ABSTRACT
Hydrolysis within the vacuole in yeast and the lysosome in mammals is required for the degradation and recycling of a multitude of substrates, many of which are delivered to the vacuole/lysosome by autophagy. In humans, defects in lysosomal hydrolysis and efflux can have devastating consequences, and contribute to a class of diseases referred to as lysosomal storage disorders. Despite the importance of these processes, many of the proteins and regulatory mechanisms involved in hydrolysis and efflux are poorly understood. In this review, we describe our current knowledge of the vacuolar/lysosomal degradation and efflux of a vast array of substrates, focusing primarily on what is known in the yeast Saccharomyces cerevisiae. We also highlight many unanswered questions, the answers to which may lead to new advances in the treatment of lysosomal storage disorders.
Abbreviations: Ams1: α-mannosidase; Ape1: aminopeptidase I; Ape3: aminopeptidase Y; Ape4: aspartyl aminopeptidase; Atg: autophagy related; Cps1: carboxypeptidase S; CTNS: cystinosin, lysosomal cystine transporter; CTSA: cathepsin A; CTSD: cathepsin D; Cvt: cytoplasm-to-vacuole targeting; Dap2: dipeptidyl aminopeptidase B; GS-bimane: glutathione-S-bimane; GSH: glutathione; LDs: lipid droplets; MVB: multivesicular body; PAS: phagophore assembly site; Pep4: proteinase A; PolyP: polyphosphate; Prb1: proteinase B; Prc1: carboxypeptidase Y; V-ATPase: vacuolar-type proton-translocating ATPase; VTC: vacuolar transporter chaperone
Introduction
The yeast vacuole (lysosome in humans) is a key center for metal ion homeostasis, nutrient storage, and cellular detoxification [Citation1]. It is perhaps best-known for its function as a degradative organelle; in yeast, the vacuole accounts for approximately 40% of protein degradation during growing conditions, which increases to 85% when cells are starved of nutrients [Citation2]. Substrates destined for the vacuole can be delivered there by a variety of trafficking mechanisms including the vacuolar protein sorting pathway, endocytosis, the cytoplasm-to-vacuole targeting (Cvt) pathway, and direct transport across the vacuole membrane [Citation1,Citation3]. Another major trafficking pathway whereby proteins and other substrates are delivered to the vacuole during stress conditions is autophagy [Citation4].
In the yeast Saccharomyces cerevisiae, there are 2 primary types of autophagy, selective and nonselective. Either of these processes can occur through microautophagy or macroautophagy (). Microautophagy involves internalization of cargo into the vacuolar lumen through invagination or protrusion of the vacuole limiting membrane. The vacuole membrane surrounding engulfed cargo scissions, generating a single-membrane vesicle within the lumen; the resultant intralumenal vesicles are subsequently lysed, and the cargo is degraded [Citation4,Citation5].
Figure 1. Macro- and microautophagy in S. cerevisiae. Macroautophagy consists of induction and nucleation at the phagophore assembly site (PAS) followed by elongation and closure of the phagophore membrane around cellular cargo to form a completed autophagosome. The outer membrane of the autophagosome fuses with the vacuole membrane, releasing a single-membrane autophagic body into the vacuole lumen. Microautophagy occurs via direct invagination of the vacuole membrane and scission to release a vesicle into the vacuole lumen. In both types of autophagy, resident vacuolar hydrolases degrade the autophagic body and its contents, which are then exported back into the cytoplasm for reuse by the cell. See text for more detail.
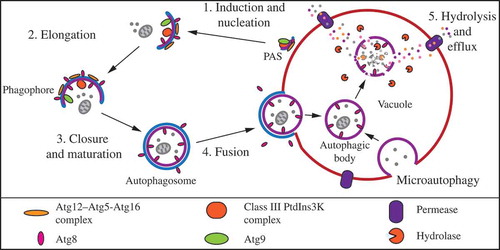
Macroautophagy, the more extensively studied form of autophagy, is characterized by de novo generation of a double-membrane structure that encapsulates cargo away from the vacuole. Under conditions of nutrient starvation, macroautophagy, hereafter autophagy, is induced at the perivacuolar phagophore assembly site (PAS) by the Atg1 kinase complex [Citation6,Citation7]. Following induction, nucleation and membrane expansion lead to formation of a transient double-membrane phagophore that forms de novo and gradually expands to surround cargo. These processes involve the transmembrane protein Atg9 and phosphatidylinositol 3-kinase (PtdIns3K) complex I, as well as 2 ubiquitin-like (Ubl) conjugation systems that include the Ubl proteins Atg8 and Atg12 [Citation4,Citation5]. Eventually, the ends of the expanding phagophore join to form a completed double-membrane autophagosome. The mature autophagosome travels to the vacuole, where the outer membrane of the autophagosome fuses with the limiting membrane of the vacuole, releasing the inner autophagosome vesicle along with its cargo into the vacuolar lumen; the single-membrane autophagosomal compartment is now termed an autophagic body. The autophagic body is subsequently lysed and the contents are broken down by the various hydrolases within the vacuole. The resulting macromolecules are then transported into the cytosol for reuse [Citation5].
The vast majority of autophagy research has focused on induction, regulation, membrane recruitment and autophagosome formation, cargo recognition, and autophagosome-vacuole fusion, whereas the intravacuolar steps of substrate degradation and efflux of the breakdown products have been largely neglected. Although generally glossed over in discussions of autophagic processes, these terminal events are critically important for completion of autophagy and maintenance of cellular health. In humans, there is an entire class of more than 50 diseases, the lysosomal storage disorders, which involve accumulation of different substrates in the lysosome resulting from defects in lysosomal hydrolases, hydrolase activators, and vacuolar transporters [Citation8,Citation9]. Altogether, lysosomal storage disorders occur in approximately 1/8000 live births and vary widely with regard to age of onset, severity, substrates accumulated, and organ systems affected [Citation10,Citation11].
In this review we discuss several major classes of vacuolar substrates and current knowledge regarding their degradation and efflux in the model organism S. cerevisiae. We also explore the consequences of dysfunctional degradation and efflux, particularly in multicellular eukaryotes, and outline unanswered questions that require further study ().
Box 1. The big questions.
Nucleic acids
The vast majority of studies on RNA degradation have focused on nuclear and cytoplasmic RNA decay and quality control pathways. However, RNA degradation also occurs in the vacuole in an autophagy-dependent manner, which contributes to cellular RNA homeostasis and regulation of translational fidelity [Citation12]. T2 RNases are a highly-conserved family of endoribonucleases that cleave single-stranded RNA, resulting in mononucleotides or oligonucleotides that terminate in a 3ʹ phosphate group [Citation13]. To date, Rny1 is the only known vacuolar RNase in S. cerevisiae [Citation14]. In the vacuole, Rny1 converts RNA to 3ʹ mononucleotides. Following cleavage by Rny1, the vacuolar phosphatase Pho8 then converts the 3ʹ mononucleotides into nucleosides, which are released into the cytoplasm for further processing [Citation15]. Similar to several autophagy-related proteins, Rny1 levels increase significantly during nitrogen starvation, as do Pho8 levels, although not to the same degree [Citation16].
In yeast cells lacking Rny1, free RNA accumulates in the vacuole following autophagy induction by nitrogen starvation [Citation15]. Similar defects examined in animal studies highlight the physiological importance of RNA degradation in the context of neurodegenerative diseases. In zebrafish lacking the Rny1 ortholog, Rnaset2, rRNA accumulates in neuronal lysosomes [Citation17]. Loss-of-function mutations in the human ortholog, RNASET2, are associated with the neurological disease cystic leukoencephalopathy [Citation18].
There are several remaining questions regarding vacuolar RNA degradation and efflux in yeast. Cells lacking the 2 major vacuolar proteases, Pep4 (proteinase A) and Prb1 (proteinase B), fail to display an increase in nucleoside levels upon nitrogen starvation [Citation15]. As will be discussed later in this review, Pep4 and Prb1 are required for the proteolytic processing and activation of several vacuolar hydrolases as well as lysis of autophagic bodies within the vacuole [Citation19]. Is it the failure to lyse autophagic bodies, failure to proteolytically activate Pho8, or both that accounts for the impaired RNA degradation in pep4∆ prb1∆ cells? It is also unclear which RNA species undergo autophagy-dependent degradation in yeast, and which transporter(s) is/are involved in efflux of the degradation products. It is possible that the nucleoside transporter Fun26 is involved in efflux, based on its localization to the vacuole membrane [Citation20–Citation23].
Lipids
Non-polar, or ‘neutral’, lipids serve a variety of purposes in eukaryotic cells; they can be used as precursors for membrane biogenesis and participate in energy production during starvation [Citation24]. Cells defective in the production of neutral lipids exhibit a block in autophagy at the early stages of autophagosome formation [Citation25,Citation26]. Neutral lipids are stored in the cytoplasm within specialized organelles called lipid droplets (LDs) that can also sequester toxic fatty acids that may be harmful to cells. LDs are comprised of a phospholipid monolayer and associated proteins that surround a hydrophobic core of neutral lipids, mostly consisting of triacylglycerol and steryl esters [Citation24,Citation27]. Lipids can be liberated from LDs in several ways, including both a cytoplasmic process and an autophagy-related process called lipophagy.
In yeast, lipophagy occurs through microautophagy and can be induced in response to nitrogen starvation [Citation28], stationary phase [Citation29], and lipid imbalances resulting from inhibition of phosphatidylcholine biosynthesis [Citation30]. Lipophagy induced by nitrogen starvation or stationary phase requires the core autophagy machinery [Citation28,Citation29]. The role of the autophagic machinery in lipid stress-induced lipophagy is unclear, but ATG7 at least is not required [Citation30]. Once inside the vacuole, turnover of LDs is largely dependent on the lipase Atg15 [Citation28].
In mammalian cells, however, lipophagy occurs through macroautophagy [Citation31]. Studies in mice and humans have indicated that functional lipophagy is important for regulating fat content in the liver. For example, in mice, lipophagy is involved in the generation of free fatty acids to be used in very-low-density lipoprotein production. Furthermore, there is strong correlative evidence linking autophagy to the prevention of nonalcoholic fatty liver disease in humans [Citation32].
Despite the importance of lipophagy, much remains unknown about the terminal steps within the vacuole. Vacuolar lipase activity in yeast is not completely abrogated in Atg15-deficient cells [Citation28]; what accounts for this residual activity? Are there other as yet unidentified vacuolar lipases? Once broken down, how are lipids then recycled?
In addition to these broader issues, many questions still remain about Atg15 itself (Box 1). Atg15 is a vacuolar phospholipase that has activity primarily towards phosphotidylserine and, to a lesser extent, cardiolipin and phosphatidylethanolamine [Citation33,Citation34]. As mentioned above, Atg15 is required for efficient turnover of LDs within the vacuole [Citation28]. Atg15 is also required for breakdown of autophagic bodies and Cvt bodies, the latter of which are single-membrane intravacuolar vesicles that form via the Cvt pathway [Citation34,Citation35]. These functions of Atg15 are critical for cell survival, as cells lacking Atg15 lose viability within 6 d of nitrogen starvation, whereas wild-type cells maintain robust viability at this time point [Citation35]. The regulation of Atg15 activity is poorly understood, but proteolytic processing is hypothesized to play a role in Atg15 activation, similar to many other vacuolar hydrolases [Citation35,Citation36]. If and how Atg15 is proteolytically activated in the vacuole remains to be elucidated.
Polyphosphate
Due to its structural incorporation into nucleic acids and phospholipids as well as roles in protein modification and signal transduction, phosphorus is an essential element for sustaining life [Citation37]. In yeast, phosphorus is primarily stored as chains of inorganic polyphosphate (polyP), most of which is retained in the vacuole [Citation37–Citation40]. Aside from acting as a phosphate storage mechanism, polyP functions in metal chelation in yeast, whereas in mammals it serves diverse functions ranging from activation of inflammatory responses and blood clotting to regulation of bone calcification [Citation40].
In yeast, polyP is simultaneously synthesized and translocated across the vacuolar membrane by the vacuolar transporter chaperone (VTC) complex, which consists of 2 proposed regulatory subunits, Vtc1 and Vtc2 or Vtc3, and the catalytic subunit Vtc4 [Citation40–Citation42]. The VTC complex can form 2 distinct subcomplexes; the first consists of Vtc1, Vtc3, and Vtc4, and localizes primarily to the vacuolar membrane, whereas the second, consisting of Vtc1, Vtc2, and Vtc4, localizes to the cell periphery, but can be found at the vacuole during phosphate starvation [Citation40]. Vtc4 synthesizes polyP from ATP in a metal ion-dependent manner, with Mn2+ being the most effective cofactor. This enzyme is highly stimulated by inorganic pyrophosphate, which is also thought to be a primer for polyP polymerization [Citation43]. The subunits of the VTC complex form a channel that allows for translocation of polyP across the membrane into the vacuolar lumen in a process that is dependent upon a proton gradient established by the vacuolar-type proton-translocating ATPase (V-ATPase) [Citation41,Citation43]. A fifth subunit of the VTC complex, Vtc5, was recently characterized; overexpression of Vtc5 enhances polyP synthesis, whereas deletion of the corresponding gene decreases it [Citation44]. The mechanistic details of how Vtc5 regulates polyP synthesis remain to be determined.
