Abstract
The autophagy receptor CALCOCO2/NDP52 functions as a bridging adaptor and plays an essential role in the selective autophagic degradation of invading pathogens by specifically recognizing ubiquitin-coated intracellular pathogens and subsequently targeting them to the autophagic machinery; thereby it is required for innate immune defense against a range of infectious pathogens in mammals. However, the mechanistic basis underlying CALCOCO2-mediated specific recognition of ubiqutinated pathogens is still unknown. Here, using biochemical and structural analyses, we demonstrated that the cargo-binding region of CALCOCO2 contains a dynamic unconventional zinc finger as well as a C2H2-type zinc-finger, and only the C2H2-type zinc finger specifically recognizes mono-ubiquitin or poly-ubiquitin chains. In addition to elucidating the specific ubiquitin recognition mechanism of CALCOCO2, the structure of the CALCOCO2 C2H2-type zinc finger in complex with mono-ubiquitin also uncovers a unique zinc finger-binding mode for ubiquitin. Our findings provide mechanistic insight into how CALCOCO2 targets ubiquitin-decorated pathogens for autophagic degradations.
Abbreviations
CALCOCO2 | = | calcium-binding and coiled-coil domain 2 |
DAPI | = | 4′,6-diamidino-2-phenylindole |
GABARAP | = | GABA(A) receptor-associated protein |
GIR | = | LGALS8/galectin 8 interacting region |
HSQC | = | heteronuclear single-quantum coherence |
ITC | = | isothermal titration calorimetry |
LC3 | = | microtubule-associated protein 1 light chain 3 |
LIR | = | LC3-interacting region |
LRSAM1 | = | leucine rich repeat and sterile α motif containing 1 |
NMR | = | nuclear magnetic resonance |
Ni2+-NTA | = | nickel-charged nitrilotriacetic acid |
S. typhimurium | = | Salmonella enterica serovar Typhimurium |
Ub | = | ubiquitin |
UBD | = | ubiquitin-binding domain |
WT | = | wild type |
ZF | = | zinc finger. |
Introduction
Autophagy is an evolutionarily conserved and tightly regulated lysosome-dependent intracellular catabolic process involving degradation of cytoplasmic components including bulk protein aggregates, dysfunctional organelles, and invading pathogens for cellular homeostasis and/or adaptation in response to multiple cellular stresses in eukaryotes.Citation1-4 As a unique subtype of autophagy, macroautophagy (hereafter referred to as autophagy) relies on double-membraned vesicles named autophagosomes, which are generated from a precursor termed the phagophore that envelopes and sequesters portions of the cytosol for delivery to lysosomes.Citation5,6 Traditionally, autophagy was deemed as a nonselective bulk process, which indiscriminately engulfs some cytosolic components for autophagic degradation. Recently, increasing evidence shows that the removal of some protein aggregates and elimination of some intracellular pathogens are highly selective processes that require specific cargo recognition by the autophagy machinery.Citation7-9 During these selective autophagy processes, a panel of unique adaptor proteins termed as autophagy receptors play essential roles.Citation7,10 So far, the identified autophagy receptors in mammals, such as SQSTM1/p62, CALCOCO2/NDP52, OPTN/optineurin, NBR1 (neighbor of BRCA1 gene 1), BNIP3L/Nix, FUNDC1 (FUN14 domain containing 1), STBD1 (starch binding domain 1) and TOLLIP (toll interacting protein), all contain a cargo-associating domain that can specifically recognize certain types of cytosolic cargoes and a LC3-interacting region (LIR) that can recruit the key autophagic factors, the Atg8 family proteins, including MAP1LC3A, MAP1LC3B, MAP1LC3C, GABARAP, GABARAPL1, and GABARAPL2 in mammals.Citation11-16 The Atg8 proteins attached to the nascent phagophore membrane by conjugation with phosphatidylethanolamine are critical for autophagosome formation.Citation17 Thereby, autophagy receptors can simultaneously bind prospective cargoes and Atg8 proteins to function as bridging adaptors coupling the specific cargoes with autophagy machinery, and subsequently target the substrates for autophagic degradation. Based on different targeting cargoes, several distinct types of selective autophagy have been described to date, such as aggrephagy (selective autophagy of aggregated proteins), xenophagy (selective autophagy of pathogens), mitophagy (selective autophagy of mitochondria), and the recently identified ferritinophagy (selective autophagy of ferritin).Citation7,18-21
Similar to the ubiquitin-proteasome degradation system, modification of cargoes with ubiquitin also serves as a common cargo targeting and degradation signal in selective autophagy.Citation22,23 Many cargoes of selective autophagy need to be marked with polyubiquitin chains before they can be specifically recognized by autophagy receptors.Citation23 For instance in xenophagy, once the invading bacteria or viruses escape from their dedicated vacuoles and enter the cytoplasm, they would be detected by the cellular innate immune system and decorated with poly-ubiquitin chains, which serve as recognition signals for the autophagy receptors to recruit downstream autophagic machinery.Citation21,24 Ubiquitin has 7 lysine residues (Lys6, Lys11, Lys27, Lys29, Lys33, Lys48, and Lys63) and an extreme N-terminal methionine residue, which can be used to form 8 distinct linkage types of ubiquitin chains.Citation25 Different linkage types of ubiquitin chains perform specific cellular functions and can be selectively recognized by ubiquitin-binding domains (UBD) with suitable linkage-type specificities in mammalian cells.Citation25-28 Although the ubiquitin chain types associated with selective autophagy are not well defined, the K48-linked, K63-linked and methionine 1 (M1)-linked ubiquitin chains are found on the surface of ubiquitin-decorated invading pathogens and implicated as specific degradation signals in xenophagy.Citation29-31 However, the relevant substrates modified by ubiquitin, the E3 ubiquitin ligases responsible for ubiquitination, and how the ubiquitin-coated pathogens are selectively recognized by specific autophagy receptors are still largely unknown.
CALCOCO2/NDP52, SQSTM1, and OPTN/optineurin are 3 ubiquitin-binding autophagy receptors involved in the selective autophagic degradation of invading pathogens.Citation32-36 In particular, CALCOCO2 participates in the xenophagy of a range of infectious pathogens including Salmonella enterica serovar Typhimurium (hereafter, S. typhimurium), Listeria monocytogenes, Mycobacterium tuberculosis and Shigella.Citation30,34,37 As an autophagy receptor, CALCOCO2 contains a N-terminal SKIP carboxyl homology (SKICH) domain that is responsible for the interaction with AZI2/NAP1,Citation37 a TBK1 (TANK-binding kinase 1)-binding adaptor required for virus- and poly(I:C)-induced IRF activation, and a noncanonical LIR motif that can specifically interact with the Atg8 ortholog LC3C ().Citation38 In addition, the middle region of CALCOCO2 is predicted to form a coiled-coil domain, which can induce the dimerization of CALCOCO2 and associate with the identified E3 ubiquitin ligase LRSAM1 (leucine rich repeat and sterile α motif containing 1) for anti-Salmonella xenophagy.Citation39 Interestingly, missense mutation of CALCOCO2 in the middle coiled-coil region is linked to Crohn disease,Citation40,41 a type of inflammatory bowel disease mainly caused by a defect in antibacterial autophagy. The C-terminal cargo-binding region of CALCOCO2 consists of a galectin 8-interacting region (GIR) that can specifically bind to the sugar receptor LGALS8/galectin 8,Citation42 and a zinc finger (ZF)-containing region that can selectively recognize ubiquitin-coated pathogens.Citation37 Recently, the underlying molecular mechanism governing the specific interaction between CALCOCO2 and LGALS8 was well characterized;Citation43,44 however, the biochemical and structural mechanisms governing the selective recognition of ubiquitin by CALCOCO2 are still poorly understood. Meanwhile, both CALCOCO2 and SQSTM1 contain a LIR motif and a UBD domain, but they are recruited independently to nonoverlapping microdomains of ubiquitin-coated bacteria and are functionally nonredundant in antibacterial autophagy.Citation33,34 One potential explanation is that the UBD domains of CALCOCO2 and SQSTM1 have distinct ubiquitin chain-type binding specificity. A preference of SQSTM1 for K63-linked ubiquitin chains has been reported,Citation45 but no detailed information on CALCOCO2 has been available yet. Therefore, the preference of CALCOCO2 for different linkage types of ubiquitin chains remains to be elucidated.
Figure 1. NMR-based characterizations of the C-terminal cargo-binding region of CALCOCO2. (A) A schematic diagram showing the domain organization of CALCOCO2. The boundaries of CALCOCO2 used in the NMR study are indicated and boxed. (B) Overlay plot of the 1H-15N HSQC spectra of CALCOCO2(365-446) purified using Ni2+-NTA agarose and the protein in the presence of additional 1 mM ZnCl2, together with the protein purified using glutathione sepharose 4B without any imidazole treatment (CALCOCO2[365-446]_no imidazole). For clarity, the insert shows the enlarged view of a representative K395 residue, which has 2 sets of peaks in the 1H-15N HSQC spectrum of CALCOCO2(365-446) purified using Ni2+-NTA agarose. In this presentation, the peak only found in the 1H-15N HSQC spectrum of CALCOCO2(365-446) purified using Ni2+-NTA agarose is assigned a red number, while the other peak found in all 3 proteins is labeled with a black number. (C) Superposition plot of the 1H-15N HSQC spectra of CALCOCO2(365–446) purified using glutathione sepharose 4B and CALCOCO2(414–446). (D) Stereo view showing the backbones of 20 superimposed NMR structures of CALCOCO2(414–446). (E) The combined ribbon representation and stick model showing a representative NMR structure of the CALCOCO2(414–446). In this drawing, the side chains of 2 cysteine and 2 histidine residues involved in zinc ion coordination are further labeled and shown in the stick model, and the coordinated zinc ion is shown in the ball model.
