Abstract
A series of polar silicones was synthesized in order to compare their dielectric properties. Different substituents with high dipole moment (epoxy, pyridyl, aldehyde, cyano-, nitroazobenzene) were attached by hydrosilylation to a poly(dimethyl-methylhydro)siloxane. Thiol-ene addition on a dimethyl-methylvinyl siloxane copolymer with similar composition was also used for chemical modifications with chloro- or carboxy- derivatives. This approach allowed comparison of properties with emphasis on dielectric behavior measured in liquid state, as a preliminary step in design and preparation of materials suitable for dielectric elastomers. Although a relatively low content of polar groups was used (8%), permittivity values of 5.4 and even 7.4 were achieved (at 10 kHz), either due to the large dipole moment or to the presence of important amounts of moisture. The water sorption capacity of the polar silicones was investigated by dynamic vapor sorption, while structural parameters of model molecules were calculated, in order to correlate the dielectric properties with the polarity/hydrophilicity of the substituents to the silicone chain. A combined effect of the calculated dipole moment, molar polarizability, molar volume, and the measured water sorption capacity on dielectric permittivity was observed.
1. Introduction
Besides acrylics, natural rubber, and polyurethanes, silicones are intensively studied for dielectric elastomer transducers (DET).[Citation1–3] Such devices are used to inter-convert electric and mechanical energy in actuators, energy harvesters, or sensors.[Citation4,5] In this context, silicones (or polysiloxanes) are very promising, due to their specific properties, as for example: very low glass transition temperature, large free volume, unusual rheological/flow properties with highly elastic behavior, low surface energy, water repellence, resistance to oxygen, ozone and sunlight irradiation, resistance to weathering and aging, shear stability, excellent dielectric strength, low toxicity, and stability of the main properties over a wide range of temperature.[Citation6,7]
In recent years, different approaches were used in order to obtain silicone-based materials with optimized properties for DET applications. In principle, the main targets that have to be achieved are high dielectric permittivity, low dielectric losses, and balanced mechanical properties (generally low Young’s modulus and moderate tensile stress). This is a challenging task, starting from a liquid polymer (as polydimethylsiloxane – PDMS-) with very low dielectric permittivity (ε′ < 3). There are several aspects that have to be carefully addressed. One of them is the cross-linking of the liquid silicone, which can be done by several mechanisms, each of them with advantages and drawbacks.[Citation8,9] A very important aspect is the cross-linking degree, which is influenced by the molar mass of the starting PDMS or by the composition of the siloxane copolymers.[Citation10,11] This step highly affects the mechanical properties of the final materials, together with other factors, like the cross-linker or filler nature and amount, or the presence of rigid, polar groups.[Citation12,13] Another aspect is the choice of a suitable way for increasing the dielectric permittivity. This can be done by mixing PDMS with ceramic, conductive, or polarizable particles [Citation14–23] or by chemical attachment of polar groups.[Citation10,13,24–29] Although a lot of research effort has been undertaken, the optimized material is still expected.[Citation8]
Numberless polar (functional) silicones were synthesized for various applications.[Citation30–32] However, relatively few polar siloxane polymers have been treated from the viewpoint of DETs, while they were investigated in cross-linked state.[Citation13,25,27,28] In the context of DETs, it is very often difficult to compare different materials, especially due to the large diversity of structures and compositions and to different testing setups. After a literature survey, we could not find a systematic investigation or a comparative study of polysiloxanes bearing various polar groups from the perspective of meaningful properties for DET applications. Most studies on polar silicones focus on a chosen polar group attached in various proportions to the polysiloxane, while considering the dipole moment the only parameter of interest in choosing the dipole. On the other hand, it is of general knowledge that water influences the dielectric properties of materials in general, including the silicones, while in fact the real water content or water sorption capacity of specific materials is not commonly investigated, but rather anticipated in a speculative way, knowing that the unmodified polysiloxanes are highly hydrophobic.
As a step in a systematic demarche toward optimized silicone materials for DETs, in this paper we report a structure-dielectric permittivity relationship on a series of copolymers with similar content of polar groups and similar molar mass. The dielectric behavior of these copolymers was influenced, as expected, by their dipole moment and molar volume, but in certain cases anomalies were observed. As ionic conductivity might artificially increase the dielectric permittivity, while being prompted in many cases by the water uptake, we also investigated the water vapors sorption behavior. A uniform increase of the dielectric permittivity with the product of the calculated dipole moment, molar polarizability, molar volume, and the measured maximum water uptake was observed.