PolyP within the vacuole can be broken down by the polyphosphatase Ppn1 [Citation40], and the expression of this enzyme increases during phosphate starvation [Citation45]. Ppn1 transits to the vacuole via the multivesicular body (MVB) pathway, after which the N-terminal transmembrane domain is cleaved, releasing the soluble enzyme into the vacuole lumen [Citation46]. Activation of Ppn1 is dependent on vacuolar proteases and involves both N-terminal and C-terminal cleavage events [Citation47,Citation48]. Following release from polyP chains, inorganic phosphate is then exported from the vacuole into the cytoplasm [Citation40]. It is suggested that the phosphate transporter Pho91 is responsible for this activity, as it localizes to the vacuole membrane [Citation49].
Extracts from cells lacking Ppn1 and the other major yeast polyphosphatase, Ppx1, which localizes to the cytosol and mitochondria, still have detectable polyphosphatase activity [Citation40,Citation50]. Very recently, Gerasimaitė and Mayer investigated Ynl217w, a previously uncharacterized vacuolar protein [Citation51], and suggested that it may be the remaining vacuolar polyphosphatase, thus the name Ppn2 was proposed. Purified vacuolar lysates from cells lacking Vtc4, Ppn1, and Ppn2 have no phosphatase activity in vitro [Citation52]. Ppn2 is an endopolyphosphatase that, similar to Ppn1, transits to the vacuole through the MVB pathway [Citation52].
PolyP has also been observed in mammalian lysosomes and lysosome-related organelles termed acidocalcisomes; however, not much is known about the mechanisms of lysosomal polyP degradation in mammals [Citation53,Citation54]. Whereas several mammalian endopolyphosphatases, namely NUDT3, 4, 10, and 11, have been identified, they are unlikely to be responsible for lysosomal polyP turnover, as they function optimally at a basic pH, and the yeast homolog of these enzymes, Ddp1, localizes to the cytosol [Citation55].
Remaining questions concerning vacuolar polyphosphate metabolism in yeast include the following (Box 1): No Ppn1 activity is detected in yeast cells lacking Pep4, Prb1, and a third major vacuolar protease, Prc1 (carboxypeptidase Y) [Citation48], suggesting that Ppn1 requires proteolytic activation; which vacuolar protease(s) is/are directly involved in the activation of Ppn1? Is Ppn2 also proteolytically activated and, if so, how? Additionally, polyP is a storage molecule localized to the same cellular compartment as the enzymes Ppn1 and Ppn2 that disassemble it; how are these phosphatases regulated to only be active when appropriate?
Several connections between phosphate metabolism and autophagy have been suggested that also require further study. For example, nitrogen starvation increases Vtc1, Vtc3, and Vtc4 localization to the vacuole membrane, and these proteins are required for microautophagy [Citation56]. How the VTC complex directly participates in microautophagy remains to be determined, as well as how this may or may not connect to phosphate levels and metabolism. It has recently been demonstrated that phosphate starvation can induce autophagy, albeit to a much lower level than autophagy induced by nitrogen or carbon starvation [Citation57]. Does polyphosphate serve as a phosphate source under these conditions?
Carbohydrates
Several types of carbohydrates, including oligosaccharides and storage carbohydrates, can undergo vacuolar degradation in yeast. Ams1 (α-mannosidase) is a peripheral membrane protein associated with the lumenal face of the vacuole membrane [Citation58–Citation61]. In the vacuole, Ams1 is involved in the degradation of free oligosaccharides generated as a result of newly synthesized, but misfolded, glycoproteins undergoing ER-associated protein degradation [Citation62]. Ams1 expression increases during both nitrogen and glucose starvation [Citation16,Citation63], similar to many other hydrolases and autophagy-related genes [Citation64,Citation65]. Expression also increases in response to treatment of cells with rapamycin, a TORC1 inhibitor, which indicates involvement of the TORC1 signaling pathway in the regulation of Ams1 levels [Citation63]. The reason behind this TORC1-dependent regulation is currently unclear, but it suggests that Ams1 may have a role in the digestion of glycoproteins during autophagic recycling, as TORC1 is also a repressor of autophagy [Citation5].
S. cerevisiae can store glucose as either trehalose or glycogen. Trehalose is a glucose disaccharide that accumulates during entry into stationary phase or in nutrient-poor conditions [Citation66]. This carbohydrate has many intracellular functions; in addition to serving as a source of carbon and energy, trehalose can protect cells from stresses including desiccation, temperature extremes, and oxidative and osmotic stress [Citation67,Citation68]. Trehalose can be degraded in the cytosol by the neutral trehalase Nth1 or in the vacuole by the acid trehalase Ath1 [Citation69]. Ath1 is a resident vacuolar protein and has optimal activity under acidic pH [Citation70–Citation72]; however, Ath1 has also been detected at the cell periphery [Citation73,Citation74]. Mutations in ATH1 lead to higher levels of intracellular trehalose and increased resistance to stresses such as freezing, dehydration, and elevated ethanol levels that can cause toxicity [Citation75], as well as an inability to grow on medium where the sole source of carbon is trehalose [Citation76].
Glycogen is a larger polymer of extensively-branched glucose chains [Citation69,Citation77]. When nutrients such as carbon, nitrogen, phosphorous, or sulfur are depleted, glycogen is synthesized in the cytoplasm from glucose donated from UDP-glucose molecules [Citation66,Citation69]. Glycogen synthesis is regulated by many of the same signaling pathways as autophagy. For example, Snf1 (a positive regulator of autophagy) and Pho85 (a negative regulator) [Citation78], also exert the same types of control on glycogen synthesis [Citation69]. Furthermore, inhibition of TORC1 by rapamycin increases glycogen synthesis [Citation79], and cells lacking Tor1 hyperaccumulate glycogen [Citation80].
Glucose can be liberated from glycogen via 2 distinct mechanisms. The first occurs in the cytoplasm, where the glycogen phosphorylase Gph1 releases glucose-1-phosphate from glycogen chain ends, and the debranching enzyme Gdb1 removes glucose at the branch points [Citation81,Citation82]. The second glycogen degradation pathway occurs in the vacuole; the vacuolar glucoamylase Sga1 releases glucose from glycogen by hydrolysis [Citation83–Citation85]. Cells lacking Sga1 show decreased degradation of glycogen in the late stationary phase of growth [Citation78].
It is unclear why there are 2 distinct pools of glycogen and how glycogen is transported into the vacuole, but it is suggested that autophagy has a role in glycogen transport and storage in the vacuole, as atg1∆ cells have reduced glycogen storage [Citation78]. This finding has led to a model whereby some of the glycogen made in the cytosol is transported to the vacuole by autophagy for storage; this localization also protects the glycogen from degradation in the cytosol by Gph1 and Gdb1. Later in starvation, vacuolar glycogen can be degraded by Sga1 [Citation78]. This model is highly speculative and warrants extensive testing. If vacuolar glycogen is delivered via autophagy, does this occur in a selective or nonselective manner? If it is selective, what are the receptor and adaptor/scaffold protein(s) involved in cargo recognition? Additionally, what is the nutritional or intracellular cue to trigger degradation of the vacuolar pool of glycogen?
In multicellular eukaryotes, degradation of vacuolar/lysosomal carbohydrate stores is crucial in maintaining cell health. In mice lacking lysosomal GAA (glucosidase alpha, acid), glycogen overaccumulates in lysosomes in multiple muscle groups [Citation86]. In humans, mutation of GAA leads to Pompe disease (glycogen storage disease type II); the infantile form, which is most severe, is characterized by cardiomegaly, hypotonia, and respiratory distress, with life expectancy being less than 1 y of age [Citation87,Citation88].
Many questions about yeast vacuolar glycogen storage, degradation, and efflux remain. As with polyP storage and degradation, both glycogen and its degradative enzyme, Sga1, are localized in the same intracellular compartment, leading to questions about the regulation of Sga1 activity and possible involvement of proteolytic processing (Box 1). In a screen for mutants affecting glycogen storage, deletion of 11 of the 17 V-ATPase subunits and assembly factors that were screened results in elevated glycogen accumulation, indicating that vacuolar acidification is required for the degradation of glycogen [Citation80], but the reason for this is currently unknown. Additionally, how glucose or mannose exit the vacuole is unclear, as no vacuolar hexose exporter has been identified. Ybr241c may be worth investigating in this regard, as it is a putative transporter of the sugar porter family that localizes to the vacuole membrane [Citation51,Citation89].
Organelles
As discussed above, various smaller cargoes are delivered to the yeast vacuole through autophagy. However, several types of selective autophagy can deliver larger structures including entire organelles or organellar fragments to the vacuole. For example, mitochondria, peroxisomes, and ribosomes can be degraded by autophagic processes termed mitophagy, pexophagy, and ribophagy, respectively [Citation90]. Depending on the cargo and nutrient conditions, this can occur by either macroautophagy or microautophagy. Numerous studies have been devoted to characterizing the induction of these processes, including the identification of target organelles by the autophagy machinery, along with receptors and adaptors involved in phagophore engulfment of these organelles [Citation90]. However, how these organelles are broken down once inside the vacuole remains a mystery. Two illustrative examples we discuss here are mitophagy and micronucleophagy (also termed piecemeal microautophagy of the nucleus).
Under mitophagy-inducing conditions, such as nitrogen starvation following growth in non-fermentable carbon sources that induce proliferation of mitochondria, the cytosolic N terminus of Atg32, a mitochondrial outer membrane protein, is phosphorylated [Citation91]. This facilitates Atg32 interaction with the cytosolic selective autophagy scaffold protein Atg11, which acts in recruiting a portion of the mitochondria to the PAS [Citation91,Citation92]. At the PAS, phosphorylated Atg32 binds to Atg8, which, in its phosphatidylethanolamine-conjugated form, associates with the expanding phagophore [Citation93,Citation94]. Following phagophore membrane expansion and autophagosome completion, the autophagosome travels to the vacuole and membrane fusion occurs, releasing the autophagic body with its mitochondrial cargo into the vacuolar lumen.
As with all macroautophagic cargoes, whether selective or nonselective, breakdown of autophagic bodies must then occur to allow cargo access to the degradative environment of the vacuolar lumen. As previously mentioned, this process is defective in cells lacking Pep4, Prb1, or Atg15 [Citation19,Citation34,Citation35]. Perhaps not surprisingly then, autophagic bodies containing mitochondria are observed in cells lacking Pep4 and Prb1 [Citation95]. Mitochondria are not the only selective autophagy cargo for which this is true; peroxisomes also fail to be degraded in cells lacking Pep4 or Atg15 [Citation96], presumably due to the autophagic body remaining intact.
Mitophagy is an important process for degrading superfluous or damaged mitochondria [Citation91]. During erythrocyte maturation in mammals, for example, mitophagy clears mitochondria from these cells as part of their proper development [Citation97]. Additionally, reactive oxygen species (ROS) produced as natural byproducts of mitochondrial oxidative phosphorylation cause damage to mitochondrial proteins and DNA. This damage then leads to increased ROS production, spawning a vicious cycle of oxidative damage and further mitochondrial dysfunction. Over time, this damage can contribute to aging, cancer, and neurodegenerative diseases [Citation98]. One pathway by which dysfunctional mitochondria are identified and targeted for mitophagy in mammals involves the proteins PINK1/PARK6 and PRKN/PARK2/Parkin. In depolarized mitochondria, the mitochondrial protein PINK1 accumulates on the mitochondrial outer membrane. There, it recruits and activates PRKN, an E3 ubiquitin ligase, which then ubiquitinates several mitochondrial outer membrane proteins, targeting the damaged mitochondria for degradation via mitophagy [Citation91,Citation99]. Mutations in PINK1 and PRKN are associated with autosomal recessive familial Parkinson disease [Citation100–Citation102], underscoring the important role of mitophagy in clearing dysfunctional mitochondria.
Selective autophagy can also occur by microautophagy. Micronucleophagy is a microautophagic process and, as such, occurs by invagination or protrusion of the vacuolar/lysosomal membrane rather than delivery to the vacuole by autophagosomes. In response to nitrogen or carbon starvation in yeast, parts of the nucleus that are nonessential become anchored to the vacuole membrane through interaction of the nuclear membrane protein Nvj1 with the vacuolar membrane protein Vac8; this protein-protein interaction forms nucleus-vacuole junctions [Citation103–Citation105]. Vacuolar membrane invagination coupled with extrusion of the nucleus then occur at these sites before the membranes pinch off, releasing small vesicles into the vacuolar lumen, where they are degraded [Citation103]. Breakdown of these vesicles depends on Pep4 and Atg15, and is also inhibited when cells are treated with the Prb1 inhibitor PMSF [Citation105,Citation106], similar to the breakdown of autophagic bodies resulting from macroautophagy [Citation19,Citation34,Citation35].
Both of these examples of specific autophagy illustrate large gaps in our knowledge of the vacuolar degradation of specific organellar autophagic cargo (Box 1). First, how does Atg15 differentiate between autophagic vesicles derived from the vacuole membrane and the vacuole membrane itself; what happens during microautophagy to mark the invaginated membrane as distinct from its source? Second, concerning both macroatuophagy and microautophagy, which hydrolases are responsible for organellar breakdown following lysis of the autophagic bodies or microautophagic vesicles by Atg15? Third, does Atg15 also disrupt the organelle membranes, or are there other lipases involved? Finally, how are other non-lipid components of the organelles degraded and recycled within the vacuole?
Proteins
Introduction to proteases
The yeast vacuole is home to a vast array of proteases and peptidases (). Whereas synthesis and trafficking of these enzymes have been extensively studied and characterized, this section will focus on what is known about their activities within the context of the vacuole.
