ABSTRACT
Introduction: Ever since their discovery, liposomes have been radiolabeled to monitor their fate in vivo. Despite extensive preclinical studies, only a limited number of radiolabeled liposomal formulations have been examined in patients. Since they can play a crucial role in patient management, it is of importance to enable translation of radiolabeled liposomes into the clinic.
Areas covered: Liposomes have demonstrated substantial advantages as drug delivery systems and can be efficiently radiolabeled. Potentially, radiolabeled drug-loaded liposomes form an elegant theranostic system, which can be tracked in vivo using single-photon emission computed tomography (SPECT) or positron emission tomography (PET) imaging. In this review, we discuss important aspects of liposomal research with a focus on the use of radiolabeled liposomes and their potential role in drug delivery and monitoring therapeutic effects.
Expert opinion: Radiolabeled drug-loaded liposomes have been poorly investigated in patients and no radiolabeled liposomes have been approved for use in clinical practice. Evaluation of the risks, pharmacokinetics, pharmacodynamics and toxicity is necessary to meet pharmaceutical and commercial requirements. It remains to be demonstrated whether the results found in animal studies translate to humans before radiolabeled liposomes can be implemented into clinical practice.
1. Introduction
Ever since their discovery by Bangham et al., liposomes have been studied extensively for their diagnostic and therapeutic potential [Citation1,Citation2]. Liposomes consist of negatively charged, positively charged, non-charged and/or zwitterionic phospholipids. These phospholipids are amphiphilic molecules that form the main component of biological membranes. After dispersion in aqueous media they spontaneously form multilamellar vesicles of varying diameters, which can be sized via extrusion or ultrasonication. The small lipid vesicles enclose an aqueous core, which can be used to carry hydrophilic drugs, while lipophilic drugs are carried in the lipid bilayer. Amphiphilic drugs consist of a lipophilic element and a hydrophilic element. The lipophilic part of these drugs resides in the lipid bilayer, while the hydrophilic part is located in the aqueous core or the exterior of the liposomes [Citation3]. Liposomes can potentially be used for delivery of their therapeutic cargo to diseased tissues. The targeting of these lipid vesicles is based on the increased vascular permeability found in tumors and infected/inflamed tissue, also known as the enhanced permeability and retention (EPR) effect [Citation4]. In addition, targeting properties are dependent on factors that affect in vivo behavior, such as size, composition, charge, and surface modification [Citation5–Citation7]. The size of the liposomes ranges from 50 nm to several micrometers, but the most stable liposome range in size from 90 to 250 nm [Citation8]. When smaller than 70 nm, liposomes are primarily taken up by the liver, while an increase of splenic uptake is observed when liposomes are used, which are larger than 200 nm. In both cases, circulation time of the liposomes is relatively short and biodistribution of the liposomes is altered. Liposomes with a diameter of 100–200 nm show up to fourfold higher tumor uptake rate compared to larger or smaller vesicles, thus emphasizing the importance of sizing liposomes during preparation [Citation9]. Apart from size, composition of the liposomes is important to obtain acceptable stability in vivo. For example, the addition of ample amounts of cholesterol to the phospholipids may result in increased rigidity and chemical stability of liposomes in physiological conditions. Several liposomal formulations are U.S. Food and Drug Administration (FDA)-approved drug carriers carrying anticancer drugs, such as vincristine (Marqibo®) or cytarabine (Depocyt®) [)] [10–13]. When administered intravenously, conventional liposomes will be coated with serum proteins. These serum proteins can act as opsonins, which results in recognition of the liposomes by phagocytic cells of the mononuclear phagocyte system and subsequent rapid clearance of the liposomes [Citation14–Citation16]. To increase their circulation time, the liposomal surface can be coated with polyethylene glycol (PEG) [)] [Citation17–Citation20]. The first FDA-approved PEGylated liposomal formulations were liposomes loaded with doxorubicin (Doxil®/Caelyx®/Myocet®) in 1995 [Citation21]. To obtain specific targeting, the liposomal surface can be modified with antibodies, peptides or proteins [] [Citation22–Citation25]. Ahmad et al. demonstrated that immunoliposomes carrying monoclonal antibodies or their fragments (Fab′) at the distal ends of their PEG chain showed specific targeting to solid tumors. In addition, they showed the enhanced therapeutic effects of the targeted liposomes containing doxorubicin, compared to non-targeted liposomes [Citation26–Citation28].