2. Experimental
2.1. Materials and methods
The following reagents were used as received, unless otherwise specified: octamethylcyclotetrasiloxane (D4), assay 98%, tetramethyl-tetravinylcyclotetrasiloxane (D4V), assay 97%, and tetramethylcyclotetrasiloxane (D4H), assay 99%, (from Alfa Aesar), hexamethyldisiloxane, assay 99.9%, (from ABCR), N-ethyl-N-(2-hydroxyethyl)-4-(4-nitrophenylazo)aniline (Disperse red 1), assay > 96%, 3-chloro-1-propanethiol, assay > 98%, 3-mercaptopropionic acid, assay > 99%, platinum(0)-1,3-divinyl-1,1,3,3-tetramethyldisiloxane complex solution in xylene (Pt(dvs), Karstedt catalyst), assay %Pt: 2.1–2.4%, 2,2-dimethoxy-2-phenylacetophenone (DMPA), assay 99%, 3-chloropropene (allyl chloride), assay 99%, 4-hydroxybenzaldehyde, assay 98%, 3-butenenitrile (allyl cyanide), assay 98%, 2-(prop-2-enoxymethyl)oxirane (allyl glycidyl ether), assay >99%, 4-aminopyridine, assay >99%, and anhydrous potassium carbonate, assay >99%, (from Sigma-Aldrich).
Styrenedivinylbenzene ion exchanger with –SO3H groups, commonly called Purolite CT-175, (exchange capacity 1.87 meq/mL) was purchased from Viromet S.A. and dehydrated by azeotrope distillation with toluene under vacuum at 110 °C/10 mm Hg. The solvents: dimethylsulfoxide, dichloromethane, diethyl ether, toluene, methanol, isopropyl alcohol, THF were purchased from Roth and used as received, unless otherwise mentioned.
Gel permeation chromatography (GPC) measurements of the precursors were made in chloroform on a PL-EMD 950 Chromatograph Evaporative Mass Detector using polystyrene standards. The infrared spectra were recorded on a Bruker Vertex 70 FT-IR instrument, in transmission mode, in the 300–4000 cm−1 range (resolution 2 cm−1, 32 scans), at ambient temperature. 1H NMR spectra were recorded on 400 MHz Bruker spectrometer in CDCl3. The sorption capacity of water vapors (dynamic vapor sorption, DVS) was measured in dynamic regime at 25 °C by using an IGAsorp equipment (Hiden Analytical, Warrington, UK) at different relative humidity (RH). After drying with flowing nitrogen (250 mL/min) until the weight of the sample was in equilibrium at RH < 1%, experiments were carried out in the RH range 0–90%, with 10% humidity steps, each having a pre-established equilibrium time between 10 and 20 min (minimum time and time out, respectively).
The complex dielectric permittivity was measured using Novocontrol Dielectric Spectrometer Concept 40. Measurements were carried out at frequencies between 10−1 and 106 Hz at room temperature. For dielectric measurements, the liquid polymers were placed between two golden plated stainless steel electrodes (diameter 20 mm) which were pressed together. The distance between the electrodes was adjusted by glass spacers to 100 μm.
2.2. Synthesis of the polysiloxane precursors
A trimethylsilyl terminated poly(dimethyl methylhydro)siloxane (P1) was synthesized by cationic ring opening polymerization of D4 and D4H with a cation exchanger as catalyst and hexamethyldisiloxane as chain blocker (Scheme ), according to the procedure described in [Citation33]. The 1H NMR and GPC analyses indicated the composition (8.1% molar methylhydrogen groups) and the molecular mass, Mn = 8600 g/mol, PDI = 1.8.
In a similar reaction, using D4 and D4 V,[Citation34] a trimethylsilyl terminated poly(dimethyl-methylvinyl)siloxane with 8.1 mol% methylvinyl groups and Mn = 9200 g/mol, PDI = 1.5 was obtained (P2).
2.3. Synthesis of allyl benzaldehyde
In a three necked flask, fitted with condenser and dry nitrogen inlet and outlet, were charged 3.92 mL allyl chloride (3.68 g, 48.15 mmol), 5.88 g (48.15 mmol) 4-hydroxybenzaldehyde 98%, recrystallized once from water (m.p.=114–116 °C), 7.68 g (42.54 mmol) anhydrous potassium carbonate and 40 mL dimethylsulfoxide. The reaction mixture was magnetically stirred at 155–160 °C for 8 h. After cooling, the brown-dark oil was poured into water. After extraction twice with dichloromethane, the organic layer was stirred for 3 h with water. Finally, the product was extracted with diethyl ether and 6.24 g (80% yield) of clear brownish oil were obtained. The refraction index was nD20 = 1.572.
1H NMR (δ, ppm CDCl3): 9.91 (s, 1H, –CH=O), 7.870, 7.848 (d, 2H, ortho to CH=O, J 8.8 Hz), 7.056, 7.034 (d, 2H, ortho to –O–, J 8.8 Hz), 6.130–6.034 (m, 1H–CH=C), 5.484–5.347 (m, 2H, CH2=), 4.660, 4.647 (d, 2H –CH2–O–).
2.4. Synthesis of polar silicones
The chemical modifications of P1 and P2 are illustrated in Schemes and , with reaction details summarized in Table . The codes of the polar silicones contain the notation for the precursor and a code for the polar group.
