Abstract
Three methacrylated glycomonomers (CMA-1–3) were synthesized, characterized, and photocrosslinked in hydrophilic conetworks. The UV photobehavior of monomers was evaluated through FTIR spectrometry using Irgacure 819/Irgacure 2959 as photoinitiator, and the results showed that Irgacure 2959 is a better photoinitiator, the conversion degree (DC) varying in the range of 69–89.2% and the maximum rate of polymerization () being ~10 s−1. The addition of poly(ethylene glycol) dimethacrylate (PEG-DMA) or benzophenone macromer (BP-UDMA) as co-monomer improved the
and DC (up to 100% after 300 s of irradiation in the latter). Specific analysis (gel fraction, water uptake, morphology) of the photopolymerized networks A1–A3 (CMA-1–3/PEG-DMA) and B1–B3 (CMA-1–3/BP-UDMA) demonstrated that B1–B3 films have interconnected inner pores, with irregular shapes and different sizes. The grid structures created through the two-photon polymerization of B1–B3 formulations in the presence of Irg819 are clearer and have a controlled porosity, of relevance for promising bioapplications.
1. Introduction
Currently, polymeric materials have become an important component of the human daily life activities, and this ‘plastics-dependence’ of the modern society stimulated the continuing effort for developing environmentally responsible products.[Citation1] This aspect together with the global awareness on the fast diminution of some natural resources produced a growing concern for recycling all over the world. So, scientists and governmental agencies in different countries are focused on the renewable feedstock in order to be used in the design of more sustainable polymeric materials. In recent years, it was noticed a real explosion in the number of studies related to the conception of new bio-based materials derived from biomass, which are widely used in a variety of areas (biomaterials, biodegradable drug delivery systems, coatings, microelectronics or optoelectronics).[Citation2–4] Biomass deposits (plants, microorganisms, fungi, marine organisms and animals) represent an easily accessible and a cheap renewable source of carbohydrate compounds as a mixture of polysaccharides (starch, cellulose, chitosan), oligosaccharides (sucrose, maltose, lactose), and monosaccharide (fructose, glucose, mannose, galactose). Thus, the selection, modification, and elaboration of new materials based on biodegradable and biocompatible carbohydrates for various applications proved to be a viable alternative for the human needs. Synthetic or natural polysaccharides were used in advanced applications, as medical devices, water absorbents, chromatographic supports and so on.[Citation5–7] Moreover, the carbohydrate-bearing polymers, especially synthetic polymers with pendant sugar moieties (known as glycopolymers), have found applications mostly in biomedicine and as biomaterials.[Citation8–11] Therefore, there have been many studies on the preparation of glycopolymers with a large diversity of architectures,[Citation12–15] using different approaches like conventional and controlled radical polymerization, living anionic polymerization, cyanoxyl-mediated polymerization, ring opening polymerization and post-polymerization modification. A classic example is the obtaining of methacrylic glycomonomers with hydroxyl protected groups, such as 3-O-methacryloyl-1,2:3,4-di-O-isopropylidene-d-galactopyranose,[Citation16–18] but the proposed methods are cumbersome and involve toxic solvents. To the best of our knowledge, the methacrylic glycomonomers have been used in conventional free radical polymerization,[Citation17] ATRP [Citation19,20] and RAFT [Citation21] polymerization.
A topic of interest, but less investigated consists in the employing of glycomonomers in the photocuring process. The photopolymerization reaction induced by UV or laser radiation represents an environmentally friendly procedure without pollutant emission such as volatile organic compounds, which can be conducted at room temperature without any degradation of sensitive molecules, and that can be easily controlled by simply turn a light on and off.[Citation22,23] However, the network formation occurs in a very short time (seconds or minutes) and does not require expensive devices. To prepare photocurable materials derived from poly-/oligo or monosaccharides, it is necessary to insert photocrosslinkable functional groups (for instance, (meth)acrylates) onto saccharide skeletons. There are some reports referring to (meth)acrylated polysaccharides (hyaluronan, dextran, heparin, chondroitin sulfate, chitosan, cellulose) [Citation24–26] and mono/oligosaccharides (glucose, sucrose, galactose) [Citation27–30] cured by the UV/Vis photocuring process, and mainly exploited in biomedical applications such as drug delivery, tissue engineering, specific recognition, and antifouling coatings. Besides, cosmetic applications for the methacrylated derivatives of sucrose and sefose (a sucrose completely modified with fatty acid chains) were found in the achievement of human fingernails under UV-light.[Citation31]
On the other hand, the use of the UV/Vis irradiation can cause a normal polymerization through single-photon (1PP) absorption, but this planar process is limited to the surface of the photopolymerizable formulation. Inducing a two-photon absorption by femtosecond (fs) laser pulses, the polymerization within the volume of the resin with the formation of 3D structures occurs, the process being thus labeled as the two-photon polymerization (2PP).[Citation32,33] In fact, the 2PP represents a rapid prototyping technology and according to Waterman et al., this type of technology may reduce the time to market by 90% and costs by up to 70%.[Citation34] Owing to the versatility of the process, the 2PP technique was useful in the fields of photonics,[Citation35] microfluidics,[Citation36] and biological tissue engineering.[Citation37] The application of a locally controlled polymerization (2PP) allows the creation of precisely defined structures, which could not be generated by means of traditional photolithography approaches. This advantage can be exploited in the fabrication of high-resolution 3D biomimetic structures (filamentous substrates for cardiac tissue, trabecular-like scaffolds, bio-inspired artificial blood vessels, and other bioapplications).[Citation38–41] Generally, the photocurable materials for 2PP are acrylates and epoxy derivatives based on poly(ethylene glycol) and organically modified silicates.[Citation42–44] Recently, scaffolds containing acrylated polysaccharides, namely hyaluronan–glycidyl methacrylate conjugate fabricated through the 2PP were reported.[Citation45] Since the hyaluronic acid-based materials are rather expensive, the research in the field has to be directed toward the development of new and cheaper materials that might mimic those from living systems. Therefore, it is interesting to extend the studies to other materials which can offer a similar response to those of the in vivo microenvironments. Taking into account that one of the greatest challenges of our times is to yield materials through non-polluting technologies, the purpose of this work is to enlarge the family of photoactive carbohydrates employing an eco-friendly synthesis method which may be easily applied for large-scale production. In the present work, the synthesis of photopolymerizable methacrylates from monosaccharide derivatives (glucofuranose, galactopyranose, mannitol) is reported in order to investigate their behavior in the photopolymerization/copolymerization reactions under exposure of UV-light in the presence of Irgacure 819 or Irgacure 2959. Also, a preliminary analysis of the two-photon polymerization process of the above structures was carried out using femtosecond laser pulses (wavelength of 775 nm).