Figure 1. Schematic overview of four types of modifications of liposomal formulations. Conventional liposomes are composed of cationic, anionic, or neutral phospholipids and cholesterol, and enclose and aqueous core (a). These liposomes can be loaded with hydrophobic as well as hydrophilic agents. PEGylated liposomes to prolong the conventional liposomes (b). Targeted liposomes to which proteins, peptides, antibodies, carbohydrates or small molecules can be attached to enable specific targeting of the liposomes and their content (c). Theranostic liposomes consist of a liposome, an imaging agent, a therapeutic component and a targeting ligand (d) (Reproduced with permission from Sercombe et al. [Citation29]).
![Figure 1. Schematic overview of four types of modifications of liposomal formulations. Conventional liposomes are composed of cationic, anionic, or neutral phospholipids and cholesterol, and enclose and aqueous core (a). These liposomes can be loaded with hydrophobic as well as hydrophilic agents. PEGylated liposomes to prolong the conventional liposomes (b). Targeted liposomes to which proteins, peptides, antibodies, carbohydrates or small molecules can be attached to enable specific targeting of the liposomes and their content (c). Theranostic liposomes consist of a liposome, an imaging agent, a therapeutic component and a targeting ligand (d) (Reproduced with permission from Sercombe et al. [Citation29]).](/cms/asset/c97d0cd2-1711-4fd9-a6b9-c0c82b5c7cee/iedd_a_1205584_f0001_oc.jpg)
Besides being a drug carrier, liposomes can also be labeled with radionuclides to allow for in vivo tracking using non-invasive radionuclide imaging techniques, which enables an theranostic approach []. Several methods have been developed to produce efficiently labeled and radiochemically stable liposomal formulations. The combination of diagnostic and therapeutic capabilities in a single agent can be used to develop specific and personalized therapies. This so-called theranostic approach has several advantages. The in vivo biodistribution behavior of radiolabeled liposomes can be monitored by radionuclide imaging. Radiolabeled liposomes could function as a companion diagnostic, which is administered prior to therapy to predict accumulation at target sites. This would be beneficial to predict the potential therapeutic response and to determine the dose to obtain an optimal therapeutic response, while lowering the side effects. If accumulation of the radiolabeled liposomes is considered sufficient, the therapeutic liposomes can be administered. In addition, drug-loaded liposomes can also be radiolabeled to verify the delivery of their encapsulated drugs to the tissue of interest and for diagnosis of disease. Insufficient accumulation of the liposomes could explain a lack of therapeutic response or imaging could visualize possible increased immune responses [Citation30]. Liposomes can be adapted when inadequate targeting is observed or the treatment strategy can be altered. So, imaging results could have an impact on patient management by protecting patients from unnecessary side effects and costs if the treatment does not reach target sites. Thus, radionuclide imaging of liposomes and liposomal drugs can play an important role in the management of the individual patient.
2. Radionuclide imaging
Positron emission tomography (PET) and single photon emission computed tomography (SPECT) image acquisition can be used to determine the in vivo behavior of radiolabeled compounds. With these imaging techniques the in vivo distribution of the radiolabeled liposomes can be monitored quantitatively during days or even weeks, depending on the half-life of the radionuclide used. SPECT imaging is based on the measurement of single photons emitted by γ-emitting radionuclides, such as 99mTc (t½ = 6 h), 111In (t½ = 2.8 days), or 123I (t½ = 13.2 h). These photons are registered by detectors that acquire multiple two-dimensional (2D) projections. These 2D images, obtained from different angles, can be reconstructed into 3D images [)].
Figure 2. (a) SPECT image acquisition. The radionuclide emits γ-rays. The photons are collimated by the lead collimator and reach the sodium-iodine (NaI) chrystal, which produce scintillation. The scintillation is converted into an electronic signal, which is stored digitally to be processed to form the actual image. (b) Coincidence principle of PET. A radionuclide emits a positron, which annihilates with an electron. This results in two γ-photons of 511 keV that are emitted in opposite direction. These photons are detected in the ring of detectors and the activity distribution can be reconstructed into an image.