Table 1. Experimental conditions for synthesis of polar silicones.
As an example of hydrosilylation reactions, the synthesis of P1EP is described. The copolymer P1 (1 g, 1.14 mmol SiH groups) and allylglycidyl ether (0.15 g, 1.31 mmol) were charged into a reaction flask under argon and dissolved in 5 mL dry toluene. Then the Pt(dvs) catalyst (30 μL) was added and the reaction mixture was stirred at 70 °C, for 7 h. The solvent and the double bond reagent excess were removed under vacuum.
As an example of thiol-ene addition, the preparation of P2COOH is described. In a reaction vessel, the siloxane copolymer P2 (1 g, 1.1 mmol vinyl groups) was dissolved in THF (5 mL), then 3-mercaptopropionic acid was added (0.144 g, 1.2 mmol) and after its dissolution, the catalyst DMPA (0.028 g, 0.11 mmol) was introduced and the vessel was closed. The UV irradiation was done with a laboratory lamp at 366 nm under magnetic stirring for 2 × 15 min intervals. As the viscosity of the solution increased after the reaction, THF was added to allow filtration of the solution. The solvent was removed and the crude product was washed several times with distilled water then dried.
P1CN, 1H-NMR (δ, ppm CDCl3): 4.723 (m, >(–)Si–H traces), 2.40 (m, 2H, NC–CH2–CH2–CH2), 1.76 (m, 2H, NC–CH2–CH2-CH2), 0.73 (m, 2H, NC–CH2–CH2–CH2-Si), 0.085 (m, >(–)Si–CH3).
P1EP, 1H-NMR (δ, ppm CDCl3): 4.726 (m, >(–)Si–H traces), 4.704–3.470 (m, 2H–CH2–CH2–CH2–O–, 2H–O–CH2–CH<), 3.176 (m, 1H–CH<), 2.824, 2.642 (m, 2H–CH2 (O) –CH < ring), 1.654 (m, 2H –CH2–CH2–CH2–), 0.552 (m, 2H >(–)Si–CH2–), 0.112 (m, >(–)Si–CH3).
P1DR1, 1H-NMR (δ, ppm CDCl3): 8.367 (m, 2H ortho to –NO2), 8.084 (m, 4H ortho to –N=N–), 7.100 (m, 2H ortho to –N<), 4.724 (m, >(–)Si–H traces), 3.983 (m, 2H >(–)Si–O–CH2–), 3.745 (m, 4H –CH2–N<), 1.354 (m, 3H–CH3), 0.135 (m, >(–)Si–CH3).
P1CHO, 1H-NMR (δ, ppm CDCl3): 9.932 (m, 1H –CH=O), 7.869 (m, 2H ortho to –CH=O), 7.106–7.008 (m, 2H ortho to –O–), 4.680 (m, >(–)Si–H traces), 4.037 (m, 2H –CH2–CH2–CH2–O–), 1.909 (m, 2H –CH2–CH2–CH2–), 0.684 (m, 2H >(–)Si–CH2–), 0.131 (m, >(–)Si–CH3).
P1Py, 1H -NMR (δ, ppm CDCl3): 8.176, 8.160 (d, 2H ortho to = N– J 6.4 Hz), 7.733 (m, 1H –OH), 6.845 (m, 1H –NH–), 6.516, 6.500 (d, 2H ortho to –NH– J 6.4 Hz), 4.232 (m, 2H –CH2–NH–), 3.941 (m, 2H –O–CH2–CH<), 3.385 (m, 2H –CH2–CH2–CH2–O–, 1H –CH<), 1.608 (m, 2H –CH2–CH2–CH2–O–), 0.502 (m, 2H >(–)Si–CH2–), 0.067 (m, >(–)Si–CH3).
P2Cl, 1H-NMR (δ, ppm CDCl3): 3.644 (m, 2H –CH2–Cl),2.633 (t, 2H Si–CH2–CH2–S–), 2.576 (t, 2H S–CH2– CH2–), 2.023 (q, 2H –CH2–CH2– CH2–), 0.871 (t, 2H Si–CH2–), 0.082 (m, >(–)Si–CH3).
P2COOH, 1H-NMR (δ, ppm CDCl3): 2.963 (m, 2H –CH2–COOH), 2.792 (m, 4H –CH2–S–CH2–), 0.879 (m, 2H Si–CH2–), 0.069 (m, >(–)Si–CH3).
3. Results and discussion
In order to allow comparison of various silicones containing polar groups while limiting the influence of molecular mass, chain ends, cross-linking conditions, or macromolecular microstructure, two trimethylsilyl end-capped polysiloxane precursors with similar molar mass and content of hetero-groups (hydrogen or vinyl) were synthesized by cation exchanger catalyzed ring opening co-polymerization of appropriate cyclosiloxanes, and were subsequently modified with several polar reagents (Schemes , Table ). The 1H NMR spectra of the two precursors (Figure S1) indicate the same content (8.1 mol %) of methylhydrogen groups (P1) and methylvinyl groups (P2), respectively. The molar mass (by GPC, SLS detector, polystyrene standards) showed very similar values of Mn (8600 g/mol for P1 vs. 9200 g/mol for P2), which indicate very similar average length of the macromolecular chains (118 structural units in P1 vs. 123 in P2).