2. Experimental
2.1. Materials
2-Isocyanatoethyl methacrylate (IEMA), 1,2:5,6-di-O-isopropylidene-α-d-glucofuranose, 1,2:3,4-di-O-isopropylidene-α-d-galactopyranose, 1,2:5,6-di-O-isopropylidene-d-mannitol, poly(ethylene glycol) dimethacrylate (PEG-DMA, Mn = 550), benzophenone-3,3′,4,4′-tetracarboxylic dianhydride, poly(ethylene glycol) (PEG, Mw = 400 g mol−1), phenyl-bis(2,4,6-trimethylbenzoyl) phosphine oxide (Irgacure 819), 2-hydroxy-1-[4-(2-hydroxyethoxy) phenyl]-2-methyl-1-propanone (Irgacure 2959) were used as received (from Sigma-Aldrich Chemical).
2.2. Synthesis
The low molecular weight monomethacrylated carbohydrates CMA-1 and CMA-2 (Scheme ) were prepared by an addition reaction following the same synthetic route and therefore, here is described only the preparation of 3-O-methacryloyloxyethylcarbamoyl-1,2:5,6-di-O-isopropylidene-α-d-glucofuranose (CMA-1): a solution of IEMA (3.4 mL, 23.06 mmol) in methylene chloride was dropwise added to a solution of 1,2:5,6-di-O-isopropylidene-α-d-glucofuranose (6 g, 23.06 mmol) in a mixture of methylene chloride and tetrahydrofuran (THF). The reaction mixture was stirred at 37 °C for 48 h in the presence of dibutyltin dilaurate used as catalyst. The reaction course was followed by infrared spectroscopy, the reaction being considered complete after the disappearance of the NCO band (2260 cm−1) from the FTIR spectrum. Using 1,2:3,4-di-O-isopropylidene-α-d-galactopyranose, 6-O-methacryloyloxyethylcarbamoyl-1,2:3,4-di-O-isopropylidene-d-galactopyranose (CMA-2) was also synthesized. For the preparation of dimethacrylated carbohydrate CMA-3 (3,4-di-O-methacryloyloxyethylcarbamoyl-1,2:5,6-di-O-isopropylidene-d-mannitol), an identical pathway was pursued, the quantities added in reaction being 5.6 mL (38.12 mmol) IEMA and 5 g (19.06 mmol) 1,2:5,6-di-O-isopropylidene-d-mannitol. Finally, the solvents were evaporated under vacuum and the glycomonomers were collected.
CMA-1. Yield: 9 g (93.9%); FTIR (KBr, cm−1): 3350(N–H), 2987–2893 (C–H), 1715 (C=O), 1637, and 815 (CH2=C), 1525 (amide II), 1460 (amide I), 1365 (C(CH3)3 linked to isopropylidene), 1165 (C–O–C); 1H NMR (400.13 MHz, CDCl3, δ ppm): 6.13 (s, 1H, CH2=C in trans position relative to CH3 unit), 5.85 (d, 1H, H1 from glucofuranose) 5.6 (s, 1H, CH2=C in cis position relative to CH3 unit), 5.41 (m, 1H, NH unit), 5.17 (s, 1H, H3 from glucofuranose), 4.56 (d, 1H, H2 from glucofuranose), 4.24 (m, 4H, CH2–O–CO and H6), 4.03 (m, 2H, H4 and H5 from glucofuranose), 3.51 (m, 2H, CH2–NH), 1.95 (s, 3H, CH3), 1.51 (d, 3H, CH3 from isopropylidene), 1.43 (d, 3H, CH3 from isopropylidene), 1.36 (d, 3H, CH3 from isopropylidene), 1.31 (d, 3H, CH3 from isopropylidene); 13C NMR (100.6 MHz, CDCl3, δ ppm): 167.1 (CH2=C–COO), 154.8 (NH–COO), 135.8 (CH2=C), 125.9 (CH2=C), 112 (C(CH3)2), 109.1 (C(CH3)2), 104.8 (CH from C1 of glucofuranose), 83.2 (CH from C2 of glucofuranose), 79.5 (CH from C4 of glucofuranose), 74.6 (CH from C3 of glucofuranose), 72.9 and 72.2 (CH2–O–CH2), 67.7 (O–CH2–CH), 63.3 (COO–CH2), 40.1 (NH–CH2), 26.7–25 (CH3 from isopropylidene), 18.1 (CH3).
CMA-2. Yield: 9.16 g (95.6%). FTIR (KBr, cm−1): 3350(N–H), 2995–2880 (C–H), 1727 (C=O), 1637, and 815 (CH2=C), 1530 (amide II), 1465 (amide I), 1380 (C(CH3)3 linked to isopropylidene), 1170 (C–O–C); 1H NMR (400.13 MHz, CDCl3, δ ppm): 6.12 (s, 1H, CH2=C in trans position relative to CH3), 5.59 (s, 1H, CH2=C in cis position relative to CH3), 5.52 (d, 1H, H1 from galactopyranose), 5.49 (m, 1H, NH unit), 4.61 (m, 1H, H2 from galactopyranose), 4.32 (m, 2H, H3 and H5 from galactopyranose), 4.25 (m, 2H, CH2–O–CO), 4.04 (d, 1H, H4 from galactopyranose), 3.48 (m, 2H, CH2–NH), 1.94 (s, 3H, CH3), 1.5 (d, 3H, CH3 from isopropylidene), 1.44 (d, 3H, CH3 from isopropylidene), 1.33 (d, 3H, CH3 from isopropylidene), 1.32 (d, 3H, CH3 from isopropylidene); 13C NMR (100.6 MHz, CDCl3, δ ppm): 166.2 (CH2=C–COO), 156.1 (NH–COO), 135.8 (CH2=C), 125.9 (CH2=C), 109.2 (C(CH3)2), 108.4 (C(CH3)2), 95.9 (CH from C1 of galactopyranose), 71.1 (CH from C4 of galactopyranose), 70.8 (CH from C3 of galactopyranose), 70.4 (CH from C2 of galactopyranose), 68 (CH from C5 of galactopyranose), 63.7 (COO–CH2), 39.7 (NH–CH2), 25.7–24.1 (CH3 from isopropylidene), 17.9 (CH3).