Table 1. Substrates and associated vacuolar hydrolases and exporters described in this review.1
The 2 major proteases in the vacuole are the endopeptidases Pep4 and Prb1. Pep4 is an aspartyl endopeptidase related to mammalian CTSD (cathepsin D) and cleaves preferentially between hydrophobic amino acids [Citation107–Citation109]. Prb1 is a subtilisin-like serine endopeptidase with fairly broad substrate specificity similar to porcine chymotrypsin C and trypsin [Citation110–Citation112]. During vegetative growth conditions, the vacuole is responsible for 40% of cellular proteolysis, which increases to 85% during nutrient starvation [Citation2]. Pep4 and Prb1 are critical in this process through their own hydrolytic activities, as well as through proteolytic activation of additional proteases, which will be discussed below [Citation65,Citation113]. When both PEP4 and PRB1 are mutated, protein degradation is severely impaired during nitrogen starvation, and sporulation is almost completely abolished [Citation2,Citation114].
Prc1 is a broad-specificity vacuolar serine carboxypeptidase that prefers cleavage between hydrophobic residues and is thought to contribute to general protein/peptide turnover in the vacuole; however, specific biological substrates have not been defined [Citation51,Citation65,Citation115–Citation117]. Atg42 is a vacuolar serine carboxypeptidase that shares a high degree of amino acid sequence similarity with Prc1 [Citation51,Citation118,Citation119]; as with the latter, the substrate specificity of Atg42 is currently unknown. Both Prc1 and Atg42 are involved in the synthesis of phytochelatins, peptides that bind heavy metal ions [Citation120], and recent work demonstrates that Atg42 and Prc1 are functional homologs required for lysis of autophagic bodies within the vacuole [Citation121].
Cps1 (carboxypeptidase S) is a vacuolar carboxypeptidase that is predicted to belong to a family of zinc metalloproteases [Citation122,Citation123]. Although its intracellular function remains unclear, Cps1 is likely to participate in hydrolysis of leucine from the C terminus of proteins along with Prc1, as Cps1 is required for growth in Prc1-deficient strains when a synthetic dipeptide with leucine as the C-terminal amino acid is provided as the sole source of nitrogen [Citation124,Citation125].
There are 3 known resident vacuolar aminopeptidases. Ape3 (aminopeptidase Y) is a broad-specificity vacuolar protease able to cleave N-terminal Lys, Arg, Leu, Met, Ala, Ser, Phe, Tyr, and Pro residues with varying efficiency and accounts for most of the aminopeptidase activity in the vacuole [Citation126], however, its biological function remains unknown. Another vacuolar aminopeptidase, Ape1 (aminopeptidase I), is activated by Zn2+, cleaves N-terminal leucine residues, and may play a role in glutathione metabolism as discussed below [Citation127–Citation130]. The third resident aminopeptidase, Ape4 (aspartyl aminopeptidase), belongs to the same family of metalloproteases as Ape1, is similar to mammalian aspartyl aminopeptidase, and cleaves the acidic residues Asp and Glu from the N terminus of substrates [Citation131,Citation132]; however, as with Ape3, its biological substrates are unknown [Citation133]. A portion of the Ape4 population resides in the vacuole during vegetative growth, although an increase in vacuolar localization of Ape4 occurs when cells are starved for nutrients, perhaps in order to assist with autophagic protein turnover [Citation133].
Dap2 (dipeptidyl aminopeptidase B) is an integral membrane vacuolar protein that is annotated as a serine hydrolase and, although its biological function remains unknown, it bears homology to Ste13, which cycles between the trans-Golgi network and endosomal system in a phosphorylation-dependent manner and is involved in proteolytic activation of the yeast α-factor [Citation119,Citation134–Citation136].
Many of these vacuolar proteases are required for the terminal steps of autophagy and cell survival under nitrogen starvation conditions. Similar to many autophagy-related genes [Citation64], most of the known vacuolar proteases are upregulated in response to nitrogen starvation or rapamycin treatment, including Ape1, Atg42, Cps1, Pep4, Prb1, and Prc1 [Citation16,Citation137,Citation138]; Ape3 and Dap2 are also upregulated, but to a lesser extent [Citation16]. Pep4 and Prb1 are especially important in cell survival and the autophagic response, as autophagic bodies accumulate within the vacuole in Pep4- and Prb1-deficient cells during nitrogen starvation [Citation19], and pep4∆ cells lose viability in nitrogen starvation after approximately 8 d, whereas wild-type cells still show 90% viability at this time [Citation35]. Prc1-deficent cells do not accumulate autophagic bodies [Citation19], but this is likely due to compensatory effects by Atg42, as autophagic bodies do accumulate in cells lacking both Atg42 and Prc1 [Citation121].
Zymogen activation cascade
Many vacuolar hydrolases are synthesized as inactive zymogens that undergo Pep4- and/or Prb1-dependent proteolytic processing in the vacuole, leading to activation () [Citation36,Citation65,Citation122]. Pep4 undergoes self-mediated processing in the vacuole to remove its N-terminal propeptide and mature into the active enzyme [Citation139]. It was originally thought that Pep4 maturation would be induced by the acidic environment of the vacuole, as most vacuolar hydrolases have an acidic pH optimum [Citation1]. Additionally, mutations that impair activity of the V-ATPase, which is required for vacuolar acidification, show accumulation of autophagic bodies and protein degradation defects following nitrogen starvation [Citation140,Citation141]. However, the maturation of Pep4 and several other Pep4-dependent zymogens is fairly normal in these mutants, albeit somewhat slower [Citation140,Citation142,Citation143], indicating that there are other vacuolar factors involved in Pep4 activation. These factors, as well as how premature activation of Pep4 is prevented, are currently unknown; however, the vacuolar milieu is distinct from that of the cytosol or other organelles, with a relatively high concentration of polyphosphate, as noted above, basic amino acids, calcium, and various other divalent cations, and a lower pH, which may contribute to the regulation of Pep4 activity. Once activated, Pep4 initiates further zymogen activation through Prb1. Prb1 undergoes 2 C-terminal processing events in the vacuole; the first is mediated by Pep4 and the second was thought to be catalyzed by Prb1 itself [Citation144–Citation146]; however, recent evidence suggests that Prc1 and Atg42 are at least partially responsible for the second cleavage [Citation121].
Figure 2. Numerous vacuolar hydrolases undergo proteolytic processing. Arrowheads indicate cleavage sites (the exact site of cleavage within Ape4 is unknown), with proteases involved in cleavage listed above. Gray boxes indicate signal sequences, black boxes indicate propeptides, maize boxes indicate cytoplasmic domains, blue boxes indicate transmembrane domains, and white boxes indicate mature vacuolar domains. aa, amino acid. See text for details.
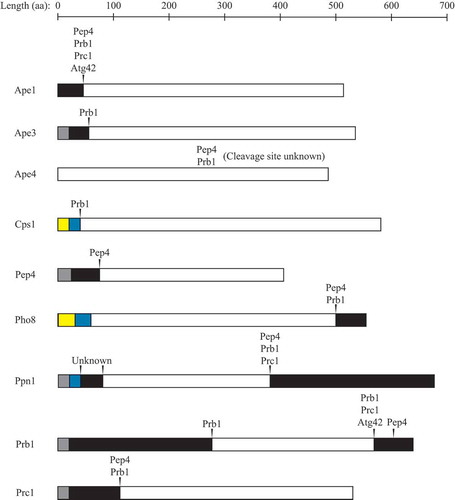
Prc1 is processed to its mature form through sequential N-terminal propeptide cleavage events mediated by Pep4 and Prb1 [Citation122]; mutations in the PEP4 gene result in accumulation of a Prc1 precursor [Citation147], referred to as the Golgi-modified p2 form. Purified Pep4 can process precursor Prc1 to an intermediate form in vitro [Citation142], and an intermediate form of Prc1 is seen in Prb1-deficient cells [Citation148]. It is currently unknown whether the Prc1 homolog Atg42 is proteolytically processed. Ape3 is also processed and activated in a Prb1-dependent manner; vacuolar extracts from cells lacking Pep4, Prb1, Prc1, and Cps1 show no Ape3 enzymatic activity; however, addition of purified Prb1 allows for cleavage of the N-terminal propeptide and an increase in Ape3 activity [Citation126].
Other targets of proteolytic activation are Pho8 and Ppn1, which participate in vacuolar RNA and polyP degradation, respectively, as discussed above. Cleavage of the Pho8 C-terminal propeptide is Pep4 dependent [Citation149]; in this case, however, Pep4 may be acting through Prb1, as overexpression of Prb1 increases activation of Pho8 [Citation150], but it remains to be determined whether activation by Pep4 and Prb1 is direct or indirect. After delivery of Ppn1 to the vacuole by the MVB pathway, the transmembrane domain is cleaved to release soluble Ppn1 into the vacuole lumen [Citation46]. There is no Ppn1 activity in pep4∆ prb1∆ prc1∆ cells [Citation48], and sequencing of the mature enzyme indicates a C-terminal cleavage event [Citation47]. It is also suggested that Ppn2 is delivered to the vacuole by the MVB pathway [Citation52], but whether or not it is proteolytically activated upon arrival is yet to be determined.
Proteolytic activation of zymogens is a strategy also employed by mammals. Lysosomal cathepsin proteases, including CTSD, CTSA, CTSL, CTSK, CTSC, and CTSF, are synthesized as inactive zymogens that undergo processing into active enzymes [Citation151–Citation154].
Not all vacuolar proteases, however, undergo proteolytic activation. Whereas yeast Prb1 does process Cps1 from a membrane-bound to a soluble enzyme within the vacuole () [Citation123], this is not an activating event; Cps1 activity is independent of both Pep4 and Prb1 [Citation124,Citation155]. Processing of precursor Ape1 (prApe1) to its mature form by cleavage of the N-terminal propeptide is also Pep4- and Prb1-dependent [Citation156,Citation157], and is severely impaired in cells lacking Prc1 and Atg42 [Citation121]; however, this may not be absolutely required for its activity, as prApe1 displays some enzymatic activity in pep4∆ cells [Citation158]. Similarly, processing of Ape4 is defective in pep4∆ prb1∆ cells [Citation133], but both full-length and cleaved forms of Ape4 exhibit enzymatic activity [Citation132]. Another protease that has activity independent of Pep4 is Dap2; disruption of the PEP4 gene has no effect on either the apparent molecular weight or in vitro enzymatic activity of Dap2 [Citation134].
Although many proteins undergo Prb1- and/or Pep4-dependent cleavage and activation, it is still unclear in most cases whether this is direct or indirect and whether the activation cascade is more complex. If it is indirect, which proteases function downstream of Prb1 to facilitate the processing of zymogens? Also, Prc1 and Atg42 have recently been shown to affect Prb1 processing [Citation121], and it remains to be seen which other hydrolases are dependent on Prc1 and Atg42 for their activation.
Glutathione catabolism
Whereas many specific functions of vacuolar proteases are unknown, there is evidence in yeast for the involvement of several vacuolar proteases in the catabolism of glutathione (GSH, L-γ-glutamyl-L-cysteinylglycine), a tripeptide that performs many functions in the cell including detoxification of toxic metabolites and protection from oxidative stress [Citation159,Citation160]. GSH is broken down within the vacuole in 2 steps; first the γ-glutamyltranspeptidase Ecm38 hydrolyzes the N-terminal glutamate, followed by degradation of the CysGly dipeptide by an as yet unidentified cysteinylglycine dipeptidase [Citation161,Citation162]. Ecm38 associates with the vacuole membrane [Citation163], with its active site facing the vacuolar lumen [Citation164]. It has been proposed, though not experimentally demonstrated, that Ape1 may act in the second step to degrade the CysGly dipeptide in the vacuole [Citation130]; however, the ability of Ape1 to cleave N-terminal Cys residues has not been previously described.
In Arabidopsis thaliana, GSH catabolism can proceed by a second pathway, during which AT1G03980/PCS (phytochelatin synthase) can remove the C-terminal Gly from GSH, resulting in a γ-GluCys dipeptide [Citation165–Citation167]. Using fluorescent glutathione-S-bimane (GS-bimane) conjugates, it was shown that S. cerevisiae can generate both CysGly-bimane and γ-GluCys-bimane from GS-bimane, indicating that this second degradation pathway also occurs in yeast [Citation168]. While S. cerevisiae does not have a PCS homolog, the vacuolar serine carboxypeptidases Prc1 and Atg42 are required for phytochelatin synthesis in yeast [Citation120], as well as the conversion of GS-bimane to γ-GluCys-bimane, with Atg42 having a larger role than Prc1 [Citation168]. Cells lacking Ecm38 accumulate γ-GluCys-bimane, whereas atg42∆ ecm38∆ prc1∆ cells show no breakdown of GS-bimane [Citation168]. Whether Prc1 and Atg42 cleave the C-terminal Gly from GSH directly or indirectly, perhaps through proteolytic activation of the actual protease, remains to be determined. Also, it is still unclear how γ-GluCys or CysGly dipeptides are broken down in the final step in either GSH degradation pathway, but there is no shortage of candidate vacuolar amino-, carboxy-, and dipeptidases available for future study.