Hydrosilylation or dehydrocoupling reactions were done on copolymer P1 in the presence of Pt catalyst (Scheme ), while thiol-ene addition on vinyl copolymer P2 was done using DMPA as photoinitiator under UV irradiation (Scheme ).
All the reactions were followed by 1H NMR and FT-IR spectroscopy and the reaction parameters were adjusted as to achieve as high as possible reaction conversion. However, the conversion of the Si–H groups in hydrosilylation of P1 was not complete. The case of allyl cyanide addition (as in P1CN) was largely discussed in our previous publications.[Citation35,36] A similar modification degree of the SiH groups was observed for the addition of allyl-benzaldehyde (P1CHO) and for dehydrocoupling with Disperse Red 1 (P1DR1). Apart from the inductive effects of the polar groups, steric hindrance might be a limiting factor, especially for P1DR1.[Citation37] At first sight, this is a drawback, since the un-reacted Si–H groups are easily hydrolysable and can act as cross-linking sites. From another perspective, this side reaction can be very nicely exploited to link such copolymers to high molecular mass HO-terminated PDMS, or to prepare cross-linked polar nanoparticles.[Citation29] Almost total conversion was achieved for the addition of allyl glycidyl ether (P1EP) and its subsequent modification with 4-aminopyridine (P1Py). As a consequence, in spite of incomplete conversion, the content of polar groups in the siloxane copolymers derived from P1 was of 7–8 mol%, thus allowing a satisfactory comparison between materials. In order to avoid premature cross-linking of the copolymers containing un-reacted Si–H groups, they were kept in solution and the solvent was removed just prior to analyses.
In Figures , the 1H NMR spectra of polar silicones P1EP, P1Py, P1CHO, and P1DR1 are given. In each case, the formation of the new copolymers was confirmed by the disappearance or diminishment of the Si–H proton signal at 4.7 ppm, as well as the disappearance of the double bond signal, which confirmed the total removal of the eventually non-reacted reagents in P1CN, P1CHO and P1EP. For P1DR1, besides the decrease of the 4.7 ppm signal, the presence of the aromatic protons in the correct ratio, as well as the shift of the CH2-O protons in DR1 confirmed the reaction (Figure ). The addition of 4-aminopyridine to P1EP was verified by the disappearance of the epoxy signal in 1H NMR spectrum at 2.7–3 ppm (Figure ).
The reactions were also followed by FT-IR spectroscopy, and confirmed by the disappearance or diminishment of the IR absorption band at 2154 cm−1, characteristic for Si–H bonds, together with the presence of absorption bands specific for the polar reagents in each case, as for example 2250 cm−1 (CN), 3050 and 910 cm−1 (epoxy), 1653 cm−1 (NH in P1Py), 1697 cm−1 (CH=O). The FT-IR spectrum of P1CHO is presented in Figure S2.
It is known that the Si–O–C bond is in principle hydrolyticaly unstable, although its sensitivity is rather low for alkoxy groups with a large number of carbon atoms.[Citation7] In P1DR1, we followed the stability of the Si–O–C groups toward hydrolysis by FT-IR. The Si–O–C bond has a few characteristic absorption bands in IR (roughy in the range 1110–1000 cm−1 – asymmetric stretch), but unfortunately they are overlapped by the strong Si-O-Si bands. According to [Citation38], the bands at 1140 and 1184 cm−1 could be more useful for comparison, being assigned to longer chain alkoxy groups (Figure S3). In the hypothesis of a significant degree of hydrolysis, these bands should diminish or disappear, while the result of such reaction would be inter-molecular Si–O–Si links, characterized by widening of the polysiloxane region (‘T’ type siloxane bonds in cross-linked structures, with characteristic bands at 1110–1000 cm−1). In Figure S3, the FT-IR spectra of P1DR1 just after synthesis and after several months of storing in normal conditions were superposed. The spectra are practically identical, except the bands indicating hydrolysis of the un-modified Si–H groups (decrease of the Si–H bands at 2158 and 910 cm−1, modification and increase of the Si-OH band at around 3440 cm−1). As the Si–O–C bands did not diminish, we suppose that the hydrophobic behavior provided by the dimethylsiloxane units, as well as the bulkiness of the DR1 fragments may ensure a relatively good stability against hydrolysis of the Si–O–C bonds.
