 μg/ml) and SNP (12.5 and 50 μg/ml) were incubated in RPMI 1640 containing 5% FCS for 72 h before NP-free supernatants were obtained by centrifugation under sterile conditions. Murine splenic lymphocytes and human PBL were then cultured in the above mentioned supernatants in the presence of mitogens (LPS, ConA, PHA and PWM) and proliferative responses assessed. Mitogen-induced proliferation was unaffected by a presence of GNP or SNP supernatants at the investigated concentrations (). Overall, mitogen-induced proliferation of murine splenic lymphocytes as well as human PBL was inhibited by GNP and SNP without any significant effect on cell viability.
Figure 6. Mitogen-stimulated proliferative responses of lymphocytes cultured in NP-free supernatants obtained from GNP and SNP incubated in complete medium for 72 h. (A) GNP G1 = 50 μg/ml, G2 = 200 μg/ml. (B) SNP: S1 = 12.5 μg/ml, S2 = 50 μg/ml. Mean stimulation indices with mitogens: LPS =22 (±1), ConA =17 (±2), PHA =21 (±1) and PWM =54 (±3) are normalized to 100% value. Data shown are means (±SEM) of three experiments.
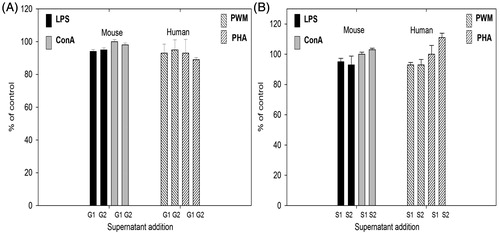
Discussion
Metallic NP have been proposed for wide use in biomedical imaging, cancer therapy and as anti-microbial agents (Almeida et al. Citation2014; Lara et al. Citation2011; Nune et al. Citation2009). In order to use NP as carriers of drugs and other biologically active ligands in vivo, it is necessary to evaluate their biocompatibility (Hanini et al. Citation2011; Liu et al. Citation2011). Investigations of immunomodulatory effects are essential for designing safe NP for use in imaging and clinical applications (Jiao et al. Citation2014). In the present work, putative immunomodulatory effects of GNP and SNP on mitogen-induced proliferative response and viability were evaluated in vitro. Murine splenic lymphocytes and human PBL stimulated by T- and B-cell-specific mitogens were used as the models.
Significant inhibition of mitogen-stimulated proliferation of murine lymphocytes and human PBL was noted by treatment with GNP and SNP at a concentrations ≥25 and ≥5 μg/ml, respectively. GNP <200 μg/ml had no effect on viability of either population used but viabilities were decreased with 25–50 μg SNP/ml. Because the data for proliferative responses were corrected for viable cell numbers, any significant inhibition of these responses were not due to any marginal decreases in viability by the GNP and SNP (at lower doses tested). The other background analyzes here showed that GNP had no effect on [3H]-thymidine incorporation by unstimulated human or mouse lymphocytes at all test concentrations. With SNP, significant inhibition in incorporation was only noted at 50 μg/ml. From all this we can surmise that any observed inhibition of mitogen-induced proliferative responses would not be due to any effects of the NP on [3H]-thymidine entry into cells/incorporation into cell DNA per se.
Enhancement of PHA-induced proliferative responses by human PBL due to GNP has been reported before (Liptrott et al. Citation2014). In an in vivo study using mice, orally administered GNP were able to differentially affect ex vivo lymphocyte proliferative responses—stimulatory at low doses and inhibitory at high doses (Małaczewska Citation2015). Dose-dependent increases in proliferative indices for CD3+ and CD4+ T-cells and no change in CD8+ T-cells were seen in human PBL treated in vitro with GNP (12 nm diameter) at concentrations of 1–25 μg/ml (Guevel et al. Citation2015). A few studies have reported that SNP were cytotoxic and/or modulated immune responses (Greulich et al. Citation2011; van der Zande et al. Citation2012). Differential effects on PHA-stimulated lymphocyte proliferative responses were also seen using SNP coated with polyvinyl–pyrrolidone + citrate; these SNP were stimulatory at 1 μg/ml but inhibitory at 10–40 μg/ml (Huang et al. Citation2016). In the present work, results suggested that SNP caused inhibitory (cytostatic) effects at low concentrations but cytotoxic effects at very high concentrations.