There is some evidence that GSH metabolism and autophagy may be connected; nitrogen starvation causes a migration of the majority of cellular GSH to the vacuole [Citation169]. The pool of GSH increases for ~2 h, followed by a decrease [Citation169]. When GSH biosynthesis is blocked during nitrogen starvation, cell growth is impaired (as measured by dry weight), suggesting that GSH may possibly be used as a source of nitrogen [Citation169]. After 3–4 h of starvation, the specific activity of Ecm38 also increases [Citation169]. Both nitrogen starvation and treatment of cells with the TORC1 inhibitor rapamycin induce expression of Ecm38 [Citation170]. Ecm38 is also derepressed under conditions of sulphate starvation, during which GSH can be used as a source of sulfur and cysteine [Citation171]. Finally, in cells defective in GSH synthesis, there is an increase in mitophagy during nitrogen starvation as compared to wild-type cells, and addition of a cell-permeable GSH derivative reduces mitophagy under these same conditions [Citation172].
There are many questions remaining about the roles of autophagy and vacuolar proteases in GSH metabolism. Is the increased influx of GSH into the vacuole during nitrogen starvation solely dependent on the vacuolar glutathione-S-conjugate transporters Ycf1 and Bpt1 [Citation173–Citation177], or is at least some of it autophagy dependent? If the latter, is this process selective or nonselective and if it is selective, what are the scaffold and receptor proteins involved in cargo recognition? Additionally, how is Ecm38 activity towards GSH regulated? Which vacuolar proteases are responsible for the breakdown of γ-GluCys and CysGly dipeptides in the second step of GSH catabolism? What is the mechanism whereby GSH metabolism regulates mitophagy? As GSH provides an alternative nitrogen source during nitrogen starvation, will blocking GSH catabolism via ECM38 mutation also impair cell viability during prolonged nitrogen starvation?
Human diseases related to lysosomal protease defects
In humans, defects in lysosomal proteolysis can have serious effects on health and disease. Cathepsins, of which there are 15 in humans, are one class of lysosomal proteases [Citation178]. Mutations or defects in the cathepsin-encoding genes result in a variety of pathologies, including several lysosomal storage disorders.
The neuronal ceroid lipofuscinoses (NCLs) correspond to neurodegenerative lysosomal storage disorders that are marked by progressive visual failure, seizures, and dementia [Citation179]. There are 14 different subtypes of NCLs based on the gene affected, although for several, the function of the causative gene is relatively unknown. Five subtypes are due to mutations in lysosomal enzymes, 3 of which are proteases (2 cathepsins and a tripeptidyl peptidase). CLN2 disease is caused by mutations in TPP1 (tripeptidyl peptidase 1), which is not a cathepsin; CLN10 disease is caused by mutations in CTSD; and CLN13 disease is caused by mutations in CTSF [Citation180]. Yeast Pep4 is related to mammalian CTSD [Citation181]. In its most severe form, which involves a complete lack of CTSD activity, CLN10 is characterized by severe neuronal loss, microcephaly, seizures, and death within hours to weeks after birth [Citation151,Citation182]. CLN13 is markedly less severe than CLN10; symptoms include progressive loss of mental and motor function, but do not manifest until late adulthood [Citation178].
Mutations in the CTSA gene, encoding a serine carboxypeptidase that is structurally similar to yeast Prc1 (and likely Atg42 as well), lead to a different type of lysosomal storage disorder called galactosialidosis [Citation152]. CTSA stabilizes a multienzyme complex of GLB1/β-galactosidase and NEU1 (neuraminidase 1), protecting them from degradation in the lysosome [Citation183]. Mutations in CTSA result in deficiency of GLB1 and NEU1, leading to accumulation of glycoproteins within the vacuole [Citation178,Citation184]. In its most severe form, galactosialidosis can cause death within the afflicted infant’s first year of life [Citation178].
Not all diseases involving cathepsin defects are classified as lysosomal storage diseases. For example, loss-of-function mutations in the CTSH (cathepsin H)-encoding gene are associated with myopia [Citation178]. Pycnodysostosis, which is a disease of the bones characterized by osteopetrosis, short stature, and skull deformities, is caused by mutations affecting CTSK [Citation178,Citation185,Citation186]. Papillon-Lefèvre syndrome, which is due to mutations in the CTSC gene, primarily affects the teeth and skin [Citation178]; aggressive periodontitis causes loss of both temporary and permanent teeth, and palmoplantar hyperkeratosis causes thickening of the skin and scaly lesions that crack and fissure [Citation187–Citation189].
Whereas the transcriptional regulation, synthesis, trafficking, and proteolytic processing of vacuolar proteases have been extensively studied, surprisingly little is known about their physiological substrates and intracellular functions. However, it is clear from the wide range of human diseases related to protease defects that vacuolar/lysosomal proteases are critically important in cellular function and survival. A significant effort must be made to better characterize and fully appreciate the vast array of proteases within the yeast vacuole and human lysosome.
Efflux of amino acids
Following protein breakdown, amino acids generated in the vacuole/lysosome can be exported back into the cytoplasm (). The AVT family of proteins in S. cerevisiae consists of 7 predicted membrane-spanning proteins related to transporters of the amino acid/auxin permease (AAAP) family in more complex eukaryotes [Citation190]. Avt1 imports glutamine, asparagine, leucine, isoleucine, and tyrosine into vacuoles for storage [Citation190,Citation191]. The substrate(s), localization, and direction of transport of Avt2 and Avt5 are unknown [Citation190], but in Schizosaccharomyces pombe, the Avt5 homolog localizes to the vacuole membrane and is involved in the uptake of amino acids into vacuoles [Citation192], which may give clues as to its function in S. cerevisiae. The remaining 4 AVT family members, Avt3, Avt4, Avt6, and Avt7 are all indicated to be involved in amino acid efflux from the vacuole. Avt3 and Avt4 export glutamine, leucine, isoleucine, asparagine, and tyrosine from vacuoles into the cytoplasm [Citation191]. Avt4 can also export the basic amino acids arginine, lysine, and histidine [Citation193]. Avt3 may additionally export proline, as vacuolar proline levels are higher in avt3∆ cells than wild-type cells [Citation194]. Avt6 exports glutamate and aspartate from vacuoles [Citation191], whereas recent work has demonstrated that Avt7 may be involved in efflux of glutamine and proline [Citation195].
Another protein involved in vacuolar amino acid efflux is Atg22, a vacuole-localized integral membrane protein that is thought to transport tyrosine, leucine, and isoleucine [Citation196]. Although biochemical methods have yet to confirm the transport activity of Atg22, the observation that cells lacking Atg22 accumulate more vacuolar tyrosine, leucine, and isoleucine as compared to wild-type cells supports the hypothesis regarding this function [Citation196].
Several proteins of the PQ-loop family are also proposed to be involved in vacuolar amino acid efflux. The first, Ers1, is similar to human CTNS (cystinosin, lysosomal cystine transporter), which exports cystine, a disulfide-linked form of cysteine resulting from lysosomal degradation of proteins, from lysosomes [Citation190,Citation197,Citation198]. Ers1 localizes to the vacuole membrane and whereas Ers1-deficient yeast cells show sensitivity to the antibiotic hygromycin B, expression of human CTNS in these cells can complement the hygromycin B sensitivity and confer resistance [Citation198]. Recently, it was demonstrated that Ers1 can transport cystine, although intracellular cystine does not increase in ers1∆ cells [Citation199], possibly indicating the presence of redundant, as yet unidentified, cystine transporters. Additional PQ-loop family members Ypq1, Ypq2, and Rtc2 also localize to the vacuole membrane, and it is proposed that they export basic amino acids such as lysine, arginine, and/or histidine [Citation200].
Efflux of vacuolar amino acids generated by autophagy is critically important to support protein synthesis and continued cell survival during starvation conditions. Similar to many other yeast autophagy-related genes [Citation64], Atg22 expression and protein level increase during nitrogen starvation [Citation137,Citation196]. Additionally, microarray data show that ATG22, AVT1, AVT4, and AVT7 are upregulated in response to rapamycin treatment [Citation138].
Free amino acids generated by autophagy are required to support increased synthesis of several proteins during nitrogen starvation, including Ape1 and Prc1 [Citation201]. In autophagy-deficient atg7∆ cells, synthesis of Ape1 and Prc1 is severely impaired compared to wild-type cells in nitrogen-starvation conditions [Citation201]. Similarly, when the genes encoding the leucine transporters Atg22, Avt3, and Avt4 are deleted, synthesis of Ape1 and Prc1 is reduced in leucine-starvation conditions [Citation196], suggesting that efflux of amino acids resulting from autophagic degradation back into the cytoplasm is necessary to support protein synthesis. The lack of these efflux permeases also affects the ability of cells to survive in starvation conditions; when starved for nitrogen, wild-type cells maintain robust viability over an extended time course, whereas cells lacking Atg22 lose viability at 12 d, cells lacking Avt3 and Avt4 lose viability at 5–6 d, and cells lacking all 3 lose viability at 4 d, similar to an autophagy-defective atg1∆ mutant [Citation196].
In humans, mutations in CTNS lead to the disease cystinosis. This condition is marked by cystine crystal accumulation within the lysosome. In its most severe and common form, the infantile nephropathic form, cystinosis leads to the development of renal Fanconi syndrome by 6–12 m of age and progressive loss of kidney function, growth retardation, neuromuscular dysfunction, hypothyroidism, and vision problems if left untreated [Citation202].
Although many amino acid efflux transporters have been identified and characterized in yeast and humans, further work must be done to determine the transport mechanism of amino acids for which transporters have not yet been identified, such as methionine, alanine, glycine, phenylalanine, serine, threonine, tryptophan, and valine.
Vacuolar proteins of unknown function
In addition to the subset of yeast vacuolar hydrolases discussed herein, there are many known or predicted vacuolar proteins for which a function has not yet been described; more than 200 of the approximately 6000 open reading frames in the yeast genome are annotated as being localized to the vacuole, at least under some conditions [Citation1]. Many of these putative proteins are just beginning to be characterized and some have not been characterized at all. In UniProt, search results for vacuolar hydrolases include Pff1 (YBR074W), Ecm14 (YHR132C), YHR202W, and YNL115C [Citation203], among others.
Pff1 and Ecm14 are predicted to be proteases. Pff1 is a predicted metalloprotease that localizes to the vacuole membrane; it is a multipass integral membrane protein, and topology studies indicate that the protease domain faces the vacuole lumen [Citation204]. Protease activity and substrate specificity have yet to be shown, as well as determination of biological function and regulation. Ecm14 localizes to the vacuole [Citation51] and is predicted to be a zinc-dependent carboxypeptidase [Citation203].
GFP-tagged Yhr202w exhibits vacuolar localization [Citation51] and may have phosphatase and/or nucleotidase activity [Citation205]. Ynl115c localizes to the vacuolar membrane [Citation51] and possesses an α/β-hydrolase fold [Citation205]. Another protein of interest is Yol019w, a protein of unknown function that localizes to the vacuole and is transcriptionally upregulated in cells treated with rapamycin [Citation51,Citation138].
Conclusions and future perspectives
While decades of study have greatly increased our knowledge of vacuolar substrate degradation and efflux in yeast, there are still many gaps in this knowledge, especially concerning protein substrates. Many known vacuolar proteases have indeterminate substrate specificities and intracellular functions, while many predicted proteases remain to be characterized. In addition, for many vacuolar zymogens it is still unknown whether activation via Pep4 and/or Prb1 occurs directly or indirectly through the action of other proteases. In the case of indirect activation, what are the additional proteases involved in the activation and processing cascade? Vacuolar proteases are especially important during nutrient starvation when autophagy is induced, as the vacuole is almost entirely responsible for cellular protein degradation under these conditions [Citation2]. In addition, many human diseases are associated with defects in lysosomal proteolysis and efflux; therefore, it is critical that we increase our understanding of these vacuolar/lysosomal events.
Disclosure statement
No potential conflict of interest was reported by the authors.
Additional information
Funding
References
- Li SC, Kane PM The yeast lysosome-like vacuole: endpoint and crossroads. Biochim Biophys Acta. 2009 Apr;1793(4):650–663. PubMed PMID: 18786576; PubMed Central PMCID: PMCPMC2906225. eng.
- Teichert U, Mechler B, Müller H, et al. Lysosomal (vacuolar) proteinases of yeast are essential catalysts for protein degradation, differentiation, and cell survival. J Biol Chem. 1989 Sep;264(27):16037–16045. PubMed PMID: 2674123; eng.
- Feyder S, De Craene JO, Bär S, et al. Membrane trafficking in the yeast Saccharomyces cerevisiae model. Int J Mol Sci. 2015 Jan;16(1):1509–1525. PubMed PMID: 25584613; PubMed Central PMCID: PMCPMC4307317. eng.
- Wen X, Klionsky DJ An overview of macroautophagy in yeast. J Mol Biol. 2016 May;428(9 Pt A):1681–1699. PubMed PMID: 26908221; PubMed Central PMCID: PMCPMC4846508. eng.
- Reggiori F, Klionsky DJ Autophagic processes in yeast: mechanism, machinery and regulation. Genetics. 2013 Jun;194(2):341–361. PubMed PMID: 23733851; PubMed Central PMCID: PMCPMC3664846. eng.
- Suzuki K, Kirisako T, Kamada Y, et al. The pre-autophagosomal structure organized by concerted functions of APG genes is essential for autophagosome formation. Embo J. 2001 Nov;20(21):5971–5981. PubMed PMID: 11689437; PubMed Central PMCID: PMCPMC125692. eng.
- Mizushima N The role of the Atg1/ULK1 complex in autophagy regulation. Curr Opin Cell Biol. 2010 Apr;22(2):132–139. PubMed PMID: 20056399; eng.