In the second series, the dimethyl-methylvinyl copolymer P2 was modified by thiol-ene addition with 3-chloro-1-propanethiol (P2Cl) or 3-mercaptopropionic acid (P2COOH). In the 1H NMR spectra, the disappearance of the vinyl protons signals at 5.5–6 ppm showed complete addition of the thiols to the vinyl groups (Figures and ), confirmed by the presence of >CH2 protons in α position to Si, while the absence of the thiol proton at 1.37 ppm in 3-chloro-1-propanethiol and at 1.67 ppm in 3-mercaptopropionic acid indicates the removal of excess reagents.
The dielectric properties of the prepared siloxane copolymers were measured in liquid state, without cross-linking (Figure ). Table summarizes the dielectric permittivity (ε′), dielectric loss (ε″), and conductivity (σ) at 10 kHz for all copolymers. At this frequency all the samples, except P1Py, exhibit a plateau of the ε′ trend. Compared to a neat PDMS, having ε′ values below 3, the permittivity values increased, as expected, when polar groups were chemically attached. Although a relatively low content of polar groups was used (ca. 8%), permittivity values of 5.4 and even over 7.4 were achieved (Table ). For the ‘more polar’ copolymers having ε′ > 5, the ε″ values (at 10 kHz) were significantly higher than for the other samples and the same trend was observed for the conductivity (Table ). At low frequencies ε′ increased, which is a common phenomenon known as the low frequency dispersion.[Citation39] The dielectric loss also increased at low frequencies as a result of enhanced mobility of charge carriers. A strong increase in the dielectric loss for copolymers with high content of polar (cyano-) groups has been previously observed,[Citation35] as well as an increase in conductivity. For the entire series of polar silicones, the conductivity values generally remained in the range of dielectric materials. Like in the case of cyano-modified silicones with very high substitution degree,[Citation35] the high dielectric losses at low frequency, with values of ε″ up to 105, correlated with their frequency dependence (linear at low frequencies in a double logarithmic scale, with the slope of log ε″(f) close to −1) point toward a mainly conductive nature of the dielectric loss.
Figure 6. Dielectric properties of a series of siloxane copolymers having around 8% different polar groups.
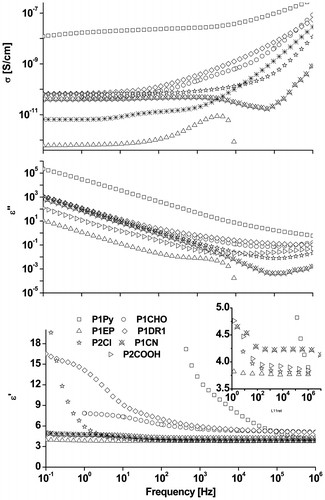
Table 2. Dielectric permittivity, loss, and conductivity (at 10 kHz) of polysiloxanes with different dipoles attached in similar proportion (ca. 8%).
It is known that the ability of a material to be polarized in an electric field depends on the polarity and content of the substituents. Given the similar content of polar groups, a linear increase of ε′ with the polarity of the substituents might be anticipated. In order to have a quantitative estimation of the effect of various polar groups, the dipole moment, molar volume, and molar polarizability of different molecules used to modify the polysiloxanes were calculated with Hyperchem [Citation40] and ACD software, respectively. To simplify the calculation and to allow comparison, we used theoretical molecules having a –CH3 group instead of the polysiloxane chain. Although it cannot provide the real parameters for the siloxane copolymers, this procedure ensures suitable models within similar approximation. The obtained values are summarized in Table .
Table 3. Dipole moments, molar polarizability, and molar volume of model molecules containing the polar substituents attached to the silicon atom and a methyl group instead of the polysiloxane chain.
When judging the polarity only in terms of dipole moment values, a direct correlation with the measured dielectric permittivity was not found. For example, although copolymers P1EP and P2Cl have different dipole moments, similar ε′ value at 10 kHz were found. Copolymer P1Py, showed an unexpectedly high ε′ of 7.4, although its dipole moment is lower than that of DR1 derivative. In this case, the very high permittivity was accompanied by very high loss and conductivity. The peculiar behavior of sample P1Py (verified with different batches) might limit the accuracy of data interpretation.
In the attempt to correlate the structural parameters with the measured ε′ values, we took into account either the dipole moment, the molar volume, the molar polarizability of the dipoles as calculated for the molecules in Table , or their product (parameter K1 in Figure (a)). In neither of these cases a direct (linear) correlation with the measured dielectric permittivity (at 10 kHz) was obtained. The ε′ versus K1 data is best described with a second-order polynomial trend line, with R2 = 0.85 (Figure (a)). Since sample P1Py has variable permittivity at high frequencies, the same plots with ε′ values at 100 kHz were made, as well as without this sample. The result did not change significantly, since polynomial trend lines with correlation factor R2 around 0.84 were found to best describe the data (Figure S4). Thus, it seems that this sample is not anomalous as we supposed at first sight, which leads to the hypothesis that other factors might have important influence on permittivity values.