CMA-3. Yield: 10.2 g (93.5%). FTIR (KBr, cm−1): 3356 cm−1 (N–H), 2989–2898 cm−1 (C–H), 1721 cm−1 (C=O), 1538 cm−1 (amide II), 1455 cm−1 (amide I), 1370 cm−1 (C(CH3)3 from isopropylidene), 1172 cm−1 (C–O–C in the sugar ring), and at 1638 and 816 cm−1(CH2=C–); 1H NMR (400.13 MHz, CDCl3, δ ppm): 6.12 ppm (s, 2H, CH2=C in trans configuration), 5.61 ppm (s, 2H, CH2=C in cis configuration), 5.18 ppm (m, 2H, NH), 4.22 ppm (m, 4H, CH2–O–CO, d), 3.51 ppm (m, 4H, CH2–NH, c), 1.95 ppm (s, 6H, CH3 linked to the double bond, h), 1.36 ppm (d, 12H, CH3 from isopropylidene, j); 13C NMR: 167.3 ppm (ester function near double bond), 155.44 ppm (carbon from ester–urethane), 135.92 and 126.18 ppm (carbons from double bond), 109.31 ppm (tetrasubstituted carbon from isopropylidene), 63.58 and 40.49 ppm (methylene carbons from ester and ester–urethane), 26.3 and 18.3 ppm (methyl carbons from isopropylidene and double bonds), among others (see Section 3.1).
The reaction pathway for the synthesis of benzophenone urethane macromer (BP-UDMA) and its detailed NMR analysis were described elsewhere.[Citation46]
2.3. Characterization
The structures of the photopolymerizable methacrylated carbohydrates were verified by 1H NMR, 13C NMR, and FTIR spectroscopies using a Bruker 400-MHz spectrometer and a Bruker Vertex 70 spectrometer, respectively. UV irradiation was performed using an Hg–Xe lamp (Hamamatsu Lightningcure Type LC8, Model L9588) with a light intensity of 25 mW cm−2. The polymerization kinetics was monitored by FTIR spectroscopy. The photosensitive methacrylated glycomonomers (CMA-1–3) alone or in mixture with comonomer (PEG-DMA, BP-UDMA) and photoinitiators (Irgacure 819 or Irgacure 2959, 1 wt%) were homogenized and coated manually on the KBr plate using a wire-wound applicator to yield photopolymerizable films with thickness of about 100 μm that were further irradiated with UV-light. The FT-IR absorption spectra were measured as a function of irradiation time, and the degree of conversion was determined from the differences appeared in the C=C stretching vibration at 1637 or 815 cm−1. The degree of conversion (DC, %) was calculated according to the equation below [Citation47]:
where At is the absorbance of the band at 1637/815 cm−1 in the spectrum of the sample after t time of UV-curing, and the A0 is the absorbance of nonirradiated sample. The maximum rate of polymerization was obtained from the slope of the linear part of the obtained kinetic curves, where the conversion degree was plotted as a function of irradiation time.
Rheological investigations were carried out at 25 °C using a MCR 302 Anton-Paar rheometer equipped with Peltier device for temperature control and plane–plane geometry (diameter of 25 mm). The shear sweeps tests were carried in controlled strain mode from 10−1 to 4 × 102 s−1 in order to determine the apparent viscosity (ηapp).
The gel fraction of the photopolymerized films was measured by evaluating the weight loss after 24-h extraction with chloroform at room temperature applying the next equation [Citation47]:
where wg is the weight of insoluble gel and w0 is the total weight of the initial sample.
The water uptake of the UV cured films was determined as follows: film samples (mass w0) were immersed in water at room temperature for 7 days, removed from the solution, and weighted (w1). The films were then dried to a constant mass (w2) at room temperature. The percentage of the water absorption was calculated with the formula [Citation48]:
The morphology of the photopolymerized films incorporating CMA-1–3 glycomonomers was examined by scanning electron microscopy (SEM) using a Quanta 200 scanning probe microscope, operating in low vacuum mode, at 20 kV. The images were taken from different points of the sample to check their reproducibility. To avoid destruction of the sample microstructure, the polymeric films were frozen in liquid nitrogen, then we crashed them, and the SEM investigation in cross section of the A1–A3 and B1–B3 films was performed.
The thermal stability of the polymeric films was analyzed through thermogravimetry using a MOM Budapest derivatograph. TG and DTA curves were recorded between 25 and 750 °C with a heating rate of 10 °C min−1 in air.
The setup for 2PP experiments is a home-made direct laser writing workstation coupled with a Clark CPA-2010 amplified femtosecond laser beam working at a wavelength of 775 nm, having pulse duration of 200 fs and a repetition rate of 2 kHz. The monomer mixtures were placed on an XYZ motion stage with stepper motors and Piezo Driver. The laser beam was moved on the sample using galvanic mirrors. A Z translation stage and a visualization system with CCD camera are used for precisely positioning the sample in the plane of the focused laser. Finally, the polymerized samples were developed by removing the unpolymerized material in chloroform. The surface morphology of the films obtained by 2PP was investigated using optical microscopy (Leica DM 2500 M) and SEM (Quanta 200).
3. Results and discussion
3.1. Glycomonomers synthesis
Given our interest in the field of photocured materials, methacrylated carbohydrates with 1,2,5,6 or 1,2,3,4 diisopropylidene protecting moieties (CMA-1, CMA-2, CMA-3) were prepared and further used in photopolymerization experiments in various conditions. The structural differences between glycomonomers consist in the nature of the monosaccharide units (α-d-glucofuranose, α-d-galactopyranose and d-mannitol, respectively) and the degree of methacrylation (CMA-1, CMA-2 are monomethacrylates; CMA-3 is a dimethacrylate). These monomers were prepared through a classical addition reaction starting from the corresponding carbohydrate derivates (1,2:5,6-di-O-isopropylidene-α-d-glucofuranose, 1,2:3,4-di-O-isopropylidene-α-d-galactopyranose or 1,2:5,6-di-O-isopropylidene-d-mannitol) and 2-isocyanatoethyl methacrylate (1:1 M ratio for CMA-1 and CMA-2, and 1:2 M ratio for CMA-3), the structure of the final compounds being presented in Scheme . The resulting methacrylated monosaccharides, 3-O-methacryloyloxyethylcarbamoyl-1,2:5,6-di-O-isopropylidene-α-d-glucofuranose (CMA-1), 6-O-methacryloyloxyethylcarbamoyl-1,2:3,4-di-O-isopropylidene-d-galactopyranose (CMA-2), and 3,4-di-O-methacryloyloxyethylcarbamoyl-1,2:5,6-di-O-isopropylidene-d-mannitol (CMA-3) are soluble in volatile organic solvents (THF, chloroform, methylene chloride, ethanol, and acetone) and in water. The proposed structures and purity of each monomer have been confirmed by FTIR, 1H NMR, and 13C NMR spectroscopy (see Section 2.2). For instance, Figure shows the FTIR spectra of monomers, and Figure (a) displays the 1H NMR spectrum of CMA-3, where can be observed the presence of the typical resonance signals assigned to the methyne protons (H3, H4) from mannitol linked to ester-urethane (5.18 ppm), along with the methine (H2, H5) and methylene protons (H1, H6) from mannitol (4.22 and, respectively, 3.92 ppm). The 13C NMR analysis brings a complementary proof for the CMA-3 structure. As can be seen in Figure (b), the characteristic peaks due to the methine carbons (C4, C5, C2, C3) from mannitol (74.87, 72.4 ppm) and the methylene carbons from mannitol (65.45 ppm) provide evidence for existing the diisopropylidene-mannitol moiety in the monomer structure.