![Figure 1. NMR-based characterizations of the C-terminal cargo-binding region of CALCOCO2. (A) A schematic diagram showing the domain organization of CALCOCO2. The boundaries of CALCOCO2 used in the NMR study are indicated and boxed. (B) Overlay plot of the 1H-15N HSQC spectra of CALCOCO2(365-446) purified using Ni2+-NTA agarose and the protein in the presence of additional 1 mM ZnCl2, together with the protein purified using glutathione sepharose 4B without any imidazole treatment (CALCOCO2[365-446]_no imidazole). For clarity, the insert shows the enlarged view of a representative K395 residue, which has 2 sets of peaks in the 1H-15N HSQC spectrum of CALCOCO2(365-446) purified using Ni2+-NTA agarose. In this presentation, the peak only found in the 1H-15N HSQC spectrum of CALCOCO2(365-446) purified using Ni2+-NTA agarose is assigned a red number, while the other peak found in all 3 proteins is labeled with a black number. (C) Superposition plot of the 1H-15N HSQC spectra of CALCOCO2(365–446) purified using glutathione sepharose 4B and CALCOCO2(414–446). (D) Stereo view showing the backbones of 20 superimposed NMR structures of CALCOCO2(414–446). (E) The combined ribbon representation and stick model showing a representative NMR structure of the CALCOCO2(414–446). In this drawing, the side chains of 2 cysteine and 2 histidine residues involved in zinc ion coordination are further labeled and shown in the stick model, and the coordinated zinc ion is shown in the ball model.](/cms/asset/602c7acf-0164-41d1-9dbf-a2a29cef6555/kaup_a_1082025_f0001_c.gif)
To address the molecular basis of ubiquitin recognition by the autophagy receptor CALCOCO2, herein we biochemically and structurally characterized the C-terminal cargo recognition region of CALCOCO2 and discovered that this region contains a dynamic unconventional zinc finger (ZF1) and a canonical C2H2-type zinc finger (ZF2). Interestingly, only the C2H2-type zinc finger can specifically bind to mono-ubiquitin or different linkage types of ubiquitin chains including K48-linked, K63-linked and M1-linked ubiquitin chains. The underlying molecular mechanism governing the specific interaction between CALCOCO2 and ubiquitin was elucidated by solving the crystal structure of CALCOCO2 ZF2 in complex with mono-ubiquitin, which represents the first atomic structure, to our knowledge, showing a C2H2-type zinc finger binding to a ubiquitin protein. Our findings expand our knowledge of the mechanism of ubiquitin-decorated pathogen recognition by the autophagy receptor CALCOCO2.
Results
CALCOCO2 contains a dynamic unconventional zinc finger and a C2H2-type zinc finger
Previously, it was reported that the C-terminal zinc finger-containing region of CALCOCO2 is responsible for binding to mono-ubiquitin.Citation37 As the first step toward understanding the mechanism of ubiquitinated pathogen recognition by CALCOCO2, we focused on this C-terminal region of this protein and carried out a detailed sequence alignment analysis. In addition to the well-characterized LGALS8 interacting motif (residues 372–380, referred to as “GIR”), the C-terminal region of CALCOCO2 contains 2 highly conserved ‘CPXC’ motifs (Fig. S1A), a signature sequence motif found in numerous zinc finger domains, indicating that this region may contain 2 zinc fingers (). Interestingly, this region of CALCOCO2 in some species also contains additional cysteine and histidine residues and was previously also classified as a LIM-like domain,Citation46 a protein-protein interaction-module coordinating 2 zinc ions.Citation47
Next, we sought to use NMR spectroscopy to elucidate the atomic structure of this C-terminal region of CALCOCO2. Interestingly, the 1H-15N HSQC spectrum of the C-terminal region of CALCOCO2 (residues 365–446) purified using glutathione sepharose affinity chromatography is different from the one purified using Ni2+-NTA agarose affinity chromatography, but is almost the same as the one purified using Ni2+-NTA agarose affinity chromatography and followed by adding 1 mM ZnCl2 (). Based on this observation, we inferred that this region of CALCOCO2 may contain a weak zinc binding site, which would partially lose the coordinated Zn2+ ion due to the high concentration of imidazole used during the purification procedure for the His-tagged protein, but could be restored to the Zn2+-coordinating stage once in the presence of additional Zn2+ ion.
After backbone chemical shift assignment of the CALCOCO2 sample purified using Ni2+-NTA agarose affinity chromatography, we found that only the region flanking the first ‘CPXC’ motif of CALCOCO2 (residues 392–413, referred hereafter as “ZF1”) showed 2 distinct sets of peaks in the 1H-15N HSQC spectrum (Fig. S2A and B), each likely corresponding to the Zn2+-bound and the Zn2+-unbound conformers, while other regions including the second ‘CPXC’ motif only showed one set of peaks (; Fig. S2B). Next, we determined the solution structure of the C-terminal region of CALCOCO2 (residues 365–446) purified using glutathione sepharose affinity chromatography. In the final structure, we could well define the extreme C-terminal region including the second ‘CPXC’ motif of CALCOCO2 (residues 414–446, referred hereafter as “ZF2”) that consists of 2 anti-parallel β-strands and a C-terminal α-helix, but we could only define a short α-helix in the ZF1 domain due to its intrinsic dynamic property, whereas the remaining region was too flexible to be well defined (Fig. S2C and D). Moreover, there was no detectable interdomain nuclear overhauser enhancement (NOE) between ZF1 and ZF2 (data not shown), indicating that there was no direct interaction between them.
To further verify the independent relationship between ZF1 and ZF2, we also purified the isolated ZF2 and found indeed its 1H-15N HSQC spectrum overlapped very well with that of the CALCOCO2 (residues 365–446) fragment containing both the ZF1 and ZF2 domains (), which is consistent with the NMR structure data. We further determined the structure of the isolated ZF2 to high resolution by NMR spectroscopy (; Table S1). The ZF2 domain coordinates a Zn2+ ion and folds into a canonical C2H2-type zinc finger. In particular, the Zn2+ ion is coordinated by the side chains of 2 cysteine residues from the ‘CPXC’ motif (Cys422 and Cys425), and 2 histidine residues located in the C-terminal α-helix (His440 and His444) (; Fig. S1A). We also confirmed that both ZF domains of CALCOCO2 could coordinate zinc ions using elementary analyses (Table S2). Taken together, we discovered that the C-terminal region of CALCOCO2 contains a dynamic ZF1 domain and a C2H2-type ZF2 domain rather than a LIM-like domain.
CALCOCO2 recognizes mono-ubiquitin and different linkage types of polyubiquitin chains through its C2H2-type zinc finger
As the C-terminal region of CALCOCO2 contains 2 zinc fingers, we wondered whether both of them were involved in ubiquitin binding. To test this hypothesis, we first investigated the interaction between CALCOCO2 and mono-ubiquitin. Using qualitative analytical gel filtration chromatography analysis, we found the CALCOCO2 fragment (residues 365–446) containing the tandem zinc finger domains or the ZF2 alone could specifically interact with mono-ubiquitin to form a good protein complex (Fig. S3A and B). Next, we took advantage of the powerful NMR spectroscopy to characterize the interaction between CALCOCO2 and mono-ubiquitin. Titration of the 15N-labeled CALCOCO2 fragment (residues 365–446) with mono-ubiquitin showed that a select set of peaks in the 1H-15N HSQC spectrum underwent significant dose-dependent peak-broadenings or chemical shift changes (), indicating that this CALCOCO2 fragment can specifically bind to mono-ubiquitin. When plotting the amide backbone chemical shift changes as a function of the residue number and mapping shift differences onto a representative solution structure of the C-terminal region of CALCOCO2 (residues 365–446) revealed that the significant backbone amide chemical shift changes were only rich in the ZF2 domain region of CALCOCO2 (residues 420–446), whereas the GIR motif and the ZF1 domain displayed negligible chemical shift changes (). This NMR-based characterization clearly demonstrated that only the ZF2 domain of CALCOCO2 was able to bind mono-ubiquitin. Finally, we quantitatively characterized the interactions between different CALCOCO2 fragments and mono-ubiquitin by measuring their binding affinities using isothermal titration calorimetry (ITC), and found that the long and short fragments of CALCOCO2 (residues 365–446 and 414–446) bound to mono-ubiquitin with similar Kd values, ∼35 μM and ∼39 μM, respectively (), confirming that the ZF2 domain alone was sufficient for binding to mono-ubiquitin.
Figure 2. Only the ZF2 domain of CALCOCO2 is able to bind ubiquitin. (A) Superposition plot of the 1H-15N HSQC spectra of CALCOCO2(365–446) titrated with increasing molar ratios of mono-ubiquitin. (B) Plot of backbone amide chemical shift differences as a function of the residue number of CALCOCO2(365–446) between the wild type and the protein titrated with mono-ubiquitin at the molar ratio of 1:1. The secondary structure of CALCOCO2(365–446) is also indicated at the top of the figure. The insert shows the shift changes mapped onto a representative NMR structure of CALCOCO2(365–446). In this representation, the combined 1H and 15N chemical shift changes are defined as: Δppm = [(ΔδHN)2 + (ΔδN×αN)2]1(2. Where ΔδHN and ΔδN represent chemical shift differences of amide proton and nitrogen chemical shifts of each residue of CALCOCO2(365–446). The scaling factor (αN) used to normalize the 1H and 15N chemical shift is 0.17. (C) ITC-based measurement of the binding affinity of CALCOCO2(365–446) with mono-ubiquitin. (D) ITC-based measurement of the binding affinity of CALCOCO2(414–446) with mono-ubiquitin.