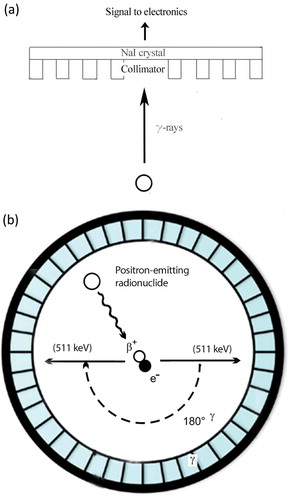
PET imaging is based on the emission of positrons by radioisotopes, such as 18F (t½ = 110 min), 68Ga (t½ = 68 min), or 64Cu (t½ = 12.7 h). These positrons annihilate with an electron that results in a pair of γ-photons, each of 511 keV, directed in opposite direction. The scintillator converts these photons into visible light, which is detected by a photomultiplier and these signals can be reconstructed into 3D images [)] [Citation31].
In a clinical setting, PET imaging outperforms SPECT imaging in terms of sensitivity and spatial resolution (PET = 2–4 mm vs. SPECT = 4–6 mm). In contrast, preclinical microSPECT imaging systems have a higher spatial resolution than microPET systems (microSPECT = 0.3–0.5 mm vs. microPET = 1.5–2.5 mm) [Citation32]. Using PET images, uptake of the radionuclides in tissues of interest can be determined quantitatively, which remains a challenge for (clinical) SPECT images due to the low resolution of these images. Both SPECT and PET can be combined with other modalities, such as computed tomography (CT) or magnetic resonance imaging to obtain additional anatomical information [Citation33]. Here, we focus on imaging of radiolabeled liposomes using SPECT and PET.
3. Radiolabeling of liposomes
The choice of radionuclide for radiolabeling of liposomes is dependent on the aim of the application. To study the in vivo behavior of the liposomes, 14C-labeled lipids and 3H-labeled cholesterol have been used. Since carbon and hydrogen are present in the original molecules of the lipids and cholesterol, the in vivo behavior will not change upon isotope substitution. However, 14C and 3H are pure ß-emitters with long half-lives (5730 and 12.33 years, respectively), which makes it unfeasible to use these isotopes noninvasively for imaging in live animals or human subjects [Citation34,Citation35]. To allow for non-invasive in vivo imaging with PET and SPECT, positron and γ-emitters are used. Alternatively, high-energy ß- or α-emitters can be used for therapeutic purposes. Radionuclides can be entrapped in the aqueous core of the liposomes, entrapped in the lipid bilayer or attached to the surface of the liposomes. The radiolabeled formulation should have a high-radiochemical stability in an in vitro and in vivo environment to prevent injection or release of the free radiolabel. Release of the radiolabel in vivo alters the biodistribution of the radiolabel and would no longer reflect the fate of the liposomes in vivo. In addition, the physical half-life of the radiolabel should be compatible with the circulatory half-life of the liposomes. To obtain radiolabeled liposomal formulations, several methods have been developed. Three main approaches for the radiolabeling of liposomes can be distinguished (). Liposomes can be radiolabeled during preparation by (1) passive encapsulation of the radionuclide or by (2) labeling of the liposomal membranes. After preparation of the liposomes, the preformed liposomes can be radiolabeled by (3) loading of the radionuclide into liposomes either via an ionophore, using a lipophilic chelator, or by labeling the liposomal surface after inclusion of a chelator on the surface of the liposomes [Citation36].
Figure 3. Schematic overview of the three radiolabelling approaches. Passive encapsulation of the radionuclides during preparation using a chelator (DOTA), liposomal membrane labelling during preparation of the liposomes, remote loading of radionuclides into preformed liposomes via ionophores or using lipophilic chelators (2-hydroxyquinoline), and surface labelling after incorporation of a chelator (DOTA) to the lipid bilayer or PEG are shown. In this figure, radionuclides are represented by the open circles.