3.2. Photopolymerization behavior
3.2.1. Effect of photoinitiators
A versatile and widely applied technique for the polymerization of (meth)acrylates is their exposure to UV radiation in the presence of free radical initiators, which can be described as either ‘hydrogen abstraction type’ or ‘alpha cleavage type’. The alpha cleavage initiators have usually a higher efficiency due to the generation of free radicals via a unimolecular process, while the hydrogen abstraction initiators lead to free radicals through a bimolecular process. In line with these features, we decided to pursue the photopolymerization behavior of glycomonomers upon UV-light exposure in the presence of two initiators of alpha cleavage type: Irgacure 819 (Irg819) and Irgacure 2959 (Irg2959). Irg819 (phenyl-bis-(2,4,6-trimethyl benzoyl)) is an acyl phosphine oxide photoinitiator of light-yellow color and have UV/Vis absorption bands at 295 and 370 nm. Irg2959 (2-hydroxy-1-[4-(2-hydroxyethoxy) phenyl]-2-methyl-1-propanone) is an alpha hydroxy ketone photoinitiator, which give non-yellowing radical and has UV/Vis absorption band at 276 nm. The last one is especially appropriate where low odor is required and for use in waterborne systems. Also, it should be mentioned that the active hydroxyl group can be reacted with some functionalized unsaturated resins.
In order to evaluate the UV-curing behavior of the above methacrylated monosaccharides, the disappearance of the characteristic acrylic double bond absorption bands at 815 and 1637 cm−1 after UV irradiation was monitored by the FTIR spectroscopy. In all cases, the photopolymerization kinetics for the carbohydrate monomers were studied under identical conditions: the same initiator concentration (1 wt% Irg819 or Irg2959), light intensity (25 mW cm−2) and at room temperature, the monomers conversion being appreciated from the decrease in the double bond stretching vibration at about 1637 cm−1. Indeed, the methacrylated glycomonomers are converted to free-standing thin films after their homopolymerization under the above experimental conditions. For all methacrylated monosaccharides, the intensity of absorption band of the methacrylic double bonds (at around 1637 cm−1) showed important spectral changes in the presence of Irg819 or Irg2959, as is exemplified for CMA-2 in Figure (a) and (b). However, it is important to note that the same glycomonomer acts different in the presence of the two photoinitiators: when Irg819 was employed, after 300 s of UV irradiation the degree of conversion (DC) is about 38%, meanwhile in the case of Irg2959, this parameter reached a value of 69%. To illustrate the conversion variation with the irradiation time for the methacrylated glycomonomers in the presence of these photoinitiators, the decrease in the characteristic area of the absorption bands positioned at 1637 cm−1 was measured, and the results are represented in Figure (c). Regarding the final conversion degree (after 300 s of irradiation), a similar behavior was observed for the CMA-1–3, but the values obtained in the presence of Irg2959 (DC = 69–89.2%) were higher than those for Irg819 (DC = 38.4–68.3%) (Figure (c) inset). Consequently, the DC for glycomonomers photopolymerized in the above conditions can be ordered as follows: DCCMA-2 < DCCMA-1 < DCCMA-3. This finding can be attributed to the different sizes of the sugar ring in monomers (CMA-2 has 6 atoms in the ring, and CMA-1 has 5 atoms) that may affect the mobility and packing arrangement of the photopolymerizable double bonds. Also, it can be remarked an important dependence between the degree of conversion of the mono-methacrylated compounds (DCCMA-1 = 69% and DCCMA-2 = 75%, values calculated for Irg2959) and di-methacrylate (DCCMA-3 = 89.2%, value calculated for Irg2959). Looking more carefully to the plot of conversion degree vs. exposure time [Figure (c)], it may be noticed that the course of photopolymerization is similar for both photoinitiators, the kinetic curves are partially overlapping and the induction period is absent, and therefore, the inflection point is missing. Thus, the maximum polymerization rates () for our compounds were determined from the slope of the straight-line part of the kinetic curves for the beginning stage of the photopolymerization process,[Citation47] and the results are listed in Table . For the photopolymerization of CMA-1–3 in the presence of Irg2959, the maximum polymerization rate of each monomer was approximately 10 s−1, whereas the values obtained in the presence of Irg819 were lower (4.1–8.6 s−1). On the basis of these results, one can conclude that the synthesized glycomonomers CMA-1–CMA-3 exhibited similar reactivity with other methacrylates, reason for which they are recommended to be used in photopolymerization reactions initiated by Irg2959 as photoinitiator.
Figure 3. Evolution of the double bond absorption band at 1637 cm−1 from CMA-2 monitored as a function of UV irradiation time in the presence of Irg819 (a) or Irg2959 (b); conversion versus irradiation time plot for CMA-3 in the presence of Irg819 or Irg2959 (c), and degree of conversion for CMA-1–CMA-3 after 300 s of UV irradiation (inset).
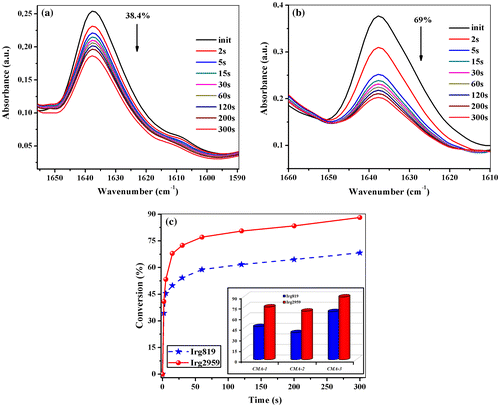
Figure 4. Evolution of the double bond absorption band at 815 cm−1 from the formulations A1 (a) and B1 (b) monitored as a function of UV irradiation time in the presence of Irg2959; and degree of conversion for the formulation A1–A3 and B1–B3 after 300 s of UV irradiation (c).