Figure 7. Dielectric permittivity of polar silicones versus a calculated parameter [K1 = (dipol moment) × (molar volume) × (molar polarizability)] of different polar substituents (a) and versus a semi-empirical parameter K2 (b) or K2′ (c), which takes into account the measured moisture sorption capacity, expressed as maximum percents in K2 and as percents for RH = 50% in K2′; K2 = K1 × (% water adsorbed).
![Figure 7. Dielectric permittivity of polar silicones versus a calculated parameter [K1 = (dipol moment) × (molar volume) × (molar polarizability)] of different polar substituents (a) and versus a semi-empirical parameter K2 (b) or K2′ (c), which takes into account the measured moisture sorption capacity, expressed as maximum percents in K2 and as percents for RH = 50% in K2′; K2 = K1 × (% water adsorbed).](/cms/asset/28863711-f34d-459e-9c01-fdc6e1792b12/tdmp_a_1169381_f0007_oc.gif)
As it is known, traces of water may induce increased ε′ values due to ionic conductivity.[Citation8,41] The presence and amount of moisture into different samples depend on their chemical structure. In principle, water was not used in the purification process and no special drying was applied before dielectric measurements, thus the atmospheric moisture would be responsible for the eventual artificial increase of ε′. Dynamic vapor sorption measurements (DVS) were done using a fully automated gravimetric analyzer with an ultrasensitive microbalance, working in dynamic regime. The water vapors sorption isotherms for all the samples are shown in Figure . Similar behavior was found for most of the samples, with maximum water sorption capacity of less than 1%, while samples P2COOH and especially P1Py adsorbed more water vapors. The low water uptake in the case of P1DR1 could explain the long-term stability of the Si–O–C bonds, as explained before, although these bonds are in theory susceptible to hydrolytic cleavage.[Citation7]
The increased hydrophilicity of copolymer P1Py observed in DVS measurements might be responsible for its dielectric behavior. At this point, it seemed logical to try to correlate the water vapor sorption capacity (expressed as maximum percent of adsorbed moisture reported to the dry sample) with the dielectric behavior. Thus, a new parameter was calculated, K2 = K1 × (max % water adsorbed) and it was plotted versus ε′ at 10 kHz. In this case, a linear trend was obtained (Figure (b)), with very good correlation factor, despite all the approximations that were used. If we go further and consider the water content of the samples in ambient (normal) conditions as being similar to the DVS values at 50% relative humidity, and take this parameter to calculate K2′ the same trend is obtained (Figure (c)), but the data is best described with a polynomial trend line.
Although more investigations are necessary to confirm these findings, at this moment we suppose that a cumulative effect of several structural factors in tailoring the dielectric permittivity of polysiloxanes modified with different polar groups might be estimated in a semi-empirical way, taking into account calculated parameters and the water sorption capacity. It was once again demonstrated that the amount of humidity that a sample is capable to store has an important consequence on its dielectric behavior. On the other hand, our experiments indicate that, although dipole moment is the first parameter taken into account when polar groups are chosen, it is not the only one that actually governs the dielectric properties.
4. Conclusions
A series of polar silicones was synthesized by hydrosilylation on a poly(dimethyl methylhydro)siloxane or by thiol-ene addition on a poly(dimethyl-methylvinyl)siloxane with similar composition and molar mass. Different substituents with high dipole moment (epoxy, pyridyl, aldehyde, cyano-, nitroazobenzene, chloro, or carboxyl) were thus attached. Their dielectric properties were measured in un-cross-linked state, as well as their dynamic water vapors sorption behavior. Although a relatively low content of polar groups was used (ca. 8%), permittivity values of 5.4 and even 7.4 (at 10 kHz) were achieved, either due to the large dipole moment of the substituent or to the presence of important amounts of moisture. A combined effect of the calculated dipole moment, molar polarizability, molar volume, and the measured maximum water sorption capacity on dielectric permittivity was observed. It was once again demonstrated that the amount of humidity that a sample is capable to store has an important consequence on its dielectric behavior. Thus, a wider approach is needed in choosing the polar molecules attached to polysiloxanes, taking into account the cumulative effect of polarity and hydrophilicity.
Supplemental data
Supplemental data for this article can be accessed http://dx.doi.10.1080/15685551.2016.1169381.
Disclosure statement
No potential conflict of interest was reported by the authors.
Funding
This research was supported by the Swiss National Science Foundation and UEFISCDI, under the Swiss-Romanian Cooperation Program [grant number IZERZO_142215/1 (10/RO-CH/RSRP/01.01.2013)].
TDMP_A_1169381_Supplemental_Information.pdf
Download PDF (466.7 KB)References
- Kornbluh R, Pelrine R. High-performance acrylic and silicone elastomers. In: Carpi F, De Rossi D, Kornbluh R, Pelrine R, Sommer-Larsen P, editors. Dielectric elestomers as electromechanical transducers: fundamentals, materials, devices, models and applications of an emerging electroactive polymer technology. Pisa: Elsevier; 2008. p. 33–42.