![Figure 2. Only the ZF2 domain of CALCOCO2 is able to bind ubiquitin. (A) Superposition plot of the 1H-15N HSQC spectra of CALCOCO2(365–446) titrated with increasing molar ratios of mono-ubiquitin. (B) Plot of backbone amide chemical shift differences as a function of the residue number of CALCOCO2(365–446) between the wild type and the protein titrated with mono-ubiquitin at the molar ratio of 1:1. The secondary structure of CALCOCO2(365–446) is also indicated at the top of the figure. The insert shows the shift changes mapped onto a representative NMR structure of CALCOCO2(365–446). In this representation, the combined 1H and 15N chemical shift changes are defined as: Δppm = [(ΔδHN)2 + (ΔδN×αN)2]1(2. Where ΔδHN and ΔδN represent chemical shift differences of amide proton and nitrogen chemical shifts of each residue of CALCOCO2(365–446). The scaling factor (αN) used to normalize the 1H and 15N chemical shift is 0.17. (C) ITC-based measurement of the binding affinity of CALCOCO2(365–446) with mono-ubiquitin. (D) ITC-based measurement of the binding affinity of CALCOCO2(414–446) with mono-ubiquitin.](/cms/asset/6a8aac3b-1460-48c7-98fa-03e411fef099/kaup_a_1082025_f0002_c.gif)
Because the ubiquitin-coated pathogens recognized by CALCOCO2 are mainly modified with poly-ubiquitin chains,Citation21,24,30 we sought to know whether CALCOCO2 had any binding selectivity toward different linkage types of ubiquitin chains, and whether both ZF domains of CALCOCO2 participated in polyubiquitin chain binding. We synthesized the K63-linked, K48-linked and M1-linked ubiquitin chains that are implicated in xenophagy,Citation29-31 and tested their interactions with different CALCOCO2 fragments using the same approaches as for mono-ubiquitin. Analytical gel filtration analyses showed that both the tandem ZF domains and the ZF2 domain alone could interact with the K63-linked, K48-linked and M1-linked di-ubiquitin as well as the M1-linked tetra-ubiquitin (Fig. S3C–J). Moreover, NMR titration experiments showed that the 1H-15N HSQC spectra of CALCOCO2 (residues 365–446) titrated with increasing molar ratios of di-ubiquitin chains linked either by K63, K48 or M1 displayed significant changes (Fig. S4A, C and E), revealing that CALCOCO2 could specifically recognize these different linkage types of ubiquitin chains.
Further detailed analyses showed that the significant chemical shift changes induced by these di-ubiquitin proteins bindings were only restrained to the ZF2 region (Fig. S4B, D and F), demonstrating that only the ZF2 domain of CALCOCO2 was able to recognize these linkage types of ubiquitin chains. In contrast, the ZF2 region showed no notable chemical shift changes when CALCOCO2 was titrated with LGALS8 (Fig. S4G and H). In addition, ITC-based measurements of the interactions between 2 monomeric CALCOCO2 fragments (residues 365–446 and 414–446) and different di-ubiquitin chains linked by K63, K48 or M1 gave similar binding stoichiometry values of ∼0.5 and Kd values of ∼20 μM (; Fig. S5A–C and E–G). Moreover, the measured Kd and stoichiometry values of the 2 monomeric CALCOCO2 fragments (residues 365–446 and 414–446) with M1-linked tetra-ubiquitin chains were also very similar, ∼10 μM and ∼0.25, respectively (; Fig. S5D and H). These quantitative ITC results not only confirmed that only the ZF2 domain of CALCOCO2 was involved in the polyubiquitin chain binding but also indicated that ZF2 domain could simultaneously and indiscriminately bind to every ubiquitin molecule of the M1-, K48-, K63-linked di-ubiquitin or the M1-linked tetra-ubiquitin chains.
Table 1. Statistics of measured ITC results between various forms of CALCOCO2 and ubiquitin proteins or their mutants. Kd shows the binding affinities, N shows the binding stoichiometry, ΔH shows the enthalpy change, ΔS shows the entropy change, these values were obtained from ITC experiments. While ΔG shows the Gibbs energy change that is defined as: ΔG = ΔH - 298*ΔS. ‘N.D.’ stands for that the value is not detectable
We also quantitatively measured the binding affinities of the full-length CALCOCO2 (residues 1–446) with the di-ubiquitin chains linked by K63, K48, and M1 using ITC. The full-length CALCOCO2 contained the middle coiled-coil domain that can mediate the dimerization of CALCOCO2 (data not shown). The measured Kd and stoichiometry values for full-length CALCOCO2 with different di-ubiquitin chains were also similar and around 10 μM and 0.25, respectively (; Fig. S5I-K), roughly corresponding to a 2-fold increase of binding affinities but a 50% decrease of binding stoichiometry values compared with that of the monomeric CALCOCO2 fragments (residues 365–446 and 414–446). Given that full-length CALCOCO2 functions as a dimer, these results suggested that rather than synergizing with each other in binding to 2 ubiquitin molecules of a M1-, K48-, or K63-linked di-ubiquitin, the 2 ZF2 domains of a full-length CALCOCO2 dimer bind to 2 separate di-ubiquitin proteins. Since we could not synthesize sufficient amounts of M1-, K63- or K48-linked longer poly-ubiquitin chains for ITC analysis, we cannot rule out the possibility that the 2 ZF2 domains of a full-length CALCOCO2 dimer could synergize with each other in binding to a M1-, K48-, or K63-linked poly-ubiquitin chain that is long enough. Nevertheless, all the above qualitative and quantitative data clearly demonstrated that CALCOCO2 specifically recognizes different linkage types of ubiquitin chains only mediated by its ZF2 domain.
Overall structure of the CALCOCO2 ZF2 domain in complex with mono-ubiquitin
To elucidate the detailed molecular mechanism governing the specific interaction between CALCOCO2 and ubiquitin, we sought to determine the structure of the CALCOCO2 ZF2 domain in complex with mono-ubiquitin. However, initial attempts using NMR spectroscopy failed due to the poor homogeneity of the 1H-15N HSQC spectrum of the ZF2 domain in complex with mono-ubiquitin (). Therefore, we resorted to X-ray crystallography to solve this complex structure. Fortunately, the ZF2-mono-ubiquitin complex formed good crystals that diffracted to 2.1-Å resolution. The complex structure was solved using the molecular replacement method (Table S3). In the final refined model, each asymmetric unit contained 2 ZF2-mono-ubiquitin complexes that are highly similar to each other (root mean squared deviation = 0.5 Å), and each ZF2-mono-ubiquitin complex had a 1:1 stoichiometry (Fig. S6A; Fig. 3A). In the complex structure, the ZF2 domain adopted a canonical C2H2-type zinc finger fold and was very similar to that of the isolated domain in solution (Fig. S6B and C), and the mono-ubiquitin formed a typical ubiquitin architecture consisting of a 5-stranded β-sheet, a short 310 helix and a 3.5-turn α-helix ().
Figure 3. The overall structure of CALCOCO2 ZF2 in complex with mono-ubiquitin. (A) The combined ribbon representation and stick model showing the overall structure of the CALCOCO2 ZF2-mono-ubiquitin complex. In this drawing, the CALCOCO2 ZF2 is shown in forest green, mono-ubiquitin in orange. The side chains of 2 cysteine and 2 histidine residues involved in zinc ion coordination are shown in the stick model, and the coordinated zinc ion is shown in the ball model. (B) Surface representations showing the overall architecture of CALCOCO2 ZF2-mono-ubiquitin complex (left panel), and the “open-book” view of the binding interface between CALCOCO2 ZF2 and mono-ubiquitin (right panel) with the same color scheme as in panel (A). (C) The combined surface representation and the ribbon-stick-dot model showing the orientations of 8 residues (M1, K6, K11, K27, K29, K33, K48, and K63) of mono-ubiquitin that can be used for poly-ubiquitin linkage formations in the ZF2-mono-ubiquitin complex. In this presentation, the mono-ubiquitin molecule is shown in the ribbon-stick-dot model and CALCOCO2 ZF2 in the surface model.
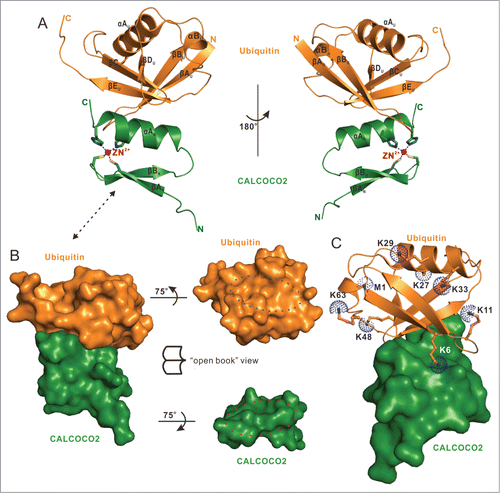
The binding of the ZF2 domain also did not induce large conformational changes to the mono-ubiquitin protein (Fig. S6D and E). Consistent with the NMR-based analysis (), only the C-terminal αA helix of ZF2 was directly involved in ubiquitin-binding, and packed against a solvent-exposed patch located in the central β-sheet of ubiquitin, covering a total of ∼500ÅCitation2 surface area (). The overall ubiquitin-binding mode of the CALCOCO2 ZF2 was similar to that of the RABGEF1/Rabex-5 zinc finger and the POLN/Polη zinc finger, which both exclusively utilize the C-terminal α-helix to interact with the solvent-exposed surface of the central β-sheet of ubiquitin.Citation48-50 In comparison with other currently known zinc finger-ubiquitin complex structures, of which the ubiquitin-binding zinc fingers are either a C2HC-type or a C4-type zinc finger, the CALCOCO2 ZF2-mono-ubiquitin complex solved in this study represents the first atomic structure showing a C2H2-type zinc finger binding to a ubiquitin protein (Fig. S7A–M). Further detailed structural analysis indicated that among the 8 residues (M1, K6, K11, K27, K29, K33, K48 and K63) that can be used for poly-ubiquitin linkage formation, only the Lys6 residue of ubiquitin was approaching the ZF2 domain, whereas the other 6 lysine residues and the N-terminal Met1 residue were away from the ZF2 domain (). Therefore, except the K6-linked poly-ubiquitin chain, all the other linkage types of poly-ubiquitin chains are likely to be recognized by the ZF2 domain.