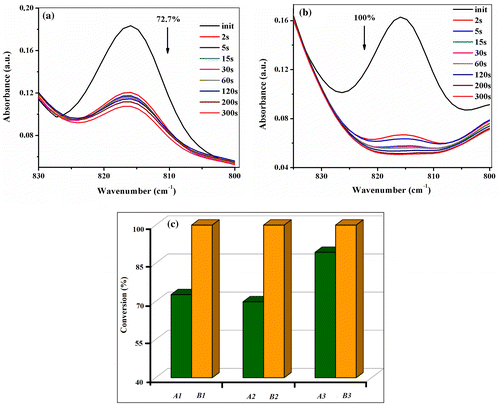
Figure 5. SEM images for the photopolymerized films A1 (CMA-1 + PEG-DMA), A2 (CMA-2 + PEG-DMA), A3 (CMA-3 + PEG-DMA), B1 (CMA-1 + BP-UDMA), B2 (CMA-2 + BP-UDMA) and B3 (CMA-3 + BP-UDMA) taken in cross section.
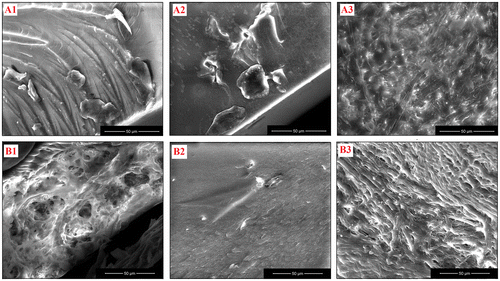
Figure 6. Microscopic images of 2PP-manufactured grid structures derived from B1 (a) and B3 (b) formulations using Irg2959 as photoinitiator.
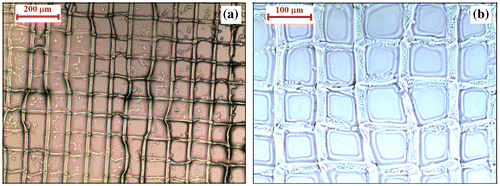
Figure 7. Microscopic (a) and SEM (b) images of 2PP-manufactured grid structures obtained from B1 to B3 formulations in the presence of Irg819.
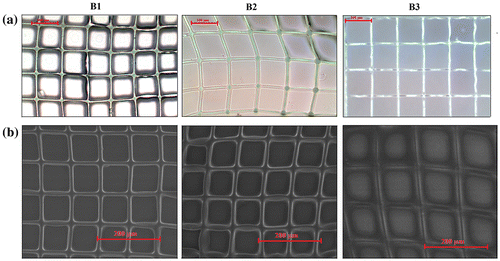
Table 1. Rate of photopolymerization for CMA-1–3 in the presence of Irg819/Irg2959.
3.2.2. Effect of comonomers
Furthermore, we investigated the photobehavior of glycomonomers in mixtures with other two dimethacrylate monomers having hydrophilic units, namely poly(ethylene glycol) dimethacrylate (PEG-DMA, Scheme ) and a benzophenone oligomer bearing acid urethane-dimethacrylate sequences, (BP-UDMA, Scheme ) [Citation46] in the presence of Irg2959. The apparent viscosities of the formulations are dependent on the monomer composition (Table ) and the highest apparent viscosity was found for the formulation containing BP-UDMA (η ~ 0.66 Pa s) as compared to that with PEG-DMA (η ~ 0.17 Pa s).
Scheme 2. Structures of the poly(ethylene glycol) dimethacrylate (PEG-DMA) and poly(ethylene oxide) dimethacrylate with benzophenone unit (BP-UDMA) used in the photopolymerization experiments.
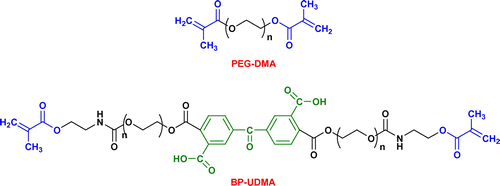
Table 2. Composition, gel fraction, and water uptake for the formulations taken in study.
Comparative evaluation of the double bond absorption band (at about 815 cm−1) of the monomer mixture CMA-1/PEG-DMA (formulation A1) [Figure (a)] showed that the degree of conversion after only 2 s of UV-curing was 60%, and the final conversion attained a value of 72.7% (after 300 s of irradiation). By the replacement of PEG-DMA with BP-UDMA – a photosensitive urethane dimethacrylate with a higher inner flexible spacer (formulation B1) the copolymerization proceed very fast in the first 2 s of irradiation [Figure (b)], the samples reaching a conversion degree over 90%. For all three formulations, the mixing of methacrylated monosaccharides with the photosensitive benzophenone macromer generated a conversion degree around 100% after 300 s of UV-curing. This result may be explained through the photoinitiator performance (Irg2959), which is improved due to the combination with the benzophenone structure from BP-UDMA, the two photosensitive units acting synergistically. This outcome is supported by the values of the polymerization rates of the formulations, the values of for the mixtures with BP-UDMA (B1–B3) being superior (17.3–18.2 s−1) to those of A1-A3 formulations (11.1–11.9 s−1) containing PEG-DMA macromer. At the same time, the variation of
as a function of the glycomonomer present in the starting mixture does not suggest important differences and they can be connected to the different size of the sugar rings in monomers and to the different degree of methacrylation from carbohydrate.
3.3. Gel fraction and water uptake of the UV cured formulations
Since it is well known that the dimethacrylates used in such reactions behave as crosslinking agents, we examined the formation of highly crosslinked networks by measuring the gel fraction in the photocured films derived from the A1–A3 and B1–B3 formulations. An insoluble gel was formed in both cases during the photopolymerization of the formulations containing PEG-DMA comonomer (A1–A3) as well as for B1–B3 mixtures (incorporating BP-UDMA). The percentage values of gel fraction after 300 s of UV irradiation are indicated in Table , and one can note that the gel content exceeds 80% in CMA-1–3/PEG-DMA formulations, whereas in the films B1–B3 (CMA-1–3 + BP-UDMA), the gel fraction is over 95%. The higher gel content in B1–B3 compared to A1–A3 formulations could be caused by the additional participation of carboxyl groups of BP-UDMA in the physical crosslinking process (H bonding). Another aspect is that the values of the gel fraction are proportional with those established for the conversion degree and the best results were obtained for A3 (97.6%) and B3 (99.7%) formulations, where the glycomonomer used in the photocuring contains two dimethacrylate units. As expected, the higher functionality of methacrylate monomer increased the crosslinking degree of polymer networks.