- Greiner-Tollersrud OK, Berg T, Saftig P. Lysosomal storage disorders. Boston, MA: Springer US; 2005. p. 60–73.
- Boustany RM Lysosomal storage diseases–the horizon expands. Nat Rev Neurol. 2013 Oct;9(10):583–598. PubMed PMID: 23938739; eng.
- Meikle PJ, Hopwood JJ, Clague AE, et al. Prevalence of lysosomal storage disorders. JAMA. 1999 Jan;281(3):249–254. PubMed PMID: 9918480; eng.
- Poorthuis BJ, Wevers RA, Kleijer WJ, et al. The frequency of lysosomal storage diseases in The Netherlands. Hum Genet. 1999 Jul-Aug; 105 (1–2): 151–156. PubMed PMID: 10480370; eng.
- Frankel LB, Lubas M, Lund AH Emerging connections between RNA and autophagy. Autophagy. 2017 Jan;13(1):3–23. PubMed PMID: 27715443; PubMed Central PMCID: PMCPMC5240835. eng.
- Irie M Structure-function relationships of acid ribonucleases: lysosomal, vacuolar, and periplasmic enzymes. Pharmacol Ther. 1999 Feb;81(2):77–89. PubMed PMID: 10190580; eng.
- MacIntosh GC, Bariola PA, Newbigin E, et al. Characterization of Rny1, the Saccharomyces cerevisiae member of the T2 RNase family of RNases: unexpected functions for ancient enzymes? Proc Natl Acad Sci U S A. 2001 Jan;98(3):1018–1023. PubMed PMID: 11158587; PubMed Central PMCID: PMCPMC14701. eng.
- Huang H, Kawamata T, Horie T, et al. Bulk RNA degradation by nitrogen starvation-induced autophagy in yeast. Embo J. 2015 Jan;34(2):154–168. PubMed PMID: 25468960; PubMed Central PMCID: PMCPMC4337068. eng.
- Müller M, Schmidt O, Angelova M, et al. The coordinated action of the MVB pathway and autophagy ensures cell survival during starvation. Elife. 2015 Apr;4:e07736. PubMed PMID: 25902403; PubMed Central PMCID: PMCPMC4424281. eng.
- Haud N, Kara F, Diekmann S, et al. rnaset2 mutant zebrafish model familial cystic leukoencephalopathy and reveal a role for RNase T2 in degrading ribosomal RNA. Proc Natl Acad Sci U S A. 2011 Jan;108(3):1099–1103. PubMed PMID: 21199949; PubMed Central PMCID: PMCPMC3024650. eng.
- Henneke M, Diekmann S, Ohlenbusch A, et al. RNASET2-deficient cystic leukoencephalopathy resembles congenital cytomegalovirus brain infection. Nat Genet. 2009 Jul;41(7):773–775. PubMed PMID: 19525954; eng.
- Takeshige K, Baba M, Tsuboi S, et al. Autophagy in yeast demonstrated with proteinase-deficient mutants and conditions for its induction. J Cell Biol. 1992 Oct;119(2):301–311. PubMed PMID: 1400575; PubMed Central PMCID: PMCPMC2289660. eng.
- Wiederhold E, Gandhi T, Permentier HP, et al. The yeast vacuolar membrane proteome. Mol Cell Proteomics. 2009 Feb;8(2):380–392. PubMed PMID: 19001347; eng.
- Vickers MF, Yao SY, Baldwin SA, et al. Nucleoside transporter proteins of Saccharomyces cerevisiae. Demonstration of a transporter (FUI1) with high uridine selectivity in plasma membranes and a transporter (FUN26) with broad nucleoside selectivity in intracellular membranes. J Biol Chem. 2000 Aug;275(34):25931–25938. PubMed PMID: 10827169; eng.
- Boswell-Casteel RC, Johnson JM, Duggan KD, et al. FUN26 (function unknown now 26) protein from saccharomyces cerevisiae is a broad selectivity, high affinity, nucleoside and nucleobase transporter. J Biol Chem. 2014 Aug;289(35):24440–24451. PubMed PMID: 25035431; PubMed Central PMCID: PMCPMC4148870. eng.
- Lu SP, Lin SJ Phosphate-responsive signaling pathway is a novel component of NAD+ metabolism in Saccharomyces cerevisiae. J Biol Chem. 2011 Apr;286(16):14271–14281. PubMed PMID: 21349851; PubMed Central PMCID: PMCPMC3077628. eng.
- Barbosa AD, Siniossoglou S Function of lipid droplet-organelle interactions in lipid homeostasis. Biochim Biophys Acta. 2017 Sep;1864(9):1459–1468. PubMed PMID: 28390906; eng.
- Li D, Song JZ, Li H, et al. Storage lipid synthesis is necessary for autophagy induced by nitrogen starvation. FEBS Lett. 2015 Jan;589(2):269–276. PubMed PMID: 25500271; eng.
- Shpilka T, Welter E, Borovsky N, et al. Lipid droplets and their component triglycerides and steryl esters regulate autophagosome biogenesis. Embo J. 2015 Aug;34(16):2117–2131. PubMed PMID: 26162625; PubMed Central PMCID: PMCPMC4557665. eng.
- Wang CW Lipid droplet dynamics in budding yeast. Cell Mol Life Sci. 2015 Jul;72(14):2677–2695. PubMed PMID: 25894691; eng.
- van Zutphen T, Todde V, de Boer R, et al. Lipid droplet autophagy in the yeast Saccharomyces cerevisiae. Mol Biol Cell. 2014 Jan;25(2):290–301. PubMed PMID: 24258026; PubMed Central PMCID: PMCPMC3890349. eng.
- Wang CW, Miao YH, Chang YS A sterol-enriched vacuolar microdomain mediates stationary phase lipophagy in budding yeast. J Cell Biol. 2014 Aug;206(3):357–366. PubMed PMID: 25070953; PubMed Central PMCID: PMCPMC4121974. eng.
- Vevea JD, Garcia EJ, Chan RB, et al. Role for lipid droplet biogenesis and microlipophagy in adaptation to lipid imbalance in yeast. Dev Cell. 2015 Dec;35(5):584–599. PubMed PMID: 26651293; PubMed Central PMCID: PMCPMC4679156. eng.
- Singh R, Kaushik S, Wang Y, et al. Autophagy regulates lipid metabolism. Nature. 2009 Apr;458(7242):1131–1135. PubMed PMID: 19339967; PubMed Central PMCID: PMCPMC2676208. eng.
- Martinez-Lopez N, Singh R Autophagy and lipid droplets in the liver. Annu Rev Nutr. 2015;35:215–237. PubMed PMID: 26076903; eng.
- Ramya V, Rajasekharan R ATG15 encodes a phospholipase and is transcriptionally regulated by YAP1 in Saccharomyces cerevisiae. FEBS Lett. 2016 Sep;590(18):3155–3167. PubMed PMID: 27543826; eng.
- Epple UD, Suriapranata I, Eskelinen EL, et al. Aut5/Cvt17p, a putative lipase essential for disintegration of autophagic bodies inside the vacuole. J Bacteriol. 2001 Oct;183(20):5942–5955. PubMed PMID: 11566994; PubMed Central PMCID: PMCPMC99673. eng.
- Teter SA, Eggerton KP, Scott SV, et al. Degradation of lipid vesicles in the yeast vacuole requires function of Cvt17, a putative lipase. J Biol Chem. 2001 Jan;276(3):2083–2087. PubMed PMID: 11085977; PubMed Central PMCID: PMCPMC2749705. eng.
- Klionsky DJ, Herman PK, Emr SD The fungal vacuole: composition, function, and biogenesis. Microbiol Rev. 1990 Sep;54(3):266–292. PubMed PMID: 2215422; PubMed Central PMCID: PMCPMC372777. eng.
- Yang SY, Huang TK, Kuo HF, et al. Role of vacuoles in phosphorus storage and remobilization. J Exp Bot. 2017 Jan. doi: 10.1093/jxb/erw481. PubMed PMID: 28077447; eng.
- Kornberg A Inorganic polyphosphate: a molecule of many functions. Prog Mol Subcell Biol. 1999;23:1–18. PubMed PMID: 10448669; eng.
- Saito K, Ohtomo R, Kuga-Uetake Y, et al. Direct labeling of polyphosphate at the ultrastructural level in Saccharomyces cerevisiae by using the affinity of the polyphosphate binding domain of Escherichia coli exopolyphosphatase. Appl Environ Microbiol. 2005 Oct;71(10):5692–5701. PubMed PMID: 16204477; PubMed Central PMCID: PMCPMC1266008. eng.
- Gerasimaitė R, Mayer A Enzymes of yeast polyphosphate metabolism: structure, enzymology and biological roles. Biochem Soc Trans. 2016 Feb;44(1):234–239. PubMed PMID: 26862210; eng.
- Gerasimaitė R, Sharma S, Desfougères Y, et al. Coupled synthesis and translocation restrains polyphosphate to acidocalcisome-like vacuoles and prevents its toxicity. J Cell Sci. 2014 Dec;127(Pt 23):5093–5104. doi: 10.1242/jcs.159772. PubMed PMID: 25315834; eng.
- Cohen A, Perzov N, Nelson H, et al. A novel family of yeast chaperons involved in the distribution of V-ATPase and other membrane proteins. J Biol Chem. 1999 Sep;274(38):26885–26893. PubMed PMID: 10480897; eng.
- Hothorn M, Neumann H, Lenherr ED, et al. Catalytic core of a membrane-associated eukaryotic polyphosphate polymerase. Science. 2009 Apr;324(5926):513–516. PubMed PMID: 19390046; eng.
- Desfougères Y, Gerasimaitė RU, Jessen HJ, et al. Vtc5, a novel subunit of the vacuolar transporter chaperone complex, regulates polyphosphate synthesis and phosphate homeostasis in yeast. J Biol Chem. 2016 Oct;291(42):22262–22275. PubMed PMID: 27587415; PubMed Central PMCID: PMCPMC5064005. eng.
- Ogawa N, DeRisi J, Brown PO New components of a system for phosphate accumulation and polyphosphate metabolism in Saccharomyces cerevisiae revealed by genomic expression analysis. Mol Biol Cell. 2000 Dec;11(12):4309–4321. PubMed PMID: 11102525; PubMed Central PMCID: PMCPMC15074. eng.
- Reggiori F, Pelham HR Sorting of proteins into multivesicular bodies: ubiquitin-dependent and -independent targeting. Embo J. 2001 Sep;20(18):5176–5186. PubMed PMID: 11566881; PubMed Central PMCID: PMCPMC125630. eng.
- Shi X, Kornberg A Endopolyphosphatase in Saccharomyces cerevisiae undergoes post-translational activations to produce short-chain polyphosphates. FEBS Lett. 2005 Mar;579(9):2014–2018. PubMed PMID: 15792812; eng.
- Sethuraman A, Rao NN, Kornberg A The endopolyphosphatase gene: essential in Saccharomyces cerevisiae. Proc Natl Acad Sci U S A. 2001 Jul;98(15):8542–8547. PubMed PMID: 11447286; PubMed Central PMCID: PMCPMC37472. eng.
- Hürlimann HC, Stadler-Waibel M, Werner TP, et al. Pho91 Is a vacuolar phosphate transporter that regulates phosphate and polyphosphate metabolism in Saccharomyces cerevisiae. Mol Biol Cell. 2007 Nov;18(11):4438–4445. PubMed PMID: 17804816; PubMed Central PMCID: PMCPMC2043573. eng.
- Lichko LP, Kulakovskaya TV, Kulakovskaya EV, et al. Inactivation of PPX1 and PPN1 genes encoding exopolyphosphatases of Saccharomyces cerevisiae does not prevent utilization of polyphosphates as phosphate reserve. Biochemistry (Mosc). 2008 Sep;73(9):985–989. PubMed PMID: 18976214; eng.
- Huh WK, Falvo JV, Gerke LC, et al. Global analysis of protein localization in budding yeast. Nature. 2003 Oct;425(6959):686–691. PubMed PMID: 14562095; eng.
- Gerasimaitė R, Mayer A Ppn2, a novel Zn(2+)-dependent polyphosphatase in the acidocalcisome-like yeast vacuole. J Cell Sci. 2017 May;130(9):1625–1636. PubMed PMID: 28302909; eng.
- Pisoni RL, Lindley ER Incorporation of [32P]orthophosphate into long chains of inorganic polyphosphate within lysosomes of human fibroblasts. J Biol Chem. 1992 Feb;267(6):3626–3631. PubMed PMID: 1740414; eng.
- Moreno-Sanchez D, Hernandez-Ruiz L, Fa R, et al. Polyphosphate is a novel pro-inflammatory regulator of mast cells and is located in acidocalcisomes. J Biol Chem. 2012 Aug;287(34):28435–28444. PubMed PMID: 22761438; PubMed Central PMCID: PMCPMC3436523. eng.
- Lonetti A, Szijgyarto Z, Bosch D, et al. Identification of an evolutionarily conserved family of inorganic polyphosphate endopolyphosphatases. J Biol Chem. 2011 Sep;286(37):31966–31974. PubMed PMID: 21775424; PubMed Central PMCID: PMCPMC3173201. eng.
- Uttenweiler A, Schwarz H, Neumann H, et al. The vacuolar transporter chaperone (VTC) complex is required for microautophagy. Mol Biol Cell. 2007 Jan;18(1):166–175. PubMed PMID: 17079729; PubMed Central PMCID: PMCPMC1751332. eng.