The molecular interface of the ZF2-mono-ubiquitin complex
Further detailed structure analysis of the binding interface of ZF2-mono-ubiquitin complex showed that the specific interaction between CALCOCO2 ZF2 and mono-ubiquitin was mainly mediated by hydrophobic and polar interactions (). In particular, the hydrophobic side chains of Phe442, Cys443, and Leu446 from the C-terminal α-helix of CALCOCO2 packed extensively with the canonical ubiquitin hydrophobic patch formed by the side chains of Leu8, Ile44 and Val70. In addition, the polar side chain groups of Asp439 and Gln435 from the C-terminal α-helix of CALCOCO2 formed 3 hydrogen bonds with the backbone amides of Ala46 and Gly47 as well as the backbone oxygen of Thr66 from ubiquitin, respectively. Moreover, the complex was further stabilized by a pair of salt bridge formed between the negatively charged carboxyl group of Leu446 located at the extreme C terminus of CALCOCO2 and the positively charged residue Arg42 located in the βC-strand of ubiquitin. Importantly, all these key residues involved in the binding interface were highly conserved during evolution (Fig. S1). Using the GST-fusion protein affinity isolation assay and the quantitative ITC analysis, we further verified the specific interaction between the ZF2 domain and ubiquitin. Deletion of the ZF2 domain or individual mutation of the key residues involved in the binding interface of the complex either from ubiquitin or CALCOCO2, such as the I44A, V70E mutations of ubiquitin and the D439R, C443K mutations of CALCOCO2, essentially abolished the specific interaction between CALCOCO2 and ubiquitin (, Fig. 4D; Fig. S8A–D). As a control, deletion of the N-terminal SKICH domain of CALCOCO2 had little effect on the interaction between CALCOCO2 and ubiquitin ().
Figure 4 (See previous page). Molecular detail of the CALCOCO2 ZF2 and mono-ubiquitin interaction. (A) The combined surface representation and the ribbon-stick model showing the hydrophobic interaction interface between CALCOCO2 ZF2 and mono-ubiquitin. In this presentation, the mono-ubiquitin molecule is shown in the surface model and CALCOCO2 ZF2 in the ribbon-stick model. The hydrophobic amino acid residues in mono-ubiquitin surface model are drawn in yellow, the positively charged residues in blue, the negatively charged residues in red, and the uncharged polar residues in gray. (B) The combined surface charge representation and the ribbon-stick model showing the charge-charge interactions between CALCOCO2 ZF2 and mono-ubiquitin. (C) Stereo view showing the detailed interactions between CALCOCO2 ZF2 and mono-ubiquitin. The hydrogen bonds involved in the binding are shown as dotted lines. (D) GST affinity isolation assays to verify the specific interaction between CALCOCO2 ZF2 and ubiquitin as well as the key binding interface residues. The left panel showing the GST affinity isolation assay using purified GST-tagged ubiquitin protein and various CALCOCO2 deletion mutants, confirming that the CALCOCO2 ZF2 domain is crucial for ubiquitin binding. The middle panel showing the GST affinity isolation assay using purified GST-tagged ubiquitin mutants and CALCOCO2 protein, confirming the important interface residues I44 and V70 of ubiquitin in binding to CALCOCO2. The right panel showing the GST affinity isolation assay using purified GST-GB-tagged CALCOCO2 ZF2 mutants and Trx-4Ub (linked by M1), confirming the key interface residues D439 and C443 of CALCOCO2 in binding to ubiquitin.
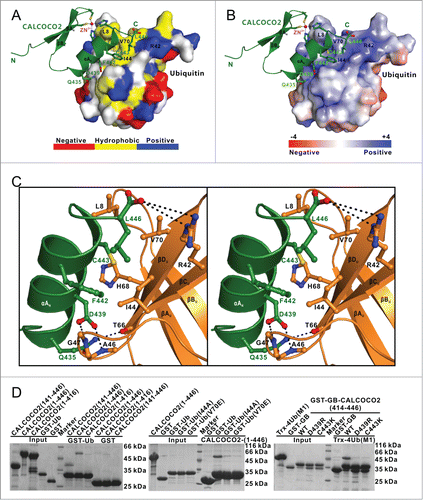
Consistent with the GST-fusion protein affinity isolation assay and the quantitative ITC analysis, co-immunoprecipitation experiments in HEK293T cells showed that deletion of the CALCOCO2 ZF2 domain (CALCOCO2[1–416] fragment) and mutations of key interface residues of the ZF2-ubiquitin complex, such as D439R, C443K mutations of CALCOCO2 and I44A, V70E mutations of ubiquitin, all remarkably decreased the specific interactions between CALCOCO2 and ubiquitin (Fig. S8E). Intriguingly, further detailed structural and sequence comparisons revealed that although the ubiquitin recognition by the CALCOCO2 ZF2 helix was similar to that of RABGEF1 and POLN zinc fingers, the CALCOCO2 ZF2 used a unique binding mode that features a D(-4)-C(0)-L(+3) motif along the ubiquitin binding-helix rather than the D(-4)-A(0)-L(+3) motif shared by RABGEF1 and POLN (Fig. S9A and B). Accordingly, the CALCOCO2 ZF2-mono-ubiquitin complex structure solved in this study uncovered a novel zinc finger-binding mode for ubiquitin.
Cellular colocalization of CALCOCO2 with ubiquitin-coated invading bacteria required the specific interaction between ZF2 and mono-ubiquitin
CALCOCO2 colocalizes with ubiquitin-coated invading pathogens, such as S. typhimurium, and mediates their destruction by the autophagy pathway in human cells.Citation37,51 Therefore, we used HeLa cells infected with S. typhimurium as the model system to evaluate the role of the ZF2-mono-ubiquitin interaction in the cellular localization of CALCOCO2 and ubiquitin-coated invading bacteria. After infection of CALCOCO2-transfected HeLa cells with S. typhimurium, the Cherry-tagged CALCOCO2 formed dense puncta and colocalized well with the ubiquitin-positive invading S. typhimurium (). In contrast, the CALCOCO2 fragment (residues 1–416) lacking the ZF2 domain was largely diffuse throughout the cytosol and almost completely lost its ability to colocalize with the ubiquitin-coated invading S. typhimurium (), in line with the in vitro biochemical study that the ZF2 domain was essential for the interaction between CALCOCO2 and ubiquitin ().
Figure 5 (See previous page). The specific ZF2-mono-ubiquitin interactions are required for the cellular recruitment of CALCOCO2 to the ubiquitin-coated invading S. typhimurium. (A-D) Confocal micrographs of HeLa cells overexpressing Cherry-tagged CALCOCO2 or its mutants infected with S. typhimurium, and stained with antibodies to ubiquitin 2 h after infection. (A) Full-length CALCOCO2 colocalizes well with ubiquitin-coated invading S. typhimurium. (B) Deletion of the ZF2 domain of CALCOCO2 abolished the colocalization of CALCOCO2 and ubiquitin-coated S. typhimurium. ((C)and D) Point mutations of key interface residues of CALCOCO2 that disrupt CALCOCO2 ZF2 and mono-ubiquitin interaction essentially eliminated the colocalization of CALCOCO2 and ubiquitin-coated S. typhimurium. (E) Statistical result related to the colocalization of ubiquitin-positive invading S. typhimurium with CALCOCO2 2 or 4 h after infection. All data are representative of 2 independent experiments, and at least 50 ubiquitin-positive bacteria were analyzed. (F) A proposed cartoon model illustrating the potential functional mode of the autophagy receptor CALCOCO2 in recognizing ubiquitin-coated pathogens in xenophagy.
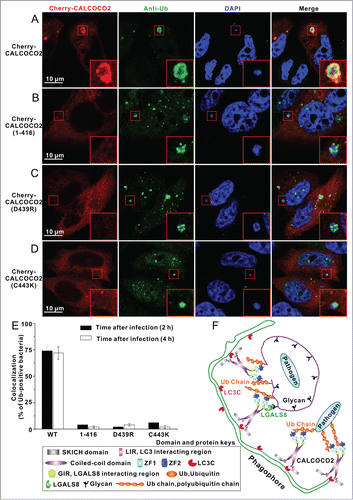
To further evaluate the ZF2-ubiquitin interaction in the cellular recruitment of CALCOCO2 to ubiquitin-coated invading S. typhimurium, we also used the D439R and C443K mutants of CALCOCO2, as mutations of these key interface residues essentially eliminated the ZF2-mono-ubiquitin interaction (; Fig. S9A and B). The overexpressed CALCOCO2 D439R or C443K mutants in S. typhimurium-infected cells were largely diffuse throughout the cytosol and their colocalization with ubiquitin-coated invading bacteria was dramatically compromised (), which indicated that the interaction between ZF2 and ubiquitin was critical for the cellular colocalization of CALCOCO2 and ubiquitin-coated invading S. typhimurium.