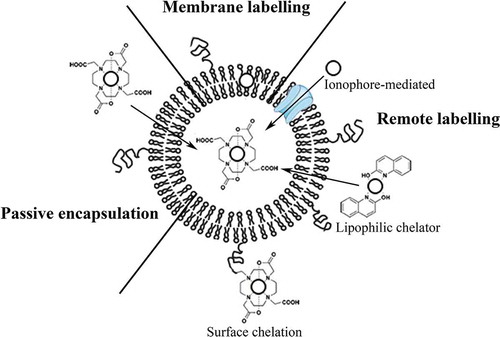
4. Encapsulation of radionuclides into liposomes
The first method that was used to radiolabel liposomes is often referred to as ‘passive encapsulation’. Using this method, the radionuclide is encapsulated into the internal compartment of liposomes during preparation of the lipid vesicles [Citation37]. To achieve encapsulation, the radionuclide (either free or chelated) is added to the aqueous solution in which the liposomes are formed. During formation of the lipid vesicles, the radionuclide is trapped in the aqueous core of the liposomes. This method has been used to entrap 2-deoxy-2-[18F]fluoro-d-glucose ([18F]FDG), 3-[18F]fluoro-1,2-dipalmitoylglycerol [18F] fluorodipalmitin ([18F]FDP) and 99mTc into liposomes (). However, the loading efficiency is low, often less than 10% which is far from optimal for the use in (pre)clinical studies [Citation38–Citation41].
Table 1. Overview of radiolabeling methods.
4.1. Radiolabeling of the liposomal membrane
The second approach involves labeling of the liposomal membrane. Radionuclides can be coupled to components of the liposomal membrane prior to the formation of liposomes, or a radionuclide containing hydrophobic molecule can be added to the formulation which would then be incorporated in the lipid bilayer. Although higher labeling efficiency can be obtained with this method, stability of the radiolabeling is a risk. Upon i.v. injection, the radiolabel could be transchelated to serum proteins in the blood, such as transferrin, particularly if chelating agent is neither incorporated in the liposomal bilayer nor in the aqueous core. This can result in loss of radioactivity from the liposomes, which can affect image quality negatively and may lead to an erroneous impression of the liposome distribution. This makes this method less attractive for the in vivo evaluation of the biodistribution profile.
4.2. Radiolabeling of preformed liposomes
For application in the preclinical or clinical setting, it is important to use a fast (0.5–2 h) method to produce a radiolabeled product. In addition, high-incorporation efficiency (preferably >50%) and good retention of the radiolabel are mandatory. Most efficient labeling and the best radiolabel retention of liposomal formulations can be obtained with remote loading methods. These methods attach the radiolabel to preformed liposomes. Various remote labeling methods have been developed, which use transmembrane pH, osmotic or a concentration gradient. Recently, it has been demonstrated that 64Cu can cross the liposomal membrane of preformed liposomes unassisted, when these liposomes contain a high-affinity copper chelator, such as 1,4,7,10-tertraazacyclotetradecane–1,4,7,10-tetraacetic acid (DOTA). The chelator binds free copper in the aqueous core, resulting in copper depletion and establishes a steep transmembrane copper gradient. Upon incubation with 64Cu, 64Cu diffuses spontaneously across the bilayer to be trapped in the liposomal core. The high-loading efficiency (>95%) and high-radionuclide retention after incubation in mouse serum (>95% after 18 h at 37°C), demonstrate that this easy remote labeling method has great potential for future use in (pre)clinical settings [Citation43]. Nevertheless, most remote labeling methods require the use of ionophores or lipophilic chelators to assist the radionuclide to accumulate in the liposomes.
4.2.1. Radiolabeling of preformed liposomes using ionophores
The use of hydrophilic ion transport molecules can be used to actively transport the radiolabel into the aqueous core of preformed liposomes, where the radionuclide can subsequently be trapped by encapsulated chelators. Ionophores form a hydrophilic channel through the lipid bilayer, which allows radionuclides to pass through the membrane without interaction with the lipophilic bilayer. For example, the ionophore A23187 can be incorporated in the lipid bilayer to label liposomes with 111In3+ at 60–80°C. After crossing the bilayer, the 111In3+ is chelated by the encapsulated chelator nitrilotriacetic acid (NTA). A high, up to 90%, labeling efficiency can be achieved, however radiolabel retention was not checked [Citation44]. Unfortunately, the incorporation of an ionophore in the lipid bilayer and the need to radiolabel at high temperatures might alter the behavior of the liposomes in vivo.