- Yokota H, Gomi K, Shintani T Induction of autophagy by phosphate starvation in an Atg11-dependent manner in Saccharomyces cerevisiae. Biochem Biophys Res Commun. 2017 Jan;483(1):522–527. PubMed PMID: 28013049; eng.
- Van der Wilden W, Matile P, Schellenberg M, et al. Vacuolar membranes: isolation from yeast cells. Z Naturforsch 28c. 1973:416–421.
- Opheim DJ alpha-D-Mannosidase of Saccharomyces cerevisiae. Characterization and modulation of activity. Biochim Biophys Acta. 1978 May;524(1):121–130. PubMed PMID: 350285; eng.
- Yoshihisa T, Anraku Y A novel pathway of import of alpha-mannosidase, a marker enzyme of vacuolar membrane, in Saccharomyces cerevisiae. J Biol Chem. 1990 Dec;265(36):22418–22425. PubMed PMID: 2266133; eng.
- Yoshihisa T, Ohsumi Y, Anraku Y Solubilization and purification of alpha-mannosidase, a marker enzyme of vacuolar membranes in Saccharomyces cerevisiae. J Biol Chem. 1988 Apr;263(11):5158–5163. PubMed PMID: 3281936; eng.
- Chantret I, Frénoy JP, Moore SE Free-oligosaccharide control in the yeast Saccharomyces cerevisiae: roles for peptide: N-glycanase(Png1p) and vacuolar mannosidase (Ams1p). Biochem J. 2003 Aug;373(Pt 3):901–908. PubMed PMID: 12723970; PubMed Central PMCID: PMCPMC1223533. eng.
- Umekawa M, Ujihara M, Makishima K, et al. The signaling pathways underlying starvation-induced upregulation of α-mannosidase Ams1 in Saccharomyces cerevisiae. Biochim Biophys Acta. 2016 Jun;1860(6):1192–1201. PubMed PMID: 26947009; eng.
- Cebollero E, Reggiori F Regulation of autophagy in yeast Saccharomyces cerevisiae. Biochim Biophys Acta. 2009 Sep;1793(9):1413–1421. PubMed PMID: 19344676; eng.
- Van Den Hazel HB, Kielland-Brandt MC, Winther JR Review: biosynthesis and function of yeast vacuolar proteases. Yeast. 1996 Jan;12(1):1–16. PubMed PMID: 8789256; eng.
- Lillie SH, Pringle JR Reserve carbohydrate metabolism in Saccharomyces cerevisiae: responses to nutrient limitation. J Bacteriol. 1980 Sep;143(3):1384–1394. PubMed PMID: 6997270; PubMed Central PMCID: PMCPMC294518. eng.
- Elbein AD, Pan YT, Pastuszak I, et al. New insights on trehalose: a multifunctional molecule. Glycobiology. 2003 Apr;13(4):17R–27R. PubMed PMID: 12626396; eng.
- Eleutherio E, Panek A, De Mesquita JF, et al. Revisiting yeast trehalose metabolism. Curr Genet. 2015 Aug;61(3):263–274. PubMed PMID: 25209979; eng.
- François J, Parrou JL Reserve carbohydrates metabolism in the yeast Saccharomyces cerevisiae. FEMS Microbiol Rev. 2001 Jan;25(1):125–145. PubMed PMID: 11152943; eng.
- Keller F, Schellenberg M, Wiemken A Localization of trehalase in vacuoles and of trehalose in the cytosol of yeast (Saccharomyces cerevisiae). Arch Microbiol. 1982 Jun;131(4):298–301. PubMed PMID: 7052008; eng.
- Mittenbühler K, Holzer H Purification and characterization of acid trehalase from the yeast suc2 mutant. J Biol Chem. 1988 Jun;263(17):8537–8543. PubMed PMID: 3286651; eng.
- Huang J, Reggiori F, Klionsky DJ The transmembrane domain of acid trehalase mediates ubiquitin-independent multivesicular body pathway sorting. Mol Biol Cell. 2007 Jul;18(7):2511–2524. PubMed PMID: 17475771; PubMed Central PMCID: PMCPMC1924822. eng.
- Jules M, Guillou V, François J, et al. Two distinct pathways for trehalose assimilation in the yeast Saccharomyces cerevisiae. Appl Environ Microbiol. 2004 May;70(5):2771–2778. PubMed PMID: 15128531; PubMed Central PMCID: PMCPMC404389. eng.
- He S, Bystricky K, Leon S, et al. The Saccharomyces cerevisiae vacuolar acid trehalase is targeted at the cell surface for its physiological function. FEBS J. 2009 Oct;276(19):5432–5446. PubMed PMID: 19703229; eng.
- Kim J, Alizadeh P, Harding T, et al. Disruption of the yeast ATH1 gene confers better survival after dehydration, freezing, and ethanol shock: potential commercial applications. Appl Environ Microbiol. 1996 May;62(5):1563–1569. PubMed PMID: 8633854; PubMed Central PMCID: PMCPMC167930. eng.
- Nwaka S, Mechler B, Holzer H Deletion of the ATH1 gene in Saccharomyces cerevisiae prevents growth on trehalose. FEBS Lett. 1996 May; 386 (2–3): 235–238. PubMed PMID: 8647289; eng.
- Wilson WA, Roach PJ, Montero M, et al. Regulation of glycogen metabolism in yeast and bacteria. FEMS Microbiol Rev. 2010 Nov;34(6):952–985. PubMed PMID: 20412306; PubMed Central PMCID: PMCPMC2927715. eng.
- Wang Z, Wilson WA, Fujino MA, et al. Antagonistic controls of autophagy and glycogen accumulation by Snf1p, the yeast homolog of AMP-activated protein kinase, and the cyclin-dependent kinase Pho85p. Mol Cell Biol. 2001 Sep;21(17):5742–5752. PubMed PMID: 11486014; PubMed Central PMCID: PMCPMC87294. eng.
- Barbet NC, Schneider U, Helliwell SB, et al. TOR controls translation initiation and early G1 progression in yeast. Mol Biol Cell. 1996 Jan;7(1):25–42. PubMed PMID: 8741837; PubMed Central PMCID: PMCPMC278610. eng.
- Wilson WA, Wang Z, Roach PJ Systematic identification of the genes affecting glycogen storage in the yeast Saccharomyces cerevisiae: implication of the vacuole as a determinant of glycogen level. Mol Cell Proteomics. 2002 Mar;1(3):232–242. PubMed PMID: 12096123; eng.
- Hwang PK, Tugendreich S, Fletterick RJ Molecular analysis of GPH1, the gene encoding glycogen phosphorylase in Saccharomyces cerevisiae. Mol Cell Biol. 1989 Apr;9(4):1659–1666. PubMed PMID: 2657401; PubMed Central PMCID: PMCPMC362584. eng.
- Teste MA, Enjalbert B, Parrou JL, et al. The Saccharomyces cerevisiae YPR184w gene encodes the glycogen debranching enzyme. FEMS Microbiol Lett. 2000 Dec;193(1):105–110. PubMed PMID: 11094287; eng.
- Colonna WJ, Magee PT Glycogenolytic enzymes in sporulating yeast. J Bacteriol. 1978 Jun;134(3):844–853. PubMed PMID: 350852; PubMed Central PMCID: PMCPMC222331. eng.
- Yamashita I, Fukui S Transcriptional control of the sporulation-specific glucoamylase gene in the yeast Saccharomyces cerevisiae. Mol Cell Biol. 1985 Nov;5(11):3069–3073. PubMed PMID: 3939312; PubMed Central PMCID: PMCPMC369120. eng.
- Pugh TA, Shah JC, Magee PT, et al. Characterization and localization of the sporulation glucoamylase of Saccharomyces cerevisiae. Biochim Biophys Acta. 1989 Feb;994(3):200–209. PubMed PMID: 2493265; eng.
- Fukuda T, Ahearn M, Roberts A, et al. Autophagy and mistargeting of therapeutic enzyme in skeletal muscle in Pompe disease. Mol Ther. 2006 Dec;14(6):831–839. PubMed PMID: 17008131; PubMed Central PMCID: PMCPMC2693339. eng.
- Kishnani PS, Hwu WL, Mandel H, et al. A retrospective, multinational, multicenter study on the natural history of infantile-onset Pompe disease. J Pediatr. 2006 May;148(5):671–676. PubMed PMID: 16737883; eng.
- van den Hout HM, Hop W, van Diggelen OP, et al. The natural course of infantile Pompe’s disease: 20 original cases compared with 133 cases from the literature. Pediatrics. 2003 Aug;112(2):332–340. PubMed PMID: 12897283; eng.
- Palma M, Goffeau A, Spencer-Martins I, et al. A phylogenetic analysis of the sugar porters in hemiascomycetous yeasts. J Mol Microbiol Biotechnol. 2007; 12 (3–4): 241–248. PubMed PMID: 17587872; eng.
- Suzuki K Selective autophagy in budding yeast. Cell Death Differ. 2013 Jan;20(1):43–48. doi: 10.1038/cdd.2012.73. PubMed PMID: 22705847; PubMed Central PMCID: PMCPMC3524628. eng.
- Kanki T, Furukawa K, Yamashita S Mitophagy in yeast: molecular mechanisms and physiological role. Biochim Biophys Acta. 2015 Oct;1853(10 Pt B):2756–2765. PubMed PMID: 25603537; eng.
- Aoki Y, Kanki T, Hirota Y, et al. Phosphorylation of Serine 114 on Atg32 mediates mitophagy. Mol Biol Cell. 2011 Sep;22(17):3206–3217. PubMed PMID: 21757540; PubMed Central PMCID: PMCPMC3164466. eng.
- Farré JC, Burkenroad A, Burnett SF, et al. Phosphorylation of mitophagy and pexophagy receptors coordinates their interaction with Atg8 and Atg11. EMBO Rep. 2013 May;14(5):441–449. PubMed PMID: 23559066; PubMed Central PMCID: PMCPMC3642380. eng.
- Ichimura Y, Kirisako T, Takao T, et al. A ubiquitin-like system mediates protein lipidation. Nature. 2000 Nov;408(6811):488–492. PubMed PMID: 11100732; eng.
- Okamoto K, Kondo-Okamoto N, Ohsumi Y Mitochondria-anchored receptor Atg32 mediates degradation of mitochondria via selective autophagy. Dev Cell. 2009 Jul;17(1):87–97. PubMed PMID: 19619494; eng.
- Epple UD, Eskelinen EL, Thumm M Intravacuolar membrane lysis in Saccharomyces cerevisiae. Does vacuolar targeting of Cvt17/Aut5p affect its function? J Biol Chem. 2003 Mar;278(10):7810–7821. PubMed PMID: 12499386; eng.
- Ashrafi G, Schwarz TL The pathways of mitophagy for quality control and clearance of mitochondria. Cell Death Differ. 2013 Jan;20(1):31–42. PubMed PMID: 22743996; PubMed Central PMCID: PMCPMC3524633. eng.
- Wallace DC A mitochondrial paradigm of metabolic and degenerative diseases, aging, and cancer: a dawn for evolutionary medicine. Annu Rev Genet. 2005;39:359–407. PubMed PMID: 16285865; PubMed Central PMCID: PMCPMC2821041. eng.
- Pickrell AM, Youle RJ The roles of PINK1, parkin, and mitochondrial fidelity in Parkinson’s disease. Neuron. 2015 Jan;85(2):257–273. PubMed PMID: 25611507; PubMed Central PMCID: PMCPMC4764997. eng.
- Rodolfo C, Campello S, Cecconi F Mitophagy in neurodegenerative diseases. Neurochem Int. 2017 Aug. doi: 10.1016/j.neuint.2017.08.004. PubMed PMID: 28797885; eng.
- Kitada T, Asakawa S, Hattori N, et al. Mutations in the parkin gene cause autosomal recessive juvenile parkinsonism. Nature. 1998 Apr;392(6676):605–608. PubMed PMID: 9560156; eng.
- Valente EM, Salvi S, Ialongo T, et al. PINK1 mutations are associated with sporadic early-onset parkinsonism. Ann Neurol. 2004 Sep;56(3):336–341. PubMed PMID: 15349860; eng.
- Kvam E, Goldfarb DS Nucleus-vacuole junctions and piecemeal microautophagy of the nucleus in S. cerevisiae. Autophagy. 2007 Mar-Apr;3(2):85–92. PubMed PMID: 17204844; eng.
- Pan X, Roberts P, Chen Y, et al. Nucleus-vacuole junctions in Saccharomyces cerevisiae are formed through the direct interaction of Vac8p with Nvj1p. Mol Biol Cell. 2000 Jul;11(7):2445–2457. PubMed PMID: 10888680; PubMed Central PMCID: PMCPMC14931. eng.
- Roberts P, Moshitch-Moshkovitz S, Kvam E, et al. Piecemeal microautophagy of nucleus in Saccharomyces cerevisiae. Mol Biol Cell. 2003 Jan;14(1):129–141. PubMed PMID: 12529432; PubMed Central PMCID: PMCPMC140233. eng.
- Krick R, Muehe Y, Prick T, et al. Piecemeal microautophagy of the nucleus requires the core macroautophagy genes. Mol Biol Cell. 2008 Oct;19(10):4492–4505. PubMed PMID: 18701704; PubMed Central PMCID: PMCPMC2555948. eng.