- Romasanta LJ, Lopez-Manchado MA, Verdejo R. Increasing the performance of dielectric elastomer actuators: a review from the materials perspective. Prog. Polym. Sci. 2015;51:188–211.10.1016/j.progpolymsci.2015.08.002
- Biggs J, Danielmeier K, Hitzbleck J, et al. Electroactive polymers: developments of and perspectives for dielectric elastomers. Angew. Chem. Int. Ed. 2013;52:9409–9421.10.1002/anie.v52.36
- Vertechy R, Fontana M, Stiubianu G, et al. Open-access dielectric elastomer material database, In: Bar-Cohen Y, editor. Proc. SPIE 9056, Electroactive Polymer Actuators and Devices (EAPAD), 2014 Mar 8, San Diego, CA. 90561R, doi:10.1117/12.2045053.
- Xu D, Tairych A, Anderson IA. Stretch not flex: programmable rubber keyboard. Smart Mater. Struct. 2016;25: p. 7. 015012.
- Kuo ACM. Poly(dimethylsiloxane): polymer data handbook. New York: Oxford University Press; 1999. p. 411–435.
- Noll W. Chemistry and technology of silicones. New York (NY): Academic Press; 1968.
- Madsen FB, Daugaard AE, Hvilsted S, et al. The current state of silicone-based dielectric elastomer transducers. Macromol. Rapid Commun. 2016;37(5):378–413. doi:10.1002/marc.201500576
- (a) Tugui C, Cazacu M, Sacarescu L, et al. Full silicone interpenetrating bi-networks with different organic groups attached to the silicon atoms. Polymer. 2015;77:312–322; (b) Tugui C, Stiubianu G, Iacob M, et al. Bimodal silicone interpenetrating networks sequentially built as electroactive dielectric elastomers. J. Mater. Chem. C. 2015;3:8963–896910.1016/j.polymer.2015.09.042
- Bele A, Cazacu M, Racles C, et al. Tuning the electromechanical properties of silicones by crosslinking agent. Adv. Eng. Mater. 2015;17:1302–1312.10.1002/adem.v17.9
- Kussmaul B, Risse S, Wegener M, et al. Matrix stiffness dependent electro-mechanical response of dipole grafted silicones. Smart Mater. Struct. 2012;21: p.6. 064005.10.1088/0964-1726/21/6/064005
- Madsen FB, Dimitrov I, Daugaard A, et al. Novel cross-linkers for PDMS networks for controlled and well distributed grafting of functionalities by click chemistry. Polym. Chem. 2013;4:1700–1707.10.1039/c2py20966g
- Risse S, Kussmaul B, Krüger H, et al. DEA material enhancement with dipole grafted PDMS networks. In: Bar-Cohen Y, Carpi F, editors. Electroactive Polymer Actuators and Devices (EAPAD), Proc. of SPIE Vol. 7976; 2011 San Diego, CA. doi:10.1117/12.881919.
- Carpi F, Gallone G, Galantini F, et al. Enhancing the dielectric permittivity of elastomers. In: Carpi F, De Rossi D, Kornbluh R, Pelrine R, Sommer-Larsen P, editors. Dielectric elastomers as electromechanical transducers: fundamentals, materials, devices, models and applications of an emerging electroactive polymer technology. Pisa: Elsevier; 2008, p. 51–68.
- Wang Z, Nelson JK, Miao J, et al. Effect of high aspect ratio filler on dielectric properties of polymer composites: a study on barium titanate fibers and graphene platelets. IEEE Trans. Dielectr. Electr. Insul. 2012;19:960–967.10.1109/TDEI.2012.6215100
- Yin Y, Chang X. Ocean engineering application of nanocomposites. In: Leng J, Lau AKT, editors. Multifunctional polymer nanocomposites. Boca Raton (FL): CRC Press / Taylor and Francis Group; 2011. p. 423–438.
- Sebastian MT, Jantunen H. Polymer–ceramic composites of 0–3 connectivity for circuits in electronics: a review. Int. J. Appl. Ceram. Technol. 2010;7:415–434.