4.2.2. Radiolabeling of preformed liposomes using lipophilic chelators
Lipophilic chelators, such as 8-hydroxyquinoline (oxine), can be used for the remote loading of radionuclides, such as 67Ga, 68Ga, 111In or, 99mTc to obtain radiolabeled liposomes. Radionuclides can form complexes with lipophilic chelators, and these complexes can diffuse through the lipid bilayer. For example, liposomes can be incubated with 111In–oxine. The hydrophilic oxine is incorporated into the lipid bilayer of the liposomes. After crossing the liposomal membrane, the chelated radionuclide can be transchelated to a pre-incorporated hydrophilic chelator, such as diethylenetriaminepentaacetic acid (DTPA) [Citation48–Citation50]. Pre-incorporation of a chelator in the core of the liposomes increases the radiolabeling efficiency and radiochemical stability significantly [Citation49]. The advantage of transchelation to encapsulated chelators, such as desferal or DTPA, is that after release from the liposomes, the radiolabeled complex is rapidly cleared via the kidneys, while non-chelated radionuclides can bind to serum proteins, resulting in accumulation in tissues, such as liver, spleen, lymph nodes, and bone marrow. The most widely known labeling procedure to label liposomes makes use of the lipophilic chelator hexamethylpropyleneamine oxime (HMPAO). 99mTc forms a lipophilic complex with HMPAO and crosses the lipid bilayer. After crossing the liposomal membrane, HMPAO is converted into membrane impermeable hydrophilic complex by interaction with the encapsulated glutathione, resulting in the entrapment of the complex in the aqueous core [Citation51,Citation60]. This strategy can exceed 90% labeling efficiency and shows good in vivo radiolabel retention. This method has been used to prepare 99mTc-labeled liposomes to detect infection and inflammation in patients [Citation61]. Using a similar approach, liposomes have also been labeled with PET radionuclides, such as 64Cu. 64Cu is transported across the lipid bilayer by the lipophilic chelator 2-hydroxyquinoline and trapped by encapsulated DOTA, resulting in stably radiolabeled liposomes [Citation56]. 99mTc can also be complexed with N,N-bis(2-mercaptoethyl)-N′,N′-diethyl-ethylenediamine (BMEDA), which is transported across the membrane in the same manner, but entrapment of the radioactive complex is based on a pH gradient. The amino groups of the complex are protonated in the acidic aqueous core of the liposomes, which traps the complex inside the liposomes [Citation5].
4.2.3. Radiolabeling of preformed liposomes using surface chelators
The surface of liposomes can be radiolabeled by exposure of chelators on the lipid bilayer. In this convenient method, a phospholipid is derivatized with a chelator and is incorporated in the lipid bilayer during preparation of the liposomes. Phospholipids, such as distearoylphosphatidylethanolamine (DSPE), have been derivatized with chelators such as DOTA, hydrazinonicotinamide (HYNIC) or DTPA. Subsequently, these liposomes can be incubated with 64Cu, 99mTc, or 111In, respectively, which results in >95% labeling efficiency and excellent radiolabel retention [Citation49,Citation59].
To use a radiolabeled liposomal formulation for diagnostic or therapeutic purposes, efficient and stable labeling of the liposomes is required. Preferably, the method to label the liposomes is a one-step procedure and can be carried out within 1 h, with minimal radiation exposure for the technical personnel during preparation. Nowadays, passive encapsulation of the radionuclide into the liposomes during preparation is barely used. Low-labeling efficiencies, the necessity of a purification step, radioactive contamination of the equipment, and the need of a fresh batch of liposomes make this process a laborious method. Although labeling efficiency is higher when the liposomal membrane is labeled, it is still not optimal because purification of the radiolabeled product is necessary, as well as a fresh batch of liposomes should be prepared each experiment. Therefore, remote labeling methods are preferred.