In order to assess the water absorption properties of the photocured films formed by the hydrophilic components, the data were collected after 7 days of water immersion of the samples A1–A3 and B1–B3, at room temperature, and the results are shown in Table . At a first look, it can be remarked that the water uptake ratio is larger for B1–B3 (6.02–7.25%) films than for A1–A3 (3.28–4.71%). This result may be attributed to the increasing hydrophilicity of the B1–B3 photocured films, where there are two hydrophilic poly(ethylene oxide) sequences between the methacrylic functionalities of BP-UDMA and the hydrophilic carboxyl groups attached to the benzophenone moieties. Moreover, it was found that the absorption ratio increased with decreasing gel fraction of the formulations (from A3 to A1 and A2, and respectively, from B3 to B1 and B2), whereas at a higher crosslinking density the water adsorption was inhibited by the more rigid network and a poorer water uptake ratio was observed.
3.4. Morphologies of the photocured films
The internal microstructures of the polymer networks formed through UV irradiation of the mixtures of glycomethacrylate (CMA-1, CMA-2, or CMA-3) and PEG-DMA/BP-UDMA were visualized by SEM. In Figure , the SEM images of the six films A1–A3 and B1–B3, in fracture are shown. For the A1–A3 formulations (with PEG-DMA macromer), the morphology of the networks is compact, not smooth, with some protrusions of different sizes. This result may indicate that the monosaccharide units of the carbohydrate derivatives formed heterogeneous domains and may produce phase separation, the phenomenon being more obvious for A1 and A2 films incorporating mono-methacrylated glycomonomers. For the A3 formulation with monosaccharide dimethacrylate, a more orderly structural organization it is observed, and the phase separation appears in smaller scale domains. The formulations based on BP-UDMA oligomer (B1–B3) displayed a porous morphology, where the shape and the size of pores depend on the nature of carbohydrate monomer. Thus, the B1 film enclosing a mono-methacrylated carbohydrate with a five atoms sugar ring has the interconnected inner pores, with irregular shapes and different pore sizes. By replacing CMA-1 with CMA-2 (B2 sample), a more uniform morphology, with fewer and smaller pores in the polymer network is observable. The use of CMA-3 dimethacrylate (B3 formulation) induced, as well as for the A3 sample, a better organized internal structure, where the distribution of porosity becomes more uniform and dense. The porous structure may favor the cells adhesion and proliferation, and so our biodegradable photopolymerized materials could be explored for their potential use as scaffolds in tissue engineering applications, but this will make the subject of an afterward study.
3.5. Thermal stability of the UV-curable polymer films
For a later inspection of the structural complexity of the photopolymerized networks, we performed the thermogravimetric analysis. From the differential thermograms of the photopolymerized formulation A1–A3 and B1–B3, the initial (Ti), the maximum (Tmax), and the final (Tf) degradation temperatures were determined, all parameters being summarized in Table . For all samples, in the first stage of degradation (200–315 °C), a mass loss ranging from 39 to 45% was found. This weight loss could be attributed to a complex process including the removal of protective groups from the monosaccharides, degradation of the sugar ring, and the breaking of ester and ester-urethane linkages from the monomer units. In the second stage of degradation (370–485 °C), the mass loss varied from 37 to 48% for A1–A3 films and from 26 to 31% for B1–B3 films, respectively. In this stage, the decomposition of the ether linkage of the PEG units of the comonomers used in formulations takes probably place. The third stage of degradation occurs between 490 and 675 °C, and the weight loss ranges from 11 to 14% for A1–A3 films, meanwhile for B1–B3, this parameter is in the range of 23–26%. This phase is characterized by the random scission of the macromolecular chain, and additionally, for B1–B3 the benzophenone moieties could be decomposed.
Table 3. Thermal parameters for UV photopolymerized formulations.
3.6. Grid structures fabrication by two-photon polymerization (2PP)
Because a variety of photosensitive materials were successfully processed by two-photon polymerization,[Citation49–51] we decided to investigate the visible light-curing behavior of binary mixtures of monomers based on CMA-1, CMA-2, or CMA-3 and BP-UDMA (B1–B3 formulations) by this procedure, the possibility of their later employment in the development of 3D scaffolds was evaluated. The two-photon polymerization experiments were performed with a femtosecond Ti-sapphire laser pulses, using different average power laser values (10–30 mW) and different scanning velocities (0.5–2 mm s−1). After performing various trials for direct femtosecond laser writing, we established that the setup optimal parameters for our 2PP experiments are 10 mW power laser and 1 mm s−1 scanning speed. Under these conditions, grid structures of 20 × 20 lines and 20 lines systems with distances between lines of 100 μm were created on glass substrates. In addition, the influence of photoinitiator (1 wt%) on the two-photon polymerization was examined. In agreement with the good results obtained in the UV photopolymerization process, firstly Irg2959 was tested for the fabrication of grid structures. The unpolymerized components were removed by washing the samples in chloroform to expose the formed structure. The optical microscopy images of the 2D structure resulted from B1 formulation based on CMA-1 and BP-UDMA [Figure (a)] revealed the presence of defects of the grid and of an intermittent polymerization process. In the case of B3 formulation containing CMA-3 and BP-UDMA dimethacrylates, the structure does not has major defects, but the photopolymerized lines seem to suggest the formation of a porous structure.
Since the laser radiation emits at around 775 nm, it is thought that the use of Irg819 is more suitable, its absorption maximum matching with the half-wavelength of the applied laser radiation.[Citation45] So, the two-photon polymerization in the presence of Irg819 was performed, and after the development in chloroform, the optical microscopy and SEM images (Figure ) show that the free-standing grid structures prepared from all formulations (B1–B3) are without defects. Hence, our new glycomonomers (CMA-1–3) could be applied in the fabrication of well-structured films by the 2PP technique for laser power values of 10 mW, working at 1 mm s−1 scanning speed and using Irg819 as photoinitiator. From this perspective, the 2PP method is ideal for the production of a series of identical structures, with defined dimensions between the polymerized lines, and if the materials processed through this technique are biocompatible and biodegradable, finally will lead to scaffolds with a well-controlled porosity and preset size of the pores.
4. Conclusions
Herein, the synthesis and characterization of photoactive urethane glycomonomers with one or two methacrylic functions on glucofuranose, galactopyranose or mannitol derivatives were reported. Following their photobehavior upon the exposure to UV-light by FTIR measurements, it was observed a good photoreactivity of the monomers in photopolymerization reactions (especially in the presence of Irg2959), which may be improved by the addition of urethane dimethacrylates as comonomers (PEG-DMA and BP-UDMA). Besides, for the 2PP experiments, Irg819 is recommended to be used as photoinitiator. The photopolymerization behavior of the glycomonomers alone or in combination with other dimethacrylates together with the results derived from each investigation may be exploited in the design of predetermined molecular architectures suitable for tissue engineering applications.