- Huang C, Zhang QM. Fully functionalized high-dielectric-constant nanophase polymers with high electromechanical response. Adv. Mater. 2005;17:1153–1158.10.1002/(ISSN)1521-4095
- Quinsaat JE, Alexandru M, Nueesch F, et al. Highly stretchable dielectric elastomer composites containing high volume fractions of silver nanoparticles. J. Mater. Chem. A. 2015;3:14675–14685.10.1039/C5TA03122B
- Bele A, Stiubianu G, Varganici C, et al. Silicone dielectric elastomers based on radical crosslinked high molar mass polydimethylsiloxane co-filled with silica and barium titanate. J. Mater. Sci. 2015;50:6822–6832.10.1007/s10853-015-9239-y
- Bele A, Cazacu M, Stiubianu G, et al. Silicone-barium titanate composites with increased electromechanical sensitivity. The effects of the filler morphology. RSC Adv. 2014;4:58522–58529.10.1039/C4RA09903F
- Stiubianu G, Bele A, Cazacu M, et al. Dielectric silicone elastomers with mixed ceramic nanoparticles. Mater. Res. Bull. 2015;71:67–74.10.1016/j.materresbull.2015.07.005
- Alexandru M, Cazacu M, Doroftei F, et al. On the morphology and potential application of polydimethylsiloxane-silica-titania composites. eXPRESS Polym. Lett. 2011;5:188–196.10.3144/expresspolymlett.2011.17
- Racles C, Cazacu M, Fischer B, et al. Synthesis and characterization of silicones containing cyanopropyl groups and their use in dielectric elastomer actuators. Smart Mater. Struct. 2013;22:104004 ( 10pp).10.1088/0964-1726/22/10/104004
- Yu L, Madsen FB, Hvilsted S, et al. Dielectric elastomers, with very high dielectric permittivity, based on silicone and ionic interpenetrating networks. RSC Adv. 2015;5:49739–49747.10.1039/C5RA07375H
- Madsen FB, Yu L, Daugaard AE, et al. A new soft dielectric silicone elastomer matrix with high mechanical integrity and low losses. RSC Adv. 2015;5:10254–10259.10.1039/C4RA13511C
- Kussmaul B, Risse S, Kofod G, et al. Enhancement of dielectric permittivity and electromechanical response in silicone elastomers: molecular grafting of organic dipoles to the macromolecular network. Adv. Funct. Mater. 2011;21:4589–4594.10.1002/adfm.v21.23
- Dünki SJ, Tress M, Kremer F, et al. Fine-tuning of the dielectric properties of polysiloxanes by chemical modification. RSC Adv. 2015;5:50054–50062.10.1039/C5RA07412F
- Racles C, Bele A, Dascalu M, et al. Polar-nonpolar interconnected elastic networks with increased permittivity and high breakdown fields for dielectric elastomer transducers. RSC Adv. 2015;5:58428–58438.10.1039/C5RA06865G
- Yilgor I, McGrath JE. Polysiloxane containing copolymers: a survey of recent developments. Adv. Polym. Sci. 1988;86:1–87.10.1007/BFb0025273
- Mark JE, Schaefer DW, Lin G. The Polysiloxanes. New York (NY): Oxford University Press; 2015.
- Cazacu M, Racles C. Recent developments in siloxane-based polymers and copolymers. In: Dragan S, editor. New trends in nonionic (co)polymers and hybrids. Nova Science; 2006; 168–213.
- Racles C, Hamaide T. Synthesis and characterization of water soluble saccharide functionalized polysiloxanes and their use as polymer surfactants for the stabilization of polycaprolactone nanoparticles. Macromol. Chem. Phys. 2005;206:1757–1768.10.1002/(ISSN)1521-3935
- (a) Cazacu M, Marcu M, Holerca M, et al. Heterogeneous catalysed copolymerization of octamethylcyclotetrasiloxane with 1,3,5,7-tetravinyl-1,3,5,7-tetramethylcyclotetrasiloxane. J. Macromol. Sci.-Pure and Appl. Chem. 1996;33: 65–76. (b) Cazacu M, Marcu M, Petrovan S, et al. Cationic heterogeneous copolymerization of octamethylcyclotetrasiloxane with 1,3,5,7-tetramethyl-1,3,5,7-tetravinylcyclotetrasiloxane. Optimization of reaction conditions. Polym. Plast. Technol. Eng. J. 1996;35:327–347.10.1080/10601329608010854
- Racles C, Alexandru M, Bele A, et al. Chemical modification of polysiloxanes with polar pendant groups by co-hydrosilylation. RSC Adv. 2014;4:37620–37628.10.1039/C4RA06955B
- Racles C, Alexandru M, Musteata V, et al. Tailoring the dielectric properties of silicones by chemical modification. In: Pandalai SG, editor. Recent research developments in polymer science. Trivandrum: Transworld Research Network; 2014. 2, p.7–36. ISBN: 978-81-7895-611-4.
- Racles C. Polysiloxanes with azo-aromatic mesogenic groups. Rev. Roum. Chim. 2009;4:589–595.
- Smith AL, editor. Analysis of silicones. Vol. 41 in Chemical Analysis, A series of monographs on analytical chemistry and its applications. New York: Wiley; 1974.
- Jonscher AK. Dielectric relaxation in solids. J. Phys. D: Appl. Phys. 1999;32:R57–R70.10.1088/0022-3727/32/14/201
- Hyper Chem (TM) Professional 7.51, Hypercube, Inc., Florida, USA.
- Grace LR. The effect of moisture contamination on the relative permittivity of polymeric composite radar-protecting structures at X-band. Compos. Struct. 2015;128:305–312.10.1016/j.compstruct.2015.03.070