Discussion
In this work, we discovered that the C-terminal region of CALCOCO2 contains a dynamic zinc finger ZF1 and a canonical C2H2-type zinc finger ZF2. We also found that only the ZF2 is responsible for ubiquitin binding. An intriguing question is: what is the function of this dynamic ZF1 of CALCOCO2? In addition to binding ubiquitin, the C-terminal region of CALCOCO2 was also reported to interact with the unconventional myosin motor MYO6/myosin VI,Citation52 which is the only myosin motor moving toward the minus end of actin filaments and is implicated in many cellular processes including endocytosis and autophagy.Citation53–56 The interaction between MYO6 and CALCOCO2 facilitates autophagosome maturation and the subsequent fusion with a lysosome.Citation54,55,57 Interestingly, our NMR titration experiments using MYO6 to titrate 15N-labeled CALCOCO2 fragment (residues 365–446) revealed that both the ZF1 and ZF2 domains are directly involved in the binding to MYO6, and the ZF2 domain is the major binding site (Fig. S10A and B). Further titration of the 15N-labeled ZF2 domain with MYO6 showed that although the ZF2 domain alone could bind to MYO6 (Fig. S10C and D), its binding affinity to MYO6 was much weaker than that of the CALCOCO2 fragment (residues 365–446) containing both ZF1 and ZF2 domains as indicated by the different chemical shift changing patterns of these 2 CALCOCO2 proteins in the presence of MYO6 proteins (Fig. S10A and C). Therefore, ZF1 somehow could cooperate with ZF2 to interact with MYO6, and the 2 zinc finger domains of CALCOCO2 have distinct functions and play different roles in binding to ubiquitin and MYO6. However, the detailed mechanism of their interaction and the relationship between ubiquitin binding and MYO6 binding of CALCOCO2 remain to be elucidated.
Our systematic biochemical and structural characterization clearly indicated that CALCOCO2 is unlikely to have obvious selectivity in binding to K63-linked, K48-linked and M1-linked poly-ubiquitin chains (; Fig. S5). Given the fact that K48-linked and K63-linked poly-ubiquitination are also involved in other selective autophagy processes, such as aggrephagy and mitophagy,Citation8,19,22,58 it is puzzling why CALCOCO2 is only involved in xenophagy, although distinct spatial distributions of CALCOCO2 and other autophagic cargoes may partially explain the above dilemma. It is likely that the specific involvement of CALCOCO2 in xenophagy is also mediated by other regulatory binding partners, especially some CALCOCO2-associated upstream pattern-recognition or danger receptors, such as the E3 ubiquitin ligase LRSAM1 and the sugar receptor LGALS8. LRSAM1 functions as a bacterial recognition protein and is responsible for bacteria-associated ubiquitination in anti-Salmonella autophagy.Citation39 It can directly interact with the middle coiled-coil region of CALCOCO2, and its localization to bacteria occurs prior to the bacterial ubiquitin and CALCOCO2 colocalization.Citation39 As a danger receptor, LGALS8 can selectively recognize host glycans exposed on damaged bacteria-containing vacuoles and the GIR motif of CALCOCO2,Citation42-44 and it can transiently recruit CALCOCO2 to damaged bacterial vacuoles in anti-Salmonella autophagy.Citation42 Thus, we speculated that the initial recruitments of CALCOCO2 around bacterial targets mediated by LRSAM1 and LGALS8 rather than ubiquitin are more likely the crucial steps to determine the specificity of CALCOCO2 in xenophagy, whereas the persistent localization of CALCOCO2 to bacteria requires the subsequent ubiquitin-dependent recruitment. Using CALCOCO2 variants (the D439R and C443K mutants) that only lost the ability to interact with ubiquitin, we demonstrated that the specific interaction between CALCOCO2 and ubiquitin was essential for the persistent colocalization of CALCOCO2 with ubiquitin-coated invading S. typhimurium (). However, due to technological limitations, we were unable to trace the initial recruitment process of CALCOCO2 to the invading bacteria. Therefore, more work is necessary to uncover the detailed molecular mechanism governing the specific recognitions of intracellular ubiquitin-decorated pathogens by the autophagy receptor CALCOCO2.
Taking all the data presented in this study together, we propose a model depicting the CALCOCO2-mediated recognition process of ubiquitin-decorated invading pathogens for xenophagy (). In this model, different linkage types of ubiquitin chains including K48-linked, K63-linked and M1-linked poly-ubiquitin chains are found in the ubiquitin coats surrounding the invading pathogens or the broken pathogen-containing vacuoles. CALCOCO2 forms a dimer unit via its middle coiled-coil region and specifically recognizes these poly-ubiquitin chains through its ZF2 domain. We speculate that these poly-ubiquitin chains decorated on the invading pathogens or the broken vacuoles may function as a super scaffold to recruit and cluster plenty of CALCOCO2 together with subsequent autophagy machinery, thereby quickly amplifying the selective autophagy process to sequester the targeting pathogens for autophagic degradation.
In summary, we have systematically characterized the C-terminal cargo-binding region of CALCOCO2, and found this region contains 2 functionally distinct zinc fingers, the dynamic ZF1, and the canonical C2H2-type ZF2. The ZF2 of CALCOCO2 is responsible for ubiquitin-binding, and it can recognize mono-ubiquitin and different linkage types of poly-ubiquitin chains. The atomic structures of the ZF2 domain alone and in complex with mono-ubiquitin determined in this study elucidated the detailed molecular mechanism governing the specific recognition of ubiquitin by CALCOCO2. Finally, the data presented in this work also provide new mechanistic insight into how the ubiquitin-binding autophagy receptor CALCOCO2 recognizes and targets ubiquitin-coated cargoes for selective autophagy.
Materials and Methods
Protein expression and purification
The different DNA fragments encoding human CALCOCO2 (residues 1–446, 1–416, 141–446, 365–446, 414–446) were PCR amplified from the full-length human CALCOCO2 cDNA. Three fragments (residues 1–446, 1–416, 141–446) were cloned into pET-32M vectors (a modified version of the pET32a vector [Novagen, 69015–3] containing a N-terminal thioredoxin-tag and His6-tag), while the other 2 fragments were cloned into pET-MG vectors (a modified version of pET32a vector containing a N-terminal GB1-tag and His6-tag). The fragments (residues 365–446, 414–446) were also cloned into a pGEX6P-2 vector (GE Healthcare, 27–4598–01), and a pGEX-GB vector (a modified version of the pGEX6P-2 vector containing a N-terminal GST-tag and GB1-tag), respectively. The coding sequence of human ubiquitin (residues 1–76) was PCR amplified from the full-length human UBA52, and cloned into a pET-M vector (a modified version of pET32a vector containing a N-terminal His6-tag) or a pGEX4T-1 vector (GE Healthcare, 27–4580–01). All point mutations of CALCOCO2 and ubiquitin used in this study were created using the standard PCR-based mutagenesis method, further checked by Taq Master mix (Vazyme Biotech Co., P112–01) and confirmed by DNA sequencing.
Recombinant proteins were expressed in BL21 (DE3) E. coli cells at 16°C. The bacterial cells were lysed by the ultrahigh-pressure homogenizer FB-110XNANO homogenizer machine (Shanghai Litu Machinery Equipment Engineering Co., Shanghai, China). Then the lysate was spun down by centrifugation at 18000 rpm (39191 g) for 30 min to remove the pellet fractions. His6-tagged proteins were purified by Ni2+-NTA agarose (GE Healthcare, 17–5318–03) affinity chromatography, while GST-tagged proteins were purified by glutathione sepharose 4B (GE Healthcare, 17–0756–04) affinity chromatography. Each recombinant protein was further purified by size-exclusion chromatography. The terminal tag of each recombinant protein was cleaved by 3C protease (purified in-house) and further removed by size-exclusion chromatography. Uniformly 15N or 15N, 13C-labeled CALCOCO2 proteins were prepared by growing bacteria in M9 minimal mediumCitation59 using 15NH4Cl (Cambridge Isotope Laboratories Inc., NLM-467) as the sole nitrogen source or 15NH4Cl and 13C6-glucose (Cambridge Isotope Laboratories Inc., CLM-1396) as the sole nitrogen and carbon sources, respectively.
Preparation of K48-linked, K63-linked, and M1-linked di-ubiquitins and M1-linked tetra-ubiquitin
The K48R-Ub, K63R-Ub, and Ub-D77 mono-ubiquitin mutants were cloned into the pET-M-3C vector (a modified version of the pET32a vector [Novagen, 69015–3] containing a N-terminal His6-tag), and expressed and purified as mono-ubiquitin for enzymatic synthesis of K48-linked di-ubiquitin or K63-linked di-ubiquitin according to published protocols.Citation60,61 The final K48-linked di-ubiquitin and K63-linked di-ubiquitin proteins were obtained by further removing the C-terminal D77 residue using the purified Ucl3 enzyme.Citation62 For M1-linked di-ubiquitin and M1-linked tetra-ubiquitin, 2 or 4 repeats of a DNA fragment encoding the human ubiquitin (residues 1–76) were directly fused together as one extended DNA fragment, which was cloned into the pET-M-3C or pET-32M-3C (a modified version of the pET32a vector [Novagen, 69015–3]) vectors. The M1-linked di-ubiquitin and tetra-ubiquitin proteins were expressed and purified following the same procedure for mono-ubiquitin.