In remote labeling methods preformed liposomes are used, making the method less time-consuming and less laborious. Efficient labeling has been shown for all remote labeling methods. However, liposomes with a chelator incorporated in the lipid bilayer can be labeled most efficiently, with high-specific activity (up to 15 GBq/mmol for 111In–DTPA–PEGylated liposomes), high radiochemical stability and without affecting the in vivo behavior or biodistribution. The simplicity of the preparation, efficient labeling, and sufficient radiochemical stability make the use of surface chelation the most convenient method to use.
5. Radionuclide imaging with radiolabeled liposomes
SPECT and PET imaging allows non-invasive monitoring of the in vivo pharmacokinetics and tissue distribution of the liposomes. Radionuclide imaging allows for longitudinal monitoring of their behavior in animals, as well as in patients. In addition to tracking radiolabeled liposomes, the liposomal payload can be radiolabeled to monitor the fate of encapsulated drugs. Potentially, these techniques may play an important role in translating liposomal drug formulations into clinical practice. In addition, the approach could be used to predict target localization and possibly therapeutic effects of liposomal drugs which could help personalize therapy for individual patients. SPECT and PET imaging studies with radiolabeled liposomes are discussed in the following.
6. SPECT imaging with radiolabeled liposomes
SPECT imaging with radiolabeled liposomes has been applied in a broad range of animal models: multiple types of cancers, imaging of infection, inflammation, artherosclerotic plaques, and blood pool imaging [Citation62,Citation63]. With their half-life of, respectively, 6 h and 2.8 days, 99mTc and 111In are well suited to monitor the fate of liposomes in vivo using SPECT imaging, since liposomes usually have a half-life of less than 24 h in rodents and 48 h in humans [Citation64–Citation67]. The only liposome-based radiotracer formulation that was used in late-stage clinical trials was a liposomal formulation radiolabeled with 111In, which was enabled by incorporation of the ionophore A23187 in the lipid bilayer and encapsulation of NTA as chelator [Citation68]. Clinical studies with this formulation showed that liposomes containing phospholipids and cholesterol were well tolerated and that a wide variety of tumors could be imaged. Imaging studies in cancer patients showed high specificity (>95%). However, due to lack of sensitivity (~70%) to detect known tumor lesions, this diagnostic tool was never approved by the FDA [Citation69–Citation71]. Although never commercialized, it provided in-depth insight in the in vivo behavior of liposomes in cancer patients and paved the road for the development of new radiolabeled liposomal formulations. SPECT imaging of PEG-coated and 111In-labeled liposomes was performed to visualize tumor lesions in different types of locally advanced cancer (head and neck, breast, bronchus and cervix cancer, and glioma patients). Uptake in the tumors was highest in patients with head and neck cancers (33.0 ± 15.8% ID/kg (percentage of injected dose/kg)), while uptake in long tumors was 18.3 ± 5.7% ID/kg, and the breast cancers showed relatively low uptake levels (5.3 ± 2.6% ID/kg). They showed that radiolabeled PEGylated liposomes accumulate in solid tumors and remain there for prolonged periods [Citation72].
Besides studies focusing on cancer imaging, SPECT imaging with radiolabeled liposomes was also applied to image infectious and inflammatory foci. As early as the late 1980s, radiolabeled liposomes were shown to localize preferentially in inflamed tissues [Citation61,Citation73–Citation77]. 99mTc-labeled PEGylated-liposomes showed superior performance as vehicles for scintigraphic imaging of inflamed joints in rats with experimental arthritis and showed increased targeting compared to non-PEGylated liposomes [Citation74,Citation78]. PEGylated liposomal formulations have also been used to detect inflammatory foci in patients. These studies showed that the use of radiolabeled liposomes for scintigraphic imaging of infection and inflammation was safe, sensitive and specific. In addition, such radiolabeled liposomes were used to image arthritic lesions in patients with rheumatoid arthritis [Citation61,Citation72,Citation74–Citation76]. These studies indicated that radiolabeled liposomes can be used to predict their potential for drug delivery by determining their accumulation in arthritic joints.