- Dreyer T Substrate specificity of proteinase yscA from saccharomyces cerevisiae. Carlsberg Res Commun. 1989;54(3):85–97. PubMed PMID: 2478140; eng.
- Ammerer G, Hunter CP, Rothman JH, et al. PEP4 gene of Saccharomyces cerevisiae encodes proteinase A, a vacuolar enzyme required for processing of vacuolar precursors. Mol Cell Biol. 1986 Jul;6(7):2490–2499. PubMed PMID: 3023936; PubMed Central PMCID: PMCPMC367803. eng.
- Woolford CA, Daniels LB, Park FJ, et al. The PEP4 gene encodes an aspartyl protease implicated in the posttranslational regulation of Saccharomyces cerevisiae vacuolar hydrolases. Mol Cell Biol. 1986 Jul;6(7):2500–2510. PubMed PMID: 3537721; PubMed Central PMCID: PMCPMC367804. eng.
- Moehle CM, Tizard R, Lemmon SK, et al. Protease B of the lysosomelike vacuole of the yeast Saccharomyces cerevisiae is homologous to the subtilisin family of serine proteases. Mol Cell Biol. 1987 Dec;7(12):4390–4399. PubMed PMID: 3325823; PubMed Central PMCID: PMCPMC368122. eng.
- Lenney JF, Matile P, Wiemken A, et al. Activities and cellular localization of yeast proteases and their inhibitors. Biochem Biophys Res Commun. 1974 Oct;60(4):1378–1383. PubMed PMID: 4609372; eng.
- Kominami E, Hoffschulte H, Leuschel L, et al. The substrate specificity of proteinase B from baker’s yeast. Biochim Biophys Acta. 1981 Sep;661(1):136–141. PubMed PMID: 7028121; eng.
- Knop M, Schiffer HH, Rupp S, et al. Vacuolar/lysosomal proteolysis: proteases, substrates, mechanisms. Curr Opin Cell Biol. 1993 Dec;5(6):990–996. PubMed PMID: 8129953; eng.
- Zubenko GS, Jones EW Protein degradation, meiosis and sporulation in proteinase-deficient mutants of Saccharomyces cerevisiae. Genetics. 1981 Jan;97(1):45–64. PubMed PMID: 7021321; PubMed Central PMCID: PMCPMC1214387. eng.
- Hayashi R Carboxypeptidase Y. Methods Enzymol. 1976;45:568–587. PubMed PMID: 13269; eng.
- Jung G, Ueno H, Hayashi R Carboxypeptidase Y: structural basis for protein sorting and catalytic triad. J Biochem. 1999 Jul;126(1):1–6. PubMed PMID: 10393313; eng.
- Stennicke HR, Mortensen UH, Breddam K Studies on the hydrolytic properties of (serine) carboxypeptidase Y. Biochemistry. 1996 Jun;35(22):7131–7141. PubMed PMID: 8679540; eng.
- Nasr F, Bécam AM, Grzybowska E, et al. An analysis of the sequence of part of the right arm of chromosome II of S. cerevisiae reveals new genes encoding an amino-acid permease and a carboxypeptidase. Curr Genet. 1994 Jul;26(1):1–7. PubMed PMID: 7954890; eng.
- Baxter SM, Rosenblum JS, Knutson S, et al. Synergistic computational and experimental proteomics approaches for more accurate detection of active serine hydrolases in yeast. Mol Cell Proteomics. 2004 Mar;3(3):209–225. PubMed PMID: 14645503; eng.
- Wünschmann J, Beck A, Meyer L, et al. Phytochelatins are synthesized by two vacuolar serine carboxypeptidases in Saccharomyces cerevisiae. FEBS Lett. 2007 Apr;581(8):1681–1687. PubMed PMID: 17408619; eng.
- Parzych KR, Ariosa A, Mari M, et al. A newly characterized vacuolar serine carboxypeptidase, Atg42/Ybr139w, is required for normal vacuole function and the terminal steps of autophagy in the yeast. Mol Biol Cell. 2018 Mar. doi: 10.1091/mbc.E17-08-0516. PubMed PMID: 29514932; eng.
- Hecht KA, O’Donnell AF, Brodsky JL The proteolytic landscape of the yeast vacuole. Cell Logist. 2014 Jan;4(1):e28023. PubMed PMID: 24843828; PubMed Central PMCID: PMCPMC4022603. eng.
- Spormann DO, Heim J, Wolf DH Biogenesis of the yeast vacuole (lysosome). The precursor forms of the soluble hydrolase carboxypeptidase yscS are associated with the vacuolar membrane. J Biol Chem. 1992 Apr;267(12):8021–8029. PubMed PMID: 1569061; eng.
- Spormann DO, Heim J, Wolf DH Carboxypeptidase yscS: gene structure and function of the vacuolar enzyme. Eur J Biochem. 1991 Apr;197(2):399–405. PubMed PMID: 2026161; eng.
- Wolf DH, Weiser U Studies on a carboxypeptidase Y mutant of yeast and evidence for a second carboxypeptidase Activity. Eur J Biochem. 1977 Mar;73(2):553–556. PubMed PMID: 403073; eng.
- Yasuhara T, Nakai T, Ohashi A Aminopeptidase Y, a new aminopeptidase from Saccharomyces cerevisiae. Purification, properties, localization, and processing by protease B. J Biol Chem. 1994 May;269(18):13644–13650. PubMed PMID: 8175799; eng.
- Frey J, Röhm KH Subcellular localization and levels of aminopeptidases and dipeptidase in Saccharomyces cerevisiae. Biochim Biophys Acta. 1978 Nov;527(1):31–41. PubMed PMID: 363165; eng.
- Metz G, Röhm KH Yeast aminopeptidase I. Chemical composition and catalytic properties. Biochim Biophys Acta. 1976 May;429(3):933–949. PubMed PMID: 5147; eng.
- Trumbly RJ, Bradley G Isolation and characterization of aminopeptidase mutants of Saccharomyces cerevisiae. J Bacteriol. 1983 Oct;156(1):36–48. PubMed PMID: 6352682; PubMed Central PMCID: PMCPMC215048. eng.
- Adamis PD, Mannarino SC, Riger CJ, et al. Lap4, a vacuolar aminopeptidase I, is involved in cadmium-glutathione metabolism. Biometals. 2009 Apr;22(2):243–249. PubMed PMID: 18716881; eng.
- Wilk S, Wilk E, Magnusson RP Purification, characterization, and cloning of a cytosolic aspartyl aminopeptidase. J Biol Chem. 1998 Jun;273(26):15961–15970. PubMed PMID: 9632644; eng.
- Yokoyama R, Kawasaki H, Hirano H. Identification of yeast aspartyl aminopeptidase gene by purifying and characterizing its product from yeast cells. FEBS J. 2006 Jan;273(1):192–198. PubMed PMID: 16367759; eng.
- Yuga M, Gomi K, Klionsky DJ, et al. Aspartyl aminopeptidase is imported from the cytoplasm to the vacuole by selective autophagy in Saccharomyces cerevisiae. J Biol Chem. 2011 Apr;286(15):13704–13713. PubMed PMID: 21343297; PubMed Central PMCID: PMCPMC3075714. eng.
- Roberts CJ, Pohlig G, Rothman JH, et al. Structure, biosynthesis, and localization of dipeptidyl aminopeptidase B, an integral membrane glycoprotein of the yeast vacuole. J Cell Biol. 1989 Apr;108(4):1363–1373. PubMed PMID: 2647766; PubMed Central PMCID: PMCPMC2115513. eng.
- Fuller RS, Sterne RE, Thorner J Enzymes required for yeast prohormone processing. Annu Rev Physiol. 1988;50:345–362. doi: 10.1146/annurev.ph.50.030188.002021. PubMed PMID: 3288097; eng.
- Johnston HD, Foote C, Santeford A, et al. Golgi-to-late endosome trafficking of the yeast pheromone processing enzyme Ste13p is regulated by a phosphorylation site in its cytosolic domain. Mol Biol Cell. 2005 Mar;16(3):1456–1468. PubMed PMID: 15647379; PubMed Central PMCID: PMCPMC551507. eng.
- Gasch AP, Spellman PT, Kao CM, et al. Genomic expression programs in the response of yeast cells to environmental changes. Mol Biol Cell. 2000 Dec;11(12):4241–4257. PubMed PMID: 11102521; PubMed Central PMCID: PMCPMC15070. eng.
- Scherens B, Feller A, Vierendeels F, et al. Identification of direct and indirect targets of the Gln3 and Gat1 activators by transcriptional profiling in response to nitrogen availability in the short and long term. FEMS Yeast Res. 2006 Aug;6(5):777–791. PubMed PMID: 16879428; eng.
- Rupp S, Hirsch HH, Wolf DH Biogenesis of the yeast vacuole (lysosome). Active site mutation in the vacuolar aspartate proteinase yscA blocks maturation of vacuolar proteinases. FEBS Lett. 1991 Nov; 293 (1–2): 62–66. PubMed PMID: 1959673; eng.
- Nakamura N, Matsuura A, Wada Y, et al. Acidification of vacuoles is required for autophagic degradation in the yeast, Saccharomyces cerevisiae. J Biochem. 1997 Feb;121(2):338–344. PubMed PMID: 9089409; eng.
- Kane PM The where, when, and how of organelle acidification by the yeast vacuolar H+-ATPase. Microbiol Mol Biol Rev. 2006 Mar;70(1):177–191. doi: 10.1128/MMBR.70.1.177-191.2006. PubMed PMID: 16524922; PubMed Central PMCID: PMCPMC1393255. eng.
- Sørensen SO, van Den Hazel HB, Kielland-Brandt MC, et al. pH-dependent processing of yeast procarboxypeptidase Y by proteinase A in vivo and in vitro. Eur J Biochem. 1994 Feb;220(1):19–27. PubMed PMID: 8119286; eng.
- Yamashiro CT, Kane PM, Wolczyk DF, et al. Role of vacuolar acidification in protein sorting and zymogen activation: a genetic analysis of the yeast vacuolar proton-translocating ATPase. Mol Cell Biol. 1990 Jul;10(7):3737–3749. PubMed PMID: 2141385; PubMed Central PMCID: PMCPMC360825. eng.
- Mechler B, Hirsch HH, Müller H, et al. Biogenesis of the yeast lysosome (vacuole): biosynthesis and maturation of proteinase yscB. Embo J. 1988 Jun;7(6):1705–1710. PubMed PMID: 3049073; PubMed Central PMCID: PMCPMC457157. eng.
- Moehle CM, Dixon CK, Jones EW Processing pathway for protease B of Saccharomyces cerevisiae. J Cell Biol. 1989 Feb;108(2):309–325. PubMed PMID: 2645294; PubMed Central PMCID: PMCPMC2115423. eng.
- Nebes VL, Jones EW Activation of the proteinase B precursor of the yeast Saccharomyces cerevisiae by autocatalysis and by an internal sequence. J Biol Chem. 1991 Dec;266(34):22851–22857. PubMed PMID: 1744078; eng.
- Hemmings BA, Zubenko GS, Hasilik A, et al. Mutant defective in processing of an enzyme located in the lysosome-like vacuole of Saccharomyces cerevisiae. Proc Natl Acad Sci U S A. 1981 Jan;78(1):435–439. PubMed PMID: 7017716; PubMed Central PMCID: PMCPMC319068. eng.
- Mechler B, Müller H, Wolf DH Maturation of vacuolar (lysosomal) enzymes in yeast: proteinase yscA and proteinase yscB are catalysts of the processing and activation event of carboxypeptidase yscY. Embo J. 1987 Jul;6(7):2157–2163. PubMed PMID: 3308453; PubMed Central PMCID: PMCPMC553608. eng.
- Klionsky DJ, Emr SD Membrane protein sorting: biosynthesis, transport and processing of yeast vacuolar alkaline phosphatase. Embo J. 1989 Aug;8(8):2241–2250. PubMed PMID: 2676517; PubMed Central PMCID: PMCPMC401154. eng.
- Merz AJ, Wickner WT Resolution of organelle docking and fusion kinetics in a cell-free assay. Proc Natl Acad Sci U S A. 2004 Aug;101(32):11548–11553. PubMed PMID: 15286284; PubMed Central PMCID: PMCPMC511018. eng.
- Steinfeld R, Reinhardt K, Schreiber K, et al. Cathepsin D deficiency is associated with a human neurodegenerative disorder. Am J Hum Genet. 2006 Jun;78(6):988–998. PubMed PMID: 16685649; PubMed Central PMCID: PMCPMC1474096. eng.
- Hiraiwa M Cathepsin A/protective protein: an unusual lysosomal multifunctional protein. Cell Mol Life Sci. 1999 Dec; 56 (11–12): 894–907. doi: 10.1007/s000180050482. PubMed PMID: 11212324; eng.
- Kaminskyy V, Zhivotovsky B Proteases in autophagy. Biochim Biophys Acta. 2012 Jan;1824(1):44–50. PubMed PMID: 21640203; eng.
- Turk B, Turk D, Turk V. Lysosomal cysteine proteases: more than scavengers. Biochim Biophys Acta. 2000 Mar; 1477 (1–2): 98–111. PubMed PMID: 10708852; eng.
- Bordallo J, Bordallo C, Gascón S, et al. Molecular cloning and sequencing of genomic DNA encoding yeast vacuolar carboxypeptidase yscS. FEBS Lett. 1991 May;283(1):27–32. PubMed PMID: 1709881; eng.