The findings reported here further indicate that metallic NP could potentially modulate lymphocyte function in situ in terms of inhibiting their activation by mitogens. The precise mechanisms by which NP bring about this inhibitory effect are not clear. As the NP are not internalized by lymphocytes, it is possible that the effects are mediated via their interactions with cell membranes or related components. It is also possible that cytostatic and cytotoxic effects observed could be due to release of respective metal ionic species from each NP. It has been proposed that ions released from metallic NP can bring about cellular and molecular toxicities, including cell death (Zhao et al. Citation2014).
In order to evaluate whether free gold and silver ions released from NP play a role in the inhibition of proliferative responses, particle-free supernatants were collected from GNP and SNP that had been incubated in culture medium. Mitogen-induced proliferative responses of splenic lymphocytes and human PBL cultured in these supernatants were identical to those of cells cultured in fresh medium. It has been shown by others that SNP release ions at negligible concentrations into culture medium containing serum (Loza et al. Citation2014). GNP have been shown to be stable in terms of release of ions into culture medium at pH 7.0 (Sabella et al. Citation2014). Thus, the effects on mitogen-induced proliferation by the GNP and SNP were not likely due to the release of ions from the parent particles. On the other hand, one cannot rule out that the particles/liberated ions were being bound up by the serum proteins during this particular 72-h incubation period. If that were the case, this does not rule out the potential for liberated ions to have acted on the cells in the initial 48-h incubation period before the tritiated thymidine was added. Clearly, further studies are needed to ascertain how these GNP/SNP are acting to inhibit responses to the mitogens.
Conclusions
The results of the present work indicate that GNP and SNP impact on lymphocyte stimulation in vitro and therefore could potentially compromise immune function in situ. Based on these initial sets of findings, future studies are needed to even better characterize any immunomodulatory potentials for GNP and SNP. The outcomes of such studies will clearly help to guide the design and evaluation of metallic NP for use in biomedical applications.
Disclosure statement
The authors report no conflicts of interest. The authors alone are responsible for the content of this article.
Funding
Financial support from the Department of Science and Technology, Government of India, under the Nanotechnology Mission to the University of Hyderabad is gratefully acknowledged.
References
- Almeida J, Figueroa E, Drezek R. 2014. Gold nanoparticle-mediated cancer immunotherapy. Nanomedicine. 10:503–514.
- Basu S, Ghosh S, Kundu S, Panigrahi S, Praharaj S, Pande S, Jana S, Pal T. 2007. Biomolecule induced nanoparticle aggregation: Effect of particle size on inter-particle coupling. J Colloid Interface Sci. 313:724–734.
- Becker K, Schroecksnadel S, Geisler S, Carriere M, Gostner J, Schennach H, Herlin N, Fuchs D. 2014. TiO2 nanoparticles and bulk material stimulate human peripheral blood mononuclear cells. Food Chem Toxicol. 65:63–69.
- Bhattacharya R, Patra C, Verma R, Kumar S, Greipp P, Mukherjee P. 2007. Gold nanoparticles inhibit the proliferation of multiple myeloma cells. Adv Mater. 19:711–716.
- Boyum A. 1964. Separation of white blood cells. Nature. 204:793–794.
- Butler K, Peeler D, Casey B, Dair B, Elespuru R. 2015. Silver nanoparticles: Correlating nanoparticle size and cellular uptake with genotoxicity. Mutagenesis. 30:577–591.