Liposomes were efficiently labeled with 99mTc with high-specific activity using HYNIC as a surface chelator. This allowed the use of a very low lipid dose. Interestingly, scintigraphic imaging showed that these liposomes were rapidly cleared from the circulation when these very low lipid doses were administrated. This was shown in rats and mice with a focal Escherichia coli infection as well as in 4 patients suspected of infection or inflammation and would not be clear without SPECT imaging [Citation79]. SPECT imaging with 111In-labeled PEG-liposomes was used to visualize Staphylococcus Aureus infection in mice () and rabbits [Citation49,Citation62]. The latter study demonstrated that the accumulation of the liposomes in the infected tissue could be visualized with SPECT up to 72 h after administration of 111In-labeled PEG-liposomes [)]. More recently, SPECT imaging contributed to gain insight in the in vivo behavior of newly developed liposomal formulations [Citation63,Citation65]. Artherosclerotic plaques are characterized by infiltrating macrophages. These macrophages recognize phosphatidylserine (PS). In an attempt to visualize vulnerable plaques, 111In-labeled PS-modified liposomes were investigated. SPECT imaging with PS-coated liposomes visualized artherosclerotic plaques in apolipoprotein E-deficient (apoE2/2) mice as well as in Watanabe heritable hyperlipidemic rabbits. Surprisingly, in this study, imaging showed that PEGylation of the liposomes did not improve targeting properties and that larger liposomes showed higher accumulation in the macrophages as compared to smaller vesicles [Citation63].
Figure 4. MicroSPECT/CT images of a mouse with a S. Aureus abscess in the left thigh muscle. Images were acquired at 1 h, 4 h, 24 h, 48 h and 72 h after injection of 111In-labeled PEGylated liposomes. At all time points uptake in the abscess and the characteristic splenic and hepatic uptake is visualized (arrows) (Reproduced with permission from van der Geest et al. [Citation49]).
![Figure 4. MicroSPECT/CT images of a mouse with a S. Aureus abscess in the left thigh muscle. Images were acquired at 1 h, 4 h, 24 h, 48 h and 72 h after injection of 111In-labeled PEGylated liposomes. At all time points uptake in the abscess and the characteristic splenic and hepatic uptake is visualized (arrows) (Reproduced with permission from van der Geest et al. [Citation49]).](/cms/asset/2cf9987f-0b49-4833-af2a-9360ce2c3f65/iedd_a_1205584_f0004_oc.jpg)
6.1. PET imaging with radiolabeled liposomes
PET imaging with radiolabeled liposomes has been studied less frequently as compared to SPECT imaging and has only been carried out in animal models. The well-known positron emitters for PET imaging, 18F and 68Ga, have been used successfully to radiolabel liposomes and to image different types of cancers. 18F and 68Ga-labeled liposomes showed stable radiolabel retention in vivo and their accumulation in tumor lesions was sufficient to visualize tumor lesions with PET imaging [Citation80–Citation82]. However, the biological half-life of PEGylated liposomes is generally between 24 and 48 h. Even though tumors could be visualized with 18F and 68Ga-labeled liposomes, the short half-life of these radionuclides (110 and 68 min, respectively), limits the duration of examination with PET. To be able to image at later time points to evaluate the dynamics of the accumulation in tumors, liposomes labeled with radionuclides with longer half-lives should be used. Liposomes labeled with 64Cu (t½ = 12.7 h) and 89Zr (t½ = 78.4 h) have been developed. These liposomes allow for tracking of liposomes for a longer period of time, up to 2 weeks after injection for 89Zr-labeled liposomes. PEG-liposomes labeled with 64Cu were used to evaluate PET imaging of cancer lesions and tumor-associated macrophages [Citation56,Citation83,Citation84]. In these studies, 64Cu-labeled PEGylated liposomes appeared stable in the circulation and accumulated efficiently and in tumor lesions. In addition, it was shown that after mannosylation of these liposomes, the liposomes were actively taken up by tumor associated macrophages (). This resulted in high-quality images up to 24-h post injection. Dosimetric analysis indicated an effective dose of 3.3 × 10–2 mSv/MBq for 64Cu-labeled PEGylated-liposomes, which would be acceptable for using these liposomes as a diagnostic imaging agent in patients [Citation84]. Liposomes have been labeled with 89Zr to evaluate their pharmacokinetics over a week with PET [Citation6]. Recently, PEGylated-liposomes labeled with 89Zr as well as with gadolinium ions have been developed. This resulted in a liposomal formulation that enabled combined PET and MR imaging. In addition, these liposomes were surface-coated with octreotide, a peptide targeting the human somatostatin receptor type II, for specific tumor targeting [Citation85]. In vitro serum protein studies and bone uptake of 89Zr uptake indicated limited stability of these radiolabeled liposomes. Still, dual PET/MR tracking of the liposomes was feasible and specific tumor uptake was observed. This provided proof of principle for ability to monitor the targeting properties of the liposomal formulation using this multimodal approach.