Elemental analysis
Three GB1-tagged CALCOCO2 fragments (residues 365–446, 414–446 and 365–416) purified using Ni2+-NTA agarose affinity chromatography were concentrated to ∼0.56 mM for elemental analysis in 20 mM Tris-HCl, pH 7.5, 100 mM NaCl, 1 mM DTT. The metal elements contained in each sample were qualitatively analyzed by Inductively Coupled Plasma Optical Emission Spectrometry (ICP-OES),Citation63 and further quantitative measurements of the zinc element in each sample were conducted by atomic absorption spectroscopy.Citation63
NMR spectroscopy
The protein samples for NMR studies were concentrated to ∼0.1 mM for titration experiments and ∼1.0 mM for structural determinations in 50 mM potassium phosphate buffer, pH 6.5, 50 mM NaCl, 1 mM DTT. NMR spectra were acquired at 25°C on an Agilent 800 MHz spectrometer at the Shanghai Institute of Organic Chemistry. Backbone and side-chain resonance assignments were achieved by combination of the standard heteronuclear correlation experiments and 2D 1H-NOESY experiments.Citation64,65 Approximate inter-proton distance restraints were derived from 2D 1H-NOESY, 3D 15N-, and 13C-seperated NOESY spectra. Structures were calculated using the program CNS.Citation66 The figures were prepared using the programs PyMOL (http://pymol.sourceforge.net/), and MOLMOL.Citation67
Crystallography
Freshly purified CALCOCO2 ZF2 domain and mono-ubiquitin were mixed at a molar ratio of 1:1, the ZF2-mono-ubiquitin complex was further purified by size-exclusion chromatography. Crystals of CALCOCO2 ZF2 in complex with the mono-ubiquitin were obtained by mixing the complex protein (30 mg/ml in 20 mM Tris–HCl, pH 7.5, 100 mM NaCl, 1 mM DTT) with equal volumes of reservoir solution containing 0.2 M magnesium acetate tetrahydrate, 20% w/v polyethylene glycol 3350 (HAMPTON RESEARCH CORP., HR2–591) using the sitting-drop, vapor-diffusion methodCitation68 for one wk at 16°C. Crystals were frozen using the above reservoir solution with 10% glycerol added as a cryoprotectant. A 2.1-Å resolution X-ray data set was collected at the beamline BL17U1 of the Shanghai Synchrotron Radiation Facility. The diffraction data were processed using the MOSFLMCitation69 and scaled by the SCALA modules in the CCP4 suite.Citation70
The phase problem of the complex was solved by the molecular replacement method using the NMR structures of CALCOCO2 ZF2 solved in this study and a crystal structure of mono-ubiquitin (PDB id: 1UBQ) as the search models with PHASER.Citation70 The initial model was rebuilt manually and then refined using REFMACCitation71 against the 2.1 Å resolution data set. Further manual model building and adjustment were completed using COOT.Citation72 The qualities of the final model were validated by MolProbity.Citation73 The final refinement statistics are listed in Table S3.
Analytical gel filtration chromatography
Analytical gel filtration chromatography was carried out on an AKTA FPLC system (GE Healthcare). Proteins were loaded onto a Superose 12 10/300 GL column (GE Healthcare, 17–5173–01) equilibrated with a buffer containing 20 mM Tris-HCl, pH 7.5, 100 mM NaCl, 1 mM DTT.
GST affinity isolation assay
Direct interactions between different CALCOCO2 and ubiquitin proteins were assayed in phosphate-buffered saline (PBS; MesGen Biotech, MG3150), pH 7.4. 50 ug of GST-tagged proteins and null-tagged proteins were mixed at a molar ratio of 1:4 in 1 ml of the assay buffer. The GST-CALCOCO2/ubiquitin complexes were pelleted by adding 30 µl of fresh glutathione sepharose 4B beads (GE Healthcare, 17–0756–04). The pellet fractions were washed 6 times with 1 ml of the assay buffer and subsequently boiled with 30 µl of 2× SDS-PAGE sample buffer. The precipitated proteins in the gel were visualized by Coomassie Blue staining.
Isothermal titration calorimetry assay
ITC measurements were carried out on an ITC200 calorimeter (GE Healthcare) at 25°C. All protein samples were in the same buffer containing 50 mM Tris, pH 7.5, 100 mM NaCl, 1 mM DTT. The concentrated 40 μM CALCOCO2 fragments and 400 μM of mono-ubiquitin or ubiquitin chain proteins were loaded into the cell and the syringe, respectively. The titration processes were performed by injecting 40 μl aliquots of the ubiquitin or ubiquitin chain proteins into CALCOCO2 fragments at time intervals of 2 min to ensure that the titration peak returned to the baseline. The titration data were analyzed using the program Origin7.0 from Micro Cal and fitted using the one-site binding model.
Cell culture, transfection, S. typhimurium infection, and fluorescence imaging
HEK293T or HeLa cells were cultured in Dulbecco's modified Eagle's medium (Invitrogen, 11995–065) supplemented with 10% fetal bovine serum (Invitrogen, 10099–141). Transfections of mCherry-CALCOCO2, GFP-ubiquitin or mutant expressing plasmids were performed with Lipofectamine 2000 (Invitrogen, 11668–019) according to the manufacturer's instructions, following 24-h culture in Dulbecco's modified Eagle's medium supplemented with 10% fetal bovine serum.
HeLa cells transfected with plasmids encoding mCherry-CALCOCO2 or its mutants were infected with S. typhimurium (strain CMCC50115) as previously described.Citation37 Briefly, S. typhimurium was grown overnight in LB medium and subcultured (1: 33) for 3.5 h before infection. HeLa cells plated in 12-well plates were infected with 40 μl of such bacterial-containing cultures for 30 min. After 2 PBS washes and a further incubation with 100 μg/ml gentamycin (Sangon, G0304–10g) for 1 h, cells were cultured in 20 μg/ml gentamicin for 2 h or 4 h before fixing with 4% (wt/vol) paraformaldehyde and quenching with PBS buffer containing 1 M glycine, 0.1% (vol/vol) Triton X-100 (Sangon, T0694–500ml). Cells were blocked in PBTB (PBS, 0.1% [vol/vol] Triton X-100, 3% [wt/vol] BSA [Bovogen, BSAS0.1]). Antibodies were applied in PBTB for 1 h. The cell images were captured using the TCS SP5 confocal microscope equipped with LES AF Lite software (Leica, Inc., Thornwood, NY, USA). At least 50 events per slide were scored in quantitative assays.
Co-immunoprecipitation
For co-immunoprecipitation, transfected HEK293T cells were lysed in ice-cold cell lysis buffer (50 mM Tris, pH 7.5, 50 mM NaCl, 0.5% Nonidet P-40 [Sangon, A100109–0500], 5% glycerol, 1 mM phenylmethylsulfonyl fluoride [Sangon, P0754–25g], 1% protease inhibitor cocktail [Ameresco, m221–1mL]) for 1 h at 4°C, and followed by centrifugation at 12000g for 15 min at 4°C. The supernatant fraction was then incubated with anti-GFP conjugated agarose beads (clone RQ2; Medical & Biological Laboratories, m20004-M) for 4 h at 4°C. The beads were washed with the cell lysis buffer and resuspended with SDS-PAGE sample buffer. The prepared samples were separated by 10% SDS-PAGE and analyzed using western blot.
Coordinates
The atomic coordinates of the CALCOCO2 ZF2 domain alone and ZF2-mono-ubiquitin complex have been deposited in the Protein Data Bank under the accession codes 2MXP and 4XKL, respectively.
Disclosure of Potential Conflicts of Interest
No potential conflicts of interest were disclosed.
Supplemental Material
Supplemental data for this article can be accessed on the publisher's website.
1082025_Supplemental_Content.pdf
Download PDF (11.6 MB)Acknowledgments
We thank SSRF BL17U and NCPSS BL19U1 for X-ray beam time, Dr. Shang Yuan and Jianchao Li for help in the X-ray diffraction data collection, Dr. Cong Liu for providing the ITC machine, Dr. Wenyu Wen for the help in the confocal microscopy imaging, Dr. Ronggui Hu for the E1 UBA1 plasmid, Prof. Jiahuai Han for the full length CALCOCO2, E2 UBE2N and UBE2V1 cDNA, and Prof. Mingjie Zhang for the critical reading of the manuscript.
Funding
This work was supported by grants from the National Natural Science Foundation of China 31470749, National Basic Research Program of China 2013CB836900, a “Thousand Talents Program” young investigator award, a Shanghai Rising Star Scholar award 13QA1404300, the start-up fund from State Key Laboratory of Bioorganic and Natural Products Chemistry and Chinese Academy of Sciences (to LP), and a China Postdoctoral Science Foundation 2014M561538 (to XX).