- Corot C, Robert P, Idee J, Port M. 2006. Recent advances in iron oxide nanocrystal technology for medical imaging. Adv Drug Deliv Rev. 58:1471–1504.
- Dobrovolskaia M, Aggarwal P, Hall J, McNeil SE. 2008. Preclinical studies to understand nanoparticle interaction with the immune system and its potential effects on nanoparticle biodistribution. Mol Pharm. 5:487–495.
- Dobrovolskaia M, McNeil S. 2007. Immunological properties of engineered nanomaterials. Nat Nanotechnol. 2:469–478.
- Elsabahy M, Wooley K. 2013. Cytokines as biomarkers of nanoparticle immunotoxicity. Chem Soc Rev. 21:5552–5576.
- Ghosh M, Manivannan J, Sinha S, Chakraborty A, Mallick S, Bandyopadhyay M, Mukherjee A. 2012. In vitro and in vivo genotoxicity of silver nanoparticles. Mutat Res. 749:60–69.
- Greulich C, Diendorf J, Gessmann J, Simon T, Habijan T, Eggeler G, Schildhauer T, Epple M, Köller M. 2011. Cell type-specific responses of peripheral blood mononuclear cells to silver nanoparticles. Acta Biomater. 7:3505–3514.
- Guevel X, Palomares F, Torresb M, Blancab M, Fernandez T, Mayorgab C. 2015. Nanoparticle size influences proliferative responses of lymphocyte sub-populations. RSC Adv. 5:85305–85309.
- Hanini A, Schmitt A, Kacem K, Chau F, Ammar S, Gavard J. 2011. Evaluation of iron oxide nanoparticle biocompatibility. Int J Nanomed. 6:787–794.
- Huang H, Lai W, Cui M, Liang L, Lin Y, Fang Q, Liu Y, Xie L. 2016. An evaluation of blood compatibility of silver nanoparticles. Sci Rep. 6:25518. doi: 10.1038/srep25518.
- Hussain S, Vanoirbeek J, Hoet P. 2012. Interactions of nanomaterials with the immune system. Wiley Interdiscip Rev Nanomed Nanobiotechnol. 4:169–183.
- Jiao Q, Li L, Mu Q, Zhang Q. 2014. Immunomodulation of nanoparticles in nanomedicine applications. Biomed Res Intl. 2014:426028.
- Kasyapa C, Ramanadham M. 1992. Alkaline phosphatase activity is expressed only in B-lymphocytes committed to proliferation. Immunol Lett. 31:111–116.
- Lara H, Garza-Treviño E, Ixtepan-Turrent L, Singh D. 2011. Silver nanoparticles are broad-spectrum bactericidal and virucidal compounds. J Nanobiotechnology. 9:30. doi: 10.1186/1477-3155-9-30.
- Lee P, Meisel D. 1982. Adsorption and surface-enhanced Raman dyes on silver and gold sols. J Phys Chem. 86:3391–3395.
- Liptrott N, Kendall E, Nieves D, Farrell J, Rannard S, Fernig D, Owen A. 2014. Partial mitigation of gold nanoparticle interactions with human lymphocytes by surface functionalization with a 'mixed matrix'. Nanomedicine. 9:2467–2479.
- Liu Y, Chen Z, Wang J. 2011. Systematic evaluation of biocompatibility of magnetic Fe3O4 nanoparticles with six different mammalian cell lines. J Nanopart Res. 13:199–212.
- Liu X, Huang N, Li H, Jin Q, Ji J. 2013. Surface and size effects on cell interaction of gold nanoparticles with both phagocytic and nonphagocytic cells. Langmuir. 29:9138–9148.
- Loza K, Diendorf J, Sengstock C, Ruiz-Gonzalez L, Gonzalez-Calbet J, Vallet-Regi M, Koller M, Epple M. 2014. Dissolution and biological effects of silver nanoparticles in biological media. J Mater Chem. 2:1634–1643.