Acknowledgments
A. L. Chibac is thankful for the financial support offered by European Social Fond – ‘Doctoral and postdoctoral programs – support for increasing research competitiveness in the field of Exact Sciences’ – ID POSDRU/159/1.5/S/137750, Sectorial Operational Programme Human Resources Development.
The authors E. C. Buruiana, T. Buruiana and G. Epurescu are thankful for the financial support offered by CNCSIS-UEFISCDI, project number PN-II-PT-PCCA-2011-3.1-1422 (146/2012).
Funding
This work was financially supported by the European Social Fond – ‘Doctoral and postdoctoral programs – support for increasing research competitiveness in the field of Exact Sciences’ [grant number ID POSDRU/159/1.5/S/137750]; CNCSIS-UEFISCDI [grant number PN-II-PT-PCCA-2011-3.1-1422 (146/2012)].
Disclosure statement
No potential conflict of interest was reported by the authors.
References
- Gandini A, Lacerda TM. From monomers to polymers from renewable resources: recent advances. Prog. Polym. Sci. 2015;48:1–39. doi:10.1016/j.progpolymsci.2014.11.002.
- Lee W, Choi D, Lee Y, et al. Preparation of micropatterned hydrogel substrate via surface graft polymerization combined with photolithography for biosensor application. Sens. Actuators, B. 2008;129:841–849.10.1016/j.snb.2007.09.085
- Tokarev I, Minko S. Multiresponsive, hierarchically structured membranes: new, challenging, biomimetic materials for biosensors, controlled release, biochemical gates, and nanoreactors. Adv. Mater. 2009;21:241–247.10.1002/adma.v21:2
- Fertier L, Koleilat H, Stemmelen M, et al. The use of renewable feedstock in UV-curable materials – a new age for polymers and green chemistry. Prog. Polym. Sci. 2013;38:932–962.10.1016/j.progpolymsci.2012.12.002
- Mahanta N, Leong WY, Valiyaveettil S. Isolation and characterization of cellulose-based nanofibers for nanoparticleextraction from an aqueous environment. J. Mater. Chem. 2012;22:1985–1993.10.1039/C1JM15018A
- Autissier A, Visage C, Pouzet C, et al. Fabrication of porous polysaccharide-based scaffolds using a combined freeze-drying/cross-linking process. Acta Biomater. 2010;6:3640–3648.10.1016/j.actbio.2010.03.004
- Yin D, Wu H, Liu C, et al. Fabrication of composition-graded collagen/chitosan-polylactide scaffolds with gradient architecture and properties. React. Funct. Polym. 2014;83:98–106.10.1016/j.reactfunctpolym.2014.07.017
- Spain SG, Gibson MI, Cameron NR. Recent advances in the synthesis of well-defined glycopolymers. J. Polym. Sci., Part A: Polym. Chem. 2007;45:2059–2072.10.1002/(ISSN)1099-0518
- Bordegé V, Muñoz-Bonilla A, León O, et al. Glycopolymers with glucosamine pendant groups: copolymerization, physico-chemical and interaction properties. React. Funct. Polym. 2011;71:1–10.10.1016/j.reactfunctpolym.2010.11.010
- Killion JA, Kehoe S, Geever LM, et al. Hydrogel/bioactive glass composites for bone regeneration applications: synthesis and characterisation. Mater. Sci. Eng. C Mater. Biol. Appl. 2013;33:4203–4212.10.1016/j.msec.2013.06.013
- Abeylath SC, Turos E. Glycosylated polyacrylate nanoparticles by emulsion polymerization. Carbohydr. Polym. 2007;70:32–37.10.1016/j.carbpol.2007.02.027
- Xiao NY, Li AL, Liang H, et al. A well-defined novel aldehyde-functionalized glycopolymer: synthesis, micelle formation, and its protein immobilization. Macromolecules. 2008;41:2374–2380.10.1021/ma702510n
- Bernard J, Hao X, Davis TP, et al. Synthesis of various glycopolymer architectures via RAFT polymerization: from block copolymers to stars. Biomacromolecules. 2006;7:232–238.10.1021/bm0506086
- Pasparakis G, Alexander C. Sweet talking double hydrophilic block copolymer vesicles. Angew. Chem., Int. Ed. 2008;47:4847–4850.10.1002/(ISSN)1521-3773
- Ghadban A, Albertin L. Synthesis of glycopolymer architectures by reversible-deactivation radical polymerization. Polymers. 2013;5:431–526.10.3390/polym5020431
- Bird TP, Black WAP, Colquhoun JA, et al. Preparation and derivatives of poly-(6-O-methacryloyl-d-galactose) and poly-(6-O-acryloyl-d-galactose). J. Chem. Soc. C. 1966;1:1913–1918.10.1039/j39660001913
- Black WAP, Colquhoun JA, Dewar ET. Polymerisable monomers of 1,2:3,4-di-O-isopropylidene-α-d-galactopyranose. Carbohydr. Res. 1967;5:362–365.10.1016/S0008-6215(00)80516-9
- Carneiro MJ, Fernandes A, Figueiredo CM, et al. Synthesis of carbohydrate based polymers. Carbohydr. Polym. 2001;45:135–138.10.1016/S0144-8617(00)00322-2
- Arslan H, Zırtıl O, Bütün V. The synthesis and solution behaviors of novel amphiphilic block copolymers based on d-galactopyranose and 2-(dimethylamino)ethyl methacrylate. Eur. Polym. J. 2013;49:4118–4129.10.1016/j.eurpolymj.2013.09.018
- Chen YM, Wulff G. ABA and star amphiphilic block copolymers composed of polymethacrylate bearing a galactose fragment and poly(ɛ-caprolactone). Macromol. Rapid Commun. 2002;23:59–63.10.1002/(ISSN)1521-3927
- Lowe AB, Wang R. Synthesis of controlled-structure AB diblock copolymers of 3-O-methacryloyl-1,2:3,4-di-O-isopropylidene-d-galactopyranose and 2-(dimethylamino)ethyl methacrylate. Polymer. 2007;48:2221–2230.10.1016/j.polymer.2007.02.062
- Yagci Y, Jockusch S, Turro NJ. Photoinitiated polymerization: advances, challenges, and opportunities. Macromolecules. 2010;43:6245–6260.10.1021/ma1007545