- Klionsky DJ, Cueva R, Yaver DS Aminopeptidase I of Saccharomyces cerevisiae is localized to the vacuole independent of the secretory pathway. J Cell Biol. 1992 Oct;119(2):287–299. PubMed PMID: 1400574; PubMed Central PMCID: PMCPMC2289658. eng.
- Seguí-Real B, Martinez M, Sandoval IV Yeast aminopeptidase I is post-translationally sorted from the cytosol to the vacuole by a mechanism mediated by its bipartite N-terminal extension. Embo J. 1995 Nov;14(22):5476–5484. PubMed PMID: 8521804; PubMed Central PMCID: PMCPMC394661. eng.
- Andrei-Selmer C, Knuppel A, Satyanarayana C, et al. A new class of mutants deficient in dodecamerization of aminopeptidase 1 and vacuolar transport. J Biol Chem. 2001 Apr;276(15):11606–11614. PubMed PMID: 11152450; eng.
- Meister A, Me A. Glutathione. Annu Rev Biochem. 1983;52:711–760. PubMed PMID: 6137189; eng.
- Penninckx MJ, Elskens MT Metabolism and functions of glutathione in micro-organisms. Adv Microb Physiol. 1993;34:239–301. PubMed PMID: 8095770; eng.
- Jaspers CJ, Gigot D, Penninckx MJ Pathways of glutathione degradation in the yeast Saccharomyces Cerevisiae. Phytochemistry. 1985;24(4):703–707.
- Penninckx MJ, Jaspers CJ Molecular and kinetic properties of purified γ-glutamyl transpeptidase from yeast (Saccharomyces cerevisiae). Phytochemistry. 1985;24(9):1913–1918.
- Jaspers CJ, Penninckx MJ Glutathione metabolism in yeast Saccharomyces cerevisiae. Evidence that gamma-glutamyltranspeptidase is a vacuolar enzyme. Biochimie. 1984 Jan;66(1):71–74. PubMed PMID: 6143574; eng.
- Mehdi K, Thierie J, Penninckx MJ gamma-Glutamyl transpeptidase in the yeast Saccharomyces cerevisiae and its role in the vacuolar transport and metabolism of glutathione. Biochem J. 2001 Nov;359(Pt 3):631–637. PubMed PMID: 11672438; PubMed Central PMCID: PMCPMC1222185. eng.
- Beck A, Lendzian K, Oven M, et al. Phytochelatin synthase catalyzes key step in turnover of glutathione conjugates. Phytochemistry. 2003 Feb;62(3):423–431. PubMed PMID: 12620355; eng.
- Blum R, Beck A, Korte A, et al. Function of phytochelatin synthase in catabolism of glutathione-conjugates. Plant J. 2007 Feb;49(4):740–749. PubMed PMID: 17253989; eng.
- Grzam A, Tennstedt P, Clemens S, et al. Vacuolar sequestration of glutathione S-conjugates outcompetes a possible degradation of the glutathione moiety by phytochelatin synthase. FEBS Lett. 2006 Nov;580(27):6384–6390. PubMed PMID: 17097087; eng.
- Wünschmann J, Krajewski M, Letzel T, et al. Dissection of glutathione conjugate turnover in yeast. Phytochemistry. 2010 Jan;71(1):54–61. PubMed PMID: 19897216; eng.
- Mehdi K, Penninckx MJ An important role for glutathione and gamma-glutamyltranspeptidase in the supply of growth requirements during nitrogen starvation of the yeast Saccharomyces cerevisiae. Microbiology. 1997 Jun;143 (Pt 6):1885–1889. PubMed PMID: 9202464; eng.
- Springael JY, Penninckx MJ Nitrogen-source regulation of yeast gamma-glutamyl transpeptidase synthesis involves the regulatory network including the GATA zinc-finger factors Gln3, Nil1/Gat1 and Gzf3. Biochem J. 2003 Apr;371(Pt 2):589–595. PubMed PMID: 12529169; PubMed Central PMCID: PMCPMC1223296. eng.
- Elskens MT, Jaspers CJ, Penninckx MJ Glutathione as an endogenous sulphur source in the yeast Saccharomyces cerevisiae. J Gen Microbiol. 1991 Mar;137(3):637–644. PubMed PMID: 1674526; eng.
- Deffieu M, Bhatia-Kissová I, Salin B, et al. Glutathione participates in the regulation of mitophagy in yeast. J Biol Chem. 2009 May;284(22):14828–14837. PubMed PMID: 19366696; PubMed Central PMCID: PMCPMC2685664. eng.
- Li ZS, Szczypka M, Lu YP, et al. The yeast cadmium factor protein (YCF1) is a vacuolar glutathione S-conjugate pump. J Biol Chem. 1996 Mar;271(11):6509–6517. PubMed PMID: 8626454; eng.
- Szczypka MS, Wemmie JA, Moye-Rowley WS, et al. A yeast metal resistance protein similar to human cystic fibrosis transmembrane conductance regulator (CFTR) and multidrug resistance-associated protein. J Biol Chem. 1994 Sep;269(36):22853–22857. PubMed PMID: 7521334; eng.
- Klein M, Ym M, Eggmann T, et al. The ATP-binding cassette (ABC) transporter Bpt1p mediates vacuolar sequestration of glutathione conjugates in yeast. FEBS Lett. 2002 Jun; 520 (1–3): 63–67. PubMed PMID: 12044871; eng.
- Rebbeor JF, Connolly GC, Dumont ME, et al. ATP-dependent transport of reduced glutathione on YCF1, the yeast orthologue of mammalian multidrug resistance associated proteins. J Biol Chem. 1998 Dec;273(50):33449–33454. PubMed PMID: 9837923; eng.
- Sharma KG, Mason DL, Liu G, et al. Localization, regulation, and substrate transport properties of Bpt1p, a Saccharomyces cerevisiae MRP-type ABC transporter. Eukaryot Cell. 2002 Jun;1(3):391–400. PubMed PMID: 12455987; PubMed Central PMCID: PMCPMC118024. eng.
- Ketterer S, Gomez-Auli A, Hillebrand LE, et al. Inherited diseases caused by mutations in cathepsin protease genes. FEBS J. 2017 May;284(10):1437–1454. PubMed PMID: 27926992; eng.
- Mole SE, Williams RE, Goebel HH Correlations between genotype, ultrastructural morphology and clinical phenotype in the neuronal ceroid lipofuscinoses. Neurogenetics. 2005 Sep;6(3):107–126. PubMed PMID: 15965709; eng.
- Mole SE, Cotman SL Genetics of the neuronal ceroid lipofuscinoses (Batten disease). Biochim Biophys Acta. 2015 Oct;1852(10 Pt B):2237–2241. PubMed PMID: 26026925; PubMed Central PMCID: PMCPMC4567481. eng.
- Parr CL, Keates RA, Bryksa BC, et al. The structure and function of Saccharomyces cerevisiae proteinase A. Yeast. 2007 Jun;24(6):467–480. PubMed PMID: 17447722; eng.
- Barohn RJ, Dowd DC, Kagan-Hallet KS Congenital ceroid-lipofuscinosis. Pediatr Neurol. 1992 Jan-Feb;8(1):54–59. PubMed PMID: 1558577; eng.
- Potier M, Michaud L, Tranchemontagne J, et al. Structure of the lysosomal neuraminidase-beta-galactosidase-carboxypeptidase multienzymic complex. Biochem J. 1990 Apr;267(1):197–202. PubMed PMID: 2109603; PubMed Central PMCID: PMCPMC1131264. eng.
- Bonten EJ, Annunziata I, d’Azzo A. Lysosomal multienzyme complex: pros and cons of working together. Cell Mol Life Sci. 2014 Jun;71(11):2017–2032. PubMed PMID: 24337808; PubMed Central PMCID: PMCPMC4037752. eng.
- Maroteaux P, Lamy M [Pyknodysostosis]. Presse Med. 1962 Apr;70:999–1002. PubMed PMID: 14470123; fre.
- Andren L, Dymling JF, Hogeman KE, et al. Osteopetrosis acro-osteolytica. A syndrome of osteopetrosis, acro-osteolysis and open sutures of the skull. Acta Chir Scand. 1962 Dec;124:496–507. PubMed PMID: 14041042; eng.
- Sreeramulu B, Shyam ND, Ajay P, et al. Papillon-Lefèvre syndrome: clinical presentation and management options. Clin Cosmet Investig Dent. 2015;7:75–81. PubMed PMID: 26203280; PubMed Central PMCID: PMCPMC4507741. eng.
- Gorlin RJ, Sedano H, Anderson VE The syndrome of palmar-plantar hyperkeratosis and premature periodontal destruction of the teeth. A clinical and genetic analysis of the Papillon-Lefèvre syndrome. J Pediatr. 1964 Dec;65:895–908. PubMed PMID: 14244097; eng.
- Haneke E The papillon-lefèvre syndrome: keratosis palmoplantaris with periodontopathy. Report of a case and review of the cases in the literature. Hum Genet. 1979 Sep;51(1):1–35. PubMed PMID: 159254; eng.
- Sekito T, Fujiki Y, Ohsumi Y, et al. Novel families of vacuolar amino acid transporters. IUBMB Life. 2008 Aug;60(8):519–525. PubMed PMID: 18459165; eng.
- Russnak R, Konczal D, McIntire SL A family of yeast proteins mediating bidirectional vacuolar amino acid transport. J Biol Chem. 2001 Jun;276(26):23849–23857. PubMed PMID: 11274162; eng.
- Chardwiriyapreecha S, Mukaiyama H, Sekito T, et al. Avt5p is required for vacuolar uptake of amino acids in the fission yeast Schizosaccharomyces pombe. FEBS Lett. 2010 Jun;584(11):2339–2345. PubMed PMID: 20388511; eng.
- Sekito T, Chardwiriyapreecha S, Sugimoto N, et al. Vacuolar transporter Avt4 is involved in excretion of basic amino acids from the vacuoles of Saccharomyces cerevisiae. Biosci Biotechnol Biochem. 2014;78(6):969–975. PubMed PMID: 25036121; eng.
- Nishida I, Watanabe D, Tsolmonbaatar A, et al. Vacuolar amino acid transporters upregulated by exogenous proline and involved in cellular localization of proline in Saccharomyces cerevisiae. J Gen Appl Microbiol. 2016 Jul;62(3):132–139. PubMed PMID: 27246536; eng.
- Tone J, Yamanaka A, Manabe K, et al. A vacuolar membrane protein Avt7p is involved in transport of amino acid and spore formation in Saccharomyces cerevisiae. Biosci Biotechnol Biochem. 2015;79(2):190–195. doi: 10.1080/09168451.2014.963501. PubMed PMID: 25266154; eng.
- Yang Z, Huang J, Geng J, et al. Atg22 recycles amino acids to link the degradative and recycling functions of autophagy. Mol Biol Cell. 2006 Dec;17(12):5094–5104. PubMed PMID: 17021250; PubMed Central PMCID: PMCPMC1679675. eng.
- Kalatzis V, Cherqui S, Antignac C, et al. Cystinosin, the protein defective in cystinosis, is a H(+)-driven lysosomal cystine transporter. Embo J. 2001 Nov;20(21):5940–5949. PubMed PMID: 11689434; PubMed Central PMCID: PMCPMC125690. eng.
- Gao XD, Wang J, Keppler-Ross S, et al. ERS1 encodes a functional homologue of the human lysosomal cystine transporter. FEBS J. 2005 May;272(10):2497–2511. PubMed PMID: 15885099; eng.
- Simpkins JA, Rickel KE, Madeo M, et al. Disruption of a cystine transporter downregulates expression of genes involved in sulfur regulation and cellular respiration. Biol Open. 2016 Jun;5(6):689–697. PubMed PMID: 27142334; PubMed Central PMCID: PMCPMC4920189. eng.
- Jézégou A, Llinares E, Anne C, et al. Heptahelical protein PQLC2 is a lysosomal cationic amino acid exporter underlying the action of cysteamine in cystinosis therapy. Proc Natl Acad Sci U S A. 2012 Dec;109(50):E3434–E43. PubMed PMID: 23169667; PubMed Central PMCID: PMCPMC3528584. eng.
- Onodera J, Ohsumi Y Autophagy is required for maintenance of amino acid levels and protein synthesis under nitrogen starvation. J Biol Chem. 2005 Sep;280(36):31582–31586. PubMed PMID: 16027116; eng.
- Elmonem MA, Veys KR, Soliman NA, et al. Cystinosis: a review. Orphanet J Rare Dis. 2016 Apr;11:47. doi: 10.1186/s13023-016-0426-y. PubMed PMID: 27102039; PubMed Central PMCID: PMCPMC4841061. eng.
- The UniProt Consortium. UniProt: the universal protein knowledgebase. Nucleic Acids Res. 2017 Jan;45(D1):D158–D169. PubMed PMID: 27899622; PubMed Central PMCID: PMCPMC5210571. eng.
- Hecht KA, Wytiaz VA, Ast T, et al. Characterization of an M28 metalloprotease family member residing in the yeast vacuole. FEMS Yeast Res. 2013 Aug;13(5):471–484. PubMed PMID: 23679341; PubMed Central PMCID: PMCPMC3708649. eng.
- Finn RD, Attwood TK, Babbitt PC, et al. InterPro in 2017-beyond protein family and domain annotations. Nucleic Acids Res. 2017 Jan;45(D1):D190–D199. PubMed PMID: 27899635; PubMed Central PMCID: PMCPMC5210578. eng.