- Małaczewska J. 2015. The splenocyte proliferative response and cytokine secretion in mice after oral administration of commercial gold nano-colloid. Pol J Vet Sci. 18:181–189.
- Mironava T, Hadjiargyrou M, Simon M, Jurukovski V, Rafailovich M. 2010. Gold nanoparticles cellular toxicity and recovery: Effect of size, concentration and exposure time. Nanotoxicology. 4:120–137.
- Mukherjee P, Bhattacharya R, Bone N, Lee Y, Patra C, Wang S, Lu L, Secreto C, Banerjee P, Yaszemski M, et al. 2007. Potential therapeutic application of gold nanoparticles in B-chronic lymphocytic leukemia (BCLL): Enhancing apoptosis. J Nanobiotechnol. 5:4. doi: 10.1186/1477-3155-5-4.
- Nune S, Gunda P, Thallapally P, Lin Y, Forrest M, Berkland C. 2009. Nanoparticles for biomedical imaging. Expert Opin Drug Deliv. 6:1175–1194.
- Rai M, Yadav A, Aniket G. 2009. Silver nanoparticles as a new generation of antimicrobials. Biotechnol Adv. 27:76–83.
- Sabella S, Carney R, Brunetti V, Malvindi M, Al-Juffali N, Vecchio G, Janes S, Bakr O, Cingolani R, Stellacci F, et al. 2014. A general mechanism for intracellular toxicity of metal-containing nanoparticles. Nanoscale. 6:7052–7061.
- Tomic S, Dokic J, Vasilijic S, Ogrinc N, Rudolf R, Pelicon P, Vucevic D, Milosavljevic P, Jankovic S, Anzel I, et al. 2014. Size-dependent effects of gold nanoparticles uptake on maturation and anti-tumor functions of human dendritic cells in vitro. PLoS One. 9:e96584. doi: 10.1371/journal.pone.0096584.
- van der Zande M, Vandebriel R, van Doren E, Kramer E, Rivera Z, Serrano-Rojero C, Gremmer E, Mast J, Peters R, Hollman P, et al. 2012. Distribution, elimination, and toxicity of silver nanoparticles and silver ions in rats after 28-day oral exposure. ACS Nano. 6:7427–7442.
- Vance M, Kuiken T, Vejerano E, McGinnis S, Hochella M, Rejeski D, Hull M. 2015. Nanotechnology in the real world: Redeveloping the nanomaterial consumer products inventory. Beilstein J Nanotechnol. 6:1769–1780.
- Vergallo C, Panzarini E, Izzo D, Carata E, Mariano S, Buccolieri A, Serra A, Manno D, Dini L. 2014. Cytotoxicity of β-D-glucose coated silver nanoparticles on human lymphocytes. AIP Conf Proc. 1603:78–86.
- Villiers C, Freitas H, Couderc R, Villiers M, Marche P. 2010. Analysis of the toxicity of gold nanoparticles on the immune system: Effect on dendritic cell functions. J Nanopart Res. 12:55–60.
- Zimmerman D, Gregory S, Kern M. 1977. Differentiation of lymphoid cells: The preferential binding of the lipid A moiety of lipopolysaccharide to B-lymphocyte populations. J Immunol. 119:1018–1023.
- Zhao Y, Wang B, Feng W, Bai C. 2014. Nanotoxicology: Toxicological and biological properties of nanomaterials. Encyclopedia of Life Support Systems; [cited 2016 Aug 10]. Available from: http://www.eolss.net.
- Zhornik E, Baranova L, Drozd E, Sudas M, Chau N, Buu N, Dung T, Chizhik S, Volotovski I. 2014. Silver nanoparticles induce lipid peroxidation and morphological changes in human lymphocytes surface. Biophysics. 59:380–386.
- Zolnik B, Gonzalez-Fernandez A, Sadrieh N, Dobrovolskaia M. 2010. Nanoparticles and the immune system. Endocrinology. 151:458–465.