References
- Klionsky DJ, Emr SD. Cell biology - Autophagy as a regulated pathway of cellular degradation. Science 2000; 290:1717-21; PMID:11099404; http://dx.doi.org/10.1126/science.290.5497.1717
- Levine B, Mizushima N, Virgin HW. Autophagy in immunity and inflammation. Nature 2011; 469:323-35; PMID:21248839; http://dx.doi.org/10.1038/nature09782
- Kroemer G, Marino G, Levine B. Autophagy and the integrated stress response. Molecular Cell 2010; 40:280-93; PMID:20965422; http://dx.doi.org/10.1016/j.molcel.2010.09.023
- Nakatogawa H, Suzuki K, Kamada Y, Ohsumi Y. Dynamics and diversity in autophagy mechanisms: lessons from yeast. Nat Rev Mol Cell Biology 2009; 10:458-67; PMID:19491929; http://dx.doi.org/10.1038/nrm2708
- Yang Z, Klionsky DJ. Eaten alive: a history of macroautophagy. Nat Cell Biol 2010; 12:814-22; PMID:20811353; http://dx.doi.org/10.1038/ncb0910-814
- Feng YC, He D, Yao ZY, Klionsky DJ. The machinery of macroautophagy. Cell Res 2014; 24:24-41; PMID:24366339; http://dx.doi.org/10.1038/cr.2013.168
- Stolz A, Ernst A, Dikic I. Cargo recognition and trafficking in selective autophagy. Nat Cell Biol 2014; 16:495-501; PMID:24875736; http://dx.doi.org/10.1038/ncb2979
- Randow F, Youle RJ. Self and nonself: How autophagy targets mitochondria and bacteria. Cell Host Micro 2014; 15:404-12; PMID:24721569; http://dx.doi.org/10.1016/j.chom.2014.03.012
- Green DR, Levine B. To be or not to be? How selective autophagy and cell death govern cell fate. Cell 2014; 157:65-75; PMID:24679527; http://dx.doi.org/10.1016/j.cell.2014.02.049
- Johansen T, Lamark T. Selective autophagy mediated by autophagic adapter proteins. Autophagy 2011; 7:279-96; PMID:21189453; http://dx.doi.org/10.4161/auto.7.3.14487
- Geng J, Klionsky DJ. The Atg8 and Atg12 ubiquitin-like conjugation systems in macroautophagy. ‘Protein modifications: beyond the usual suspects’ review series. EMBO Rep 2008; 9:859-64; PMID:18704115; http://dx.doi.org/10.1038/embor.2008.163
- Liu L, Feng D, Chen G, Chen M, Zheng Q, Song P, Ma Q, Zhu C, Wang R, Qi W, et al. Mitochondrial outer-membrane protein FUNDC1 mediates hypoxia-induced mitophagy in mammalian cells. Nat Cell Biol 2012; 14:177-85; PMID:22267086; http://dx.doi.org/10.1038/ncb2422
- Rogov V, Dotsch V, Johansen T, Kirkin V. Interactions between autophagy receptors and ubiquitin-like proteins form the molecular basis for selective autophagy. Mol Cell 2014; 53:167-178; PMID:24462201; http://dx.doi.org/10.1016/j.molcel.2013.12.014
- Jiang S, Wells CD, Roach PJ. Starch-binding domain-containing protein 1 (Stbd1) and glycogen metabolism: Identification of the Atg8 family interacting motif (AIM) in Stbd1 required for interaction with GABARAPL1. Biochem Biophys Res Commun 2011; 413:420-5; PMID:21893048; http://dx.doi.org/10.1016/j.bbrc.2011.08.106
- Behrends C, Fulda S. Receptor proteins in selective autophagy. Int J Cell Biol 2012; 2012:673290; PMID:22536250; http://dx.doi.org/10.1155/2012/673290
- Lu K, Psakhye I, Jentsch S. Autophagic clearance of polyQ proteins mediated by ubiquitin-Atg8 adaptors of the conserved CUET protein family. Cell 2014; 158:549-63; PMID:25042851; http://dx.doi.org/10.1016/j.cell.2014.05.048
- Ichimura Y, Kirisako T, Takao T, Satomi Y, Shimonishi Y, Ishihara N, Mizushima N, Tanida I, Kominami E, Ohsumi M, et al. A ubiquitin-like system mediates protein lipidation. Nature 2000; 408:488-92; PMID:11100732; http://dx.doi.org/10.1038/35044114
- Ding WX, Yin XM. Mitophagy: mechanisms, pathophysiological roles, and analysis. Biol Chem 2012; 393:547-64; PMID:22944659
- Lamark T, Johansen T. Aggrephagy: selective disposal of protein aggregates by macroautophagy. Int J Cell Biol 2012; 2012:736905; PMID:22518139; http://dx.doi.org/10.1155/2012/736905
- Mancias JD, Wang XX, Gygi SP, Harper JW, Kimmelman AC. Quantitative proteomics identifies NCOA4 as the cargo receptor mediating ferritinophagy. Nature 2014; 509:105-+; PMID:24695223; http://dx.doi.org/10.1038/nature13148
- Gomes LC, Dikic I. Autophagy in antimicrobial immunity. Mol Cell 2014; 54:224-33; PMID:24766886; http://dx.doi.org/10.1016/j.molcel.2014.03.009
- Kirkin V, McEwan DG, Novak I, Dikic I. A role for ubiquitin in selective autophagy. Mol Cell 2009; 34:259-69; PMID:19450525; http://dx.doi.org/10.1016/j.molcel.2009.04.026
- Shaid S, Brandts CH, Serve H, Dikic I. Ubiquitination and selective autophagy. Cell Death Differ 2013; 20:21-30; PMID:22722335; http://dx.doi.org/10.1038/cdd.2012.72
- Randow F. How cells deploy ubiquitin and autophagy to defend their cytosol from bacterial invasion. Autophagy 2011; 7:304-9; PMID:21193841; http://dx.doi.org/10.4161/auto.7.3.14539
- Dikic I, Wakatsuki S, Walters KJ. Ubiquitin-binding domains - from structures to functions. Nat Rev Mol Cell Biol 2009; 10:659-71; PMID:19773779; http://dx.doi.org/10.1038/nrm2767
- Ikeda F, Dikic I. Atypical ubiquitin chains: new molecular signals. ‘Protein Modifications: Beyond the Usual Suspects’ review series. EMBO Rep 2008; 9:536-42; PMID:18516089; http://dx.doi.org/10.1038/embor.2008.93
- Hicke L, Schubert HL, Hill CP. Ubiquitin-binding domains. Nat Rev Mol Cell Biol 2005; 6:610-21; PMID:16064137; http://dx.doi.org/10.1038/nrm1701
- Searle MS, Garner TP, Strachan J, Long J, Adlington J, Cavey JR, Shaw B, Layfield R. Structural insights into specificity and diversity in mechanisms of ubiquitin recognition by ubiquitin-binding domains. Biochem Soc Trans 2012; 40:404-8; PMID:22435820; http://dx.doi.org/10.1042/BST20110729
- van Wijk SJ, Fiskin E, Putyrski M, Pampaloni F, Hou J, Wild P, Kensche T, Grecco HE, Bastiaens P, Dikic I. Fluorescence-based sensors to monitor localization and functions of linear and K63-linked ubiquitin chains in cells. Mol Cell 2012; 47:797-809; PMID:22819327; http://dx.doi.org/10.1016/j.molcel.2012.06.017
- Watson RO, Manzanillo PS, Cox JS. Extracellular M. tuberculosis DNA targets bacteria for autophagy by activating the host DNA-sensing pathway. Cell 2012; 150:803-15; PMID:22901810; http://dx.doi.org/10.1016/j.cell.2012.06.040
- Manzanillo PS, Ayres JS, Watson RO, Collins AC, Souza G, Rae CS, Schneider DS, Nakamura K, Shiloh MU, Cox JS. The ubiquitin ligase parkin mediates resistance to intracellular pathogens. Nature 2013; 501:512-6; PMID:24005326; http://dx.doi.org/10.1038/nature12566
- Ivanov S, Roy CR. NDP52: the missing link between ubiquitinated bacteria and autophagy. Nat Immunol 2009; 10:1137-9; PMID:19841643; http://dx.doi.org/10.1038/ni1109-1137
- Cemma M, Kim PK, Brumell JH. The ubiquitin-binding adaptor proteins p62/SQSTM1 and NDP52 are recruited independently to bacteria-associated microdomains to target Salmonella to the autophagy pathway. Autophagy 2011; 7:341-5; PMID:21079414; http://dx.doi.org/10.4161/auto.7.3.14046
- Mostowy S, Sancho-Shimizu V, Hamon MA, Simeone R, Brosch R, Johansen T, Cossart P. p62 and NDP52 proteins target intracytosolic Shigella and Listeria to different autophagy pathways. J Biol Chem 2011; 286:26987-95; PMID:21646350; http://dx.doi.org/10.1074/jbc.M111.223610
- Wild P, Farhan H, McEwan DG, Wagner S, Rogov VV, Brady NR, Richter B, Korac J, Waidmann O, Choudhary C, et al. Phosphorylation of the autophagy receptor optineurin restricts Salmonella growth. Science 2011; 333:228-33; PMID:21617041; http://dx.doi.org/10.1126/science.1205405
- Zheng YT, Shahnazari S, Brech A, Lamark T, Johansen T, Brumell JH. The adaptor protein p62/SQSTM1 targets invading bacteria to the autophagy pathway. J Immunol 2009; 183:5909-16; PMID:19812211; http://dx.doi.org/10.4049/jimmunol.0900441
- Thurston TL, Ryzhakov G, Bloor S, von Muhlinen N, Randow F. The TBK1 adaptor and autophagy receptor NDP52 restricts the proliferation of ubiquitin-coated bacteria. Nat Immunol 2009; 10:1215-21; PMID:19820708; http://dx.doi.org/10.1038/ni.1800
- Shpilka T, Elazar Z. Essential role for the mammalian ATG8 isoform LC3C in xenophagy. Mol Cell 2012; 48:325-6; PMID:23141200; http://dx.doi.org/10.1016/j.molcel.2012.10.020