- Chatani S, Kloxin CJ, Bowman CN. The power of light in polymer science: photochemical processes to manipulate polymer formation, structure, and properties. Polym. Chem. 2014;5:2187–2201.10.1039/C3PY01334K
- Pitarresi G, Casadei MA, Mandracchia D, et al. Photocrosslinking of dextran and polyaspartamide derivatives: a combination suitable for colon-specific drug delivery. J. Controlled Release. 2007;119:328–338.10.1016/j.jconrel.2007.03.005
- Pescosolido L, Schuurman W, Malda J, et al. Hyaluronic acid and dextran-based semi-IPN hydrogels as biomaterials for bioprinting. Biomacromolecules. 2011;12:1831–1838.10.1021/bm200178w
- Zhou Y, Yang D, Gao X, et al. Semi-interpenetrating polymer network hydrogels based on water-soluble N-carboxylethyl chitosan and photopolymerized poly (2-hydroxyethyl methacrylate). Carbohydr. Polym. 2009;75:293–298.10.1016/j.carbpol.2008.07.024
- Yang Q, Strathmann M, Rumpf A, et al. Grafted glycopolymer-based receptor mimics on polymer support for selective adhesion of bacteria. ACS Appl. Mater. Interfaces. 2010;2:3555–3562.10.1021/am1007276
- Pfaff A, Shinde VS, Lu Y, et al. Glycopolymer-grafted polystyrene nanospheres. Macromol. Biosci. 2011;11:199–210.10.1002/mabi.v11.2
- Deng C, Li F, Hackett JM, et al. Collagen and glycopolymer based hydrogel for potential corneal application. Acta Biomater. 2010;6:187–194.10.1016/j.actbio.2009.07.027
- Gu JS, Yu HY, Huang L, et al. Chain-length dependence of the antifouling characteristics of the glycopolymer-modified polypropylene membrane in an SMBR. J. Membr. Sci. 2009;326:145–152.10.1016/j.memsci.2008.09.043
- Steffier LW. UV-curable nail coating formulations based on renewable polyols. United States patent US 8,574,558. 2013.
- Wu S, Serbin J, Gu M. Two-photon polymerisation for three-dimensional micro-fabrication. J. Photochem. Photobiol., A. 2006;181:1–11.10.1016/j.jphotochem.2006.03.004
- Obata K, El-Tamer A, Koch L, et al. High-aspect 3D two-photon polymerization structuring with widened objective working range (WOW-2PP). Light: Sci. Appl. 2013;2: e116.
- Waterman NA, Dickens P. Rapid product development in the USA, Europe and Japan. World Class Des. Manuf. 1994;1:27–36.10.1108/09642369210056629
- Deubel M, Wegener M, Linden S, et al. 3D–2D–3D photonic crystal heterostructures fabricated by direct laser writing. Opt. Lett. 2006;31:805–807.10.1364/OL.31.000805
- Amato L, Gu Y, Bellini N, et al. Integrated three-dimensional filter separates nanoscale from microscale elements in a microfluidic chip. Lab Chip. 2012;12:1135–1142.10.1039/c2lc21116e
- Ovsianikov A, Gruene M, Pflaum M, et al. Laser printing of cells into 3D scaffolds. Biofabrication. 2010;2:014104.10.1088/1758-5082/2/1/014104
- Culver JC, Hoffmann JC, Poché RA, et al. Three-dimensional biomimetic patterning in hydrogels to guide cellular organization. Adv. Mater. 2012;24:2344–2348.10.1002/adma.201200395
- Marino A, Filippeschi C, Genchi GG, et al. The osteoprint: a bioinspired two-photon polymerized 3-D structure for the enhancement of bone-like cell differentiation. Acta Biomater. 2014;10:4304–4313.10.1016/j.actbio.2014.05.032
- Ma Z, Koo S, Finnegan MA, et al. Three-dimensional filamentous human diseased cardiac tissue model. Biomaterials. 2014;35:1367–1377.10.1016/j.biomaterials.2013.10.052
- Marino A, Filippeschi C, Mattoli V, et al. Biomimicry at the nanoscale: current research and perspectives of two-photon polymerization. Nanoscale. 2015;7:2841–2850.10.1039/C4NR06500J
- LaFratta CN, Fourkas JT, Baldacchini T, et al. Multiphoton fabrication. Angew. Chem. Int. Ed. 2007;46:6238–6258.10.1002/anie.v46:33
- Klein F, Richter B, Striebel T, et al. Two-component polymer scaffolds for controlled three-dimensional cell culture. Adv. Mater. 2011;23:1341–1345.10.1002/adma.v23.11
- Lee SH, Moon JJ, West JL. Three-dimensional micropatterning of bioactive hydrogels via two-photon laser scanning photolithography for guided 3D cell migration. Biomaterials. 2008;29:2962–2968.10.1016/j.biomaterials.2008.04.004
- Kufelt O, El-Tamer A, Sehring C, et al. Hyaluronic acid based materials for scaffolding via two-photon polymerization. Biomacromolecules. 2014;15:650–659.10.1021/bm401712q
- Buruiana EC, Chibac AL, Buruiana T, et al. A benzophenone-bearing acid oligodimethacrylate and its application to the preparation of silver/gold nanoparticles/polymer nanocomposites. J. Nanopart. Res. 2013;15:1335.10.1007/s11051-012-1335-1
- Kaczmarek H, Vukovic-Kwiatkowska I. Preparation and characterization of interpenetrating networks based on polyacrylates and poly(lactic acid). Express Polym. Lett. 2012;6:78–94.
- Liao W, Teng H, Qu J, et al. Fabrication of chemically bonded polyacrylate/silica hybrid films with high silicon contents by the sol-gel method. Prog. Org. Coat. 2011;71:376–383.10.1016/j.porgcoat.2011.04.008
- Schlie S, Ngezahayo A, Ovsianikov A, et al. Three-dimensional cell growth on structures fabricated from ORMOCER(R) by two-photon polymerization technique. J. Biomater. Appl. 2007;22:275–287.10.1177/0885328207077590
- Ovsianikov A, Schlie S, Ngezahayo A, et al. Two-photon polymerization technique for microfabrication of CAD-designed 3D scaffolds from commercially available photosensitive materials. J. Tissue Eng. Regener. Med. 2007;1:443–449.10.1002/(ISSN)1932-7005
- Narayan RJ, Doraiswamy A, Chrisey DB, et al. Medical prototyping using two photon polymerization. Mater. Today. 2010;13:42–48.10.1016/S1369-7021(10)70223-6