Figure 5. MicroPET/CT-images of a mouse bearing human colon adenocacinoma (HT29, arrows) on their right and left flank. Image acquisition was performed 24 h post injection of 64Cu-labelled PEGylated liposomes (a) Coronal PET image showing both tumours (arrows). The characteristic uptake of liposomes in spleen and liver is also visualized (arrowheads). (b) Axial PET image (top) and PET/CT image (bottom), showing both tumours (arrows) (Reproduced with permission from Petersen et al. [Citation56]).
![Figure 5. MicroPET/CT-images of a mouse bearing human colon adenocacinoma (HT29, arrows) on their right and left flank. Image acquisition was performed 24 h post injection of 64Cu-labelled PEGylated liposomes (a) Coronal PET image showing both tumours (arrows). The characteristic uptake of liposomes in spleen and liver is also visualized (arrowheads). (b) Axial PET image (top) and PET/CT image (bottom), showing both tumours (arrows) (Reproduced with permission from Petersen et al. [Citation56]).](/cms/asset/3dff9a7e-6365-4c7f-94e3-fbc518af7ba6/iedd_a_1205584_f0005_oc.jpg)
Only preclinical studies demonstrated the potential of using radiolabeled liposomes for PET imaging. So far, to our knowledge, no clinical studies have yet been published that include PET imaging to monitor radiolabeled liposomes.
7. Theranostic liposomes
Radiolabeled drug-loaded liposomes can be applied simultaneously for drug delivery and radionuclide imaging. The combination of a diagnostic agent and a therapeutic agent results in a theranostic compound. The most obvious way to conceive theranostic liposomes is to radiolabel a drug containing liposomal formulation to monitor the liposomes and their content using imaging methods. Besides radiolabeling of drug-loaded liposomes, liposomes can be labeled with beta-emitting radionuclides, such as 90Y, 166Ho, 177Lu, 186Re, and 188Re or with alpha-emitters, such as 225Ac or 213Bi and directly function as radiopharmaceutical or enable the examination the combination of chemotherapy and radionuclide therapy. Liposomes can be labeled with these radionuclides using similar methods as described above (2: radiolabeling methods). However, in vivo behavior of these theranostic agents has not been widely examined.
Chow et al. demonstrated that 111In-labeled vinorelbine–liposomes showed that accumulation in human colorectal carcinoma HT-29/luc xenografts in mice could be visualized using scintigraphy. In addition, they showed that tumor growth could be inhibited by the combination therapy of 111In-labeled vinorelbine–liposomes and unlabeled vinorelbine–liposomes. They demonstrated the possibility to provide diagnostic and therapeutic efficacy evaluation using a single theranostic liposomal formulation [Citation86].
The first and only clinical study including radiolabeled drug-loaded liposomes was performed with 99mTc-DTPA-labeled doxorubicin loaded PEGylated liposomes to image uptake in patients with glioblastomas and metastatic brain tumors [Citation87]. The authors claimed successful accumulation of the radiolabeled drug in tumor tissue suggesting that doxorubicin loaded PEGylated liposomes may increase the effectiveness of the (radio) therapy for brain tumors. However, there are some major concerns about the labeling methods that have been used in this study [Citation88]. The method is based on the assumption that adding 99mTc-DTPA to PEGylated liposomes results in radiolabeled liposomes. However, due to the lack of any driving force to escort 99mTc-DTPA through the lipid bilayer, this method might reveal an unstable radiolabeled product. In addition, the applied quality control method did not distinguish between 99mTc-DTPA and 99mTc-DTPA-liposomes [Citation