- Huett A, Heath RJ, Begun J, Sassi SO, Baxt LA, Vyas JM, Goldberg MB, Xavier RJ. The LRR and RING domain protein LRSAM1 is an E3 ligase crucial for ubiquitin-dependent autophagy of intracellular Salmonella Typhimurium. Cell Host Microbe 2012; 12:778-90; PMID:23245322; http://dx.doi.org/10.1016/j.chom.2012.10.019
- Ellinghaus D, Zhang H, Zeissig S, Lipinski S, Till A, Jiang T, Stade B, Bromberg Y, Ellinghaus E, Keller A, et al. Association between variants of PRDM1 and NDP52 and Crohn's disease, based on exome sequencing and functional studies. Gastroenterology 2013; 145:339-47; PMID:23624108; http://dx.doi.org/10.1053/j.gastro.2013.04.040
- Till A, Lipinski S, Ellinghaus D, Mayr G, Subramani S, Rosenstiel P, Franke A. Autophagy receptor CALCOCO2/NDP52 takes center stage in Crohn disease. Autophagy 2013; 9:1256-7; PMID:23820297; http://dx.doi.org/10.4161/auto.25483
- Thurston TL, Wandel MP, von Muhlinen N, Foeglein A, Randow F. Galectin 8 targets damaged vesicles for autophagy to defend cells against bacterial invasion. Nature 2012; 482:414-8; PMID:22246324; http://dx.doi.org/10.1038/nature10744
- Li S, Wandel MP, Li F, Liu Z, He C, Wu J, Shi Y, Randow F. Sterical hindrance promotes selectivity of the autophagy cargo receptor NDP52 for the danger receptor galectin-8 in antibacterial autophagy. Sci Signal 2013; 6:ra9; PMID:23386746
- Kim BW, Hong SB, Kim JH, Kwon do H, Song HK. Structural basis for recognition of autophagic receptor NDP52 by the sugar receptor galectin-8. Nat Commun 2013; 4:1613; PMID:23511477; http://dx.doi.org/10.1038/ncomms2606
- Long J, Gallagher TR, Cavey JR, Sheppard PW, Ralston SH, Layfield R, Searle MS. Ubiquitin recognition by the ubiquitin-associated domain of p62 involves a novel conformational switch. J Biol Chem 2008; 283:5427-40; PMID:18083707; http://dx.doi.org/10.1074/jbc.M704973200
- Korioth F, Gieffers C, Maul GG, Frey J. Molecular characterization of NDP52, a novel protein of the nuclear domain 10, which is redistributed upon virus infection and interferon treatment. J Cell Biol 1995; 130:1-13; PMID:7540613; http://dx.doi.org/10.1083/jcb.130.1.1
- Bach I. The LIM domain: regulation by association. Mech Dev 2000; 91:5-17; PMID:10704826; http://dx.doi.org/10.1016/S0925-4773(99)00314-7
- Penengo L, Mapelli M, Murachelli AG, Confalonieri S, Magri L, Musacchio A, Di Fiore PP, Polo S, Schneider TR. Crystal structure of the ubiquitin binding domains of rabex-5 reveals two modes of interaction with ubiquitin. Cell 2006; 124:1183-95; PMID:16499958; http://dx.doi.org/10.1016/j.cell.2006.02.020
- Lee S, Tsai YC, Mattera R, Smith WJ, Kostelansky MS, Weissman AM, Bonifacino JS, Hurley JH. Structural basis for ubiquitin recognition and autoubiquitination by Rabex-5. Nat Struct Mol Biol 2006; 13:264-71; PMID:16462746; http://dx.doi.org/10.1038/nsmb1064
- Bomar MG, Pai MT, Tzeng SR, Li SSC, Zhou P. Structure of the ubiquitin-binding zinc finger domain of human DNA Y-polymerase eta. Embo Rep 2007; 8:247-251; PMID:17304240; http://dx.doi.org/10.1038/sj.embor.7400901
- von Muhlinen N, Akutsu M, Ravenhill BJ, Foeglein A, Bloor S, Rutherford TJ, Freund SM, Komander D, Randow F. LC3C, bound selectively by a noncanonical LIR motif in NDP52, is required for antibacterial autophagy. Mol Cell 2012; 48:329-42; PMID:23022382; http://dx.doi.org/10.1016/j.molcel.2012.08.024
- Morriswood B, Ryzhakov G, Puri C, Arden SD, Roberts R, Dendrou C, Kendrick-Jones J, Buss F. T6BP and NDP52 are myosin VI binding partners with potential roles in cytokine signalling and cell adhesion. J Cell Sci 2007; 120:2574-85; PMID:17635994; http://dx.doi.org/10.1242/jcs.007005
- Wells AL, Lin AW, Chen LQ, Safer D, Cain SM, Hasson T, Carragher BI, Milligan RA, Sweeney HL. Myosin VI is an actin-based motor that moves backwards. Nature 1999; 401:505-508; PMID:10519557; http://dx.doi.org/10.1038/46835
- Tumbarello DA, Waxse BJ, Arden SD, Bright NA, Kendrick-Jones J, Buss F. Autophagy receptors link myosin VI to autophagosomes to mediate Tom1-dependent autophagosome maturation and fusion with the lysosome. Nat Cell Biol 2012; 14:1024-35; PMID:23023224; http://dx.doi.org/10.1038/ncb2589
- Tumbarello DA, Kendrick-Jones J, Buss F. Myosin VI and its cargo adaptors - linking endocytosis and autophagy. J Cell Sci 2013; 126:2561-70; PMID:23781020; http://dx.doi.org/10.1242/jcs.095554
- Yu C, Feng W, Wei Z, Miyanoiri Y, Wen W, Zhao Y, Zhang M. Myosin VI undergoes cargo-mediated dimerization. Cell 2009; 138:537-48; PMID:19665975; http://dx.doi.org/10.1016/j.cell.2009.05.030
- Verlhac P, Gregoire IP, Azocar O, Petkova DS, Baguet J, Viret C, Faure M. Autophagy Receptor NDP52 Regulates Pathogen-Containing Autophagosome Maturation. Cell Host Microbe 2015; 17:515-25; PMID:25771791; http://dx.doi.org/10.1016/j.chom.2015.02.008
- Geisler S, Holmstrom KM, Skujat D, Fiesel FC, Rothfuss OC, Kahle PJ, Springer W. PINK1/Parkin-mediated mitophagy is dependent on VDAC1 and p62/SQSTM1. Nat Cell Biol 2010; 12:119-U70; PMID:20098416; http://dx.doi.org/10.1038/ncb2012
- Joseph S, David WR. Molecular cloning, a laboratory manual. 3rd ed. New York: Cold Spring Harbor Laboratory Press; 2001. 2344 p.
- Hofmann RM, Pickart CM. In vitro assembly and recognition of Lys-63 polyubiquitin chains. J Biol Chem 2001; 276:27936-43; PMID:11369780; http://dx.doi.org/10.1074/jbc.M103378200
- Piotrowski J, Beal R, Hoffman L, Wilkinson KD, Cohen RE, Pickart CM. Inhibition of the 26 S proteasome by polyubiquitin chains synthesized to have defined lengths. J Biol Chem 1997; 272:23712-23721; PMID:9295315; http://dx.doi.org/10.1074/jbc.272.38.23712
- Larsen CN, Krantz BA, Wilkinson KD. Substrate specificity of deubiquitinating enzymes: Ubiquitin C-terminal hydrolases. Biochemistry 1998; 37:3358-68; PMID:9521656; http://dx.doi.org/10.1021/bi972274d
- Ma RL, McLeod CW, Tomlinson K, Poole RK. Speciation of protein-bound trace elements by gel electrophoresis and atomic spectrometry. Electrophoresis 2004; 25:2469-2477; PMID:15300764; http://dx.doi.org/10.1002/elps.200405999
- Bax A, Grzesiek S. Methodological advances in rotein Nmr. Accoun Chem Res 1993; 26:131-138; http://dx.doi.org/10.1021/ar00028a001
- Wuthrich K. NMR of proteins and nucleic acids. 1st ed. New York: Wiley-Interscience; 1986. 292 p.
- Brunger AT, Adams PD, Clore GM, DeLano WL, Gros P, Grosse-Kunstleve RW, Jiang JS, Kuszewski J, Nilges M, Pannu NS, et al. Crystallography & NMR system: A new software suite for macromolecular structure determination. Acta Crystallogr D Biol Crystallogr 1998; 54:905-921; PMID:9757107; http://dx.doi.org/10.1107/S0907444998003254
- Koradi R, Billeter M, Wuthrich K. MOLMOL: A program for display and analysis of macromolecular structures. J Mol Graph 1996; 14:51-&; PMID:8744573; http://dx.doi.org/10.1016/0263-7855(96)00009-4
- Rhodes G. Crystallography made crystal clear: A guide for users of macromolecular models. Burlington: Academic Press; 2006. 352 p.
- Leslie AGW. Recent changes to the MOSFLM package for processing film and image plate data. Joint CCP4 + ESF-EAMCB Newsletter on Protein Crystallography 1992; No26
- Storoni LC, McCoy AJ, Read RJ. Likelihood-enhanced fast rotation functions. Acta Crystallogr D Biol Crystallogr 2004; 60:432-8; PMID:14993666; http://dx.doi.org/10.1107/S0907444903028956
- Murshudov GN, Vagin AA, Dodson EJ. Refinement of macromolecular structures by the maximum-likelihood method. Acta Crystallogr D Biol Crystallogr 1997; 53:240-55; PMID:15299926; http://dx.doi.org/10.1107/S0907444996012255
- Emsley P, Cowtan K. Coot: model-building tools for molecular graphics. Acta Crystallogr D Biol Crystallogr 2004; 60:2126-32; PMID:15572765; http://dx.doi.org/10.1107/S0907444904019158
- Davis IW, Leaver-Fay A, Chen VB, Block JN, Kapral GJ, Wang X, Murray LW, Arendall WB, Snoeyink J, Richardson JS, et al. MolProbity: all-atom contacts and structure validation for proteins and nucleic acids. Nucleic Acids Res 2007; 35:W375-83; PMID:17452350; http://dx.doi.org/10.1093/nar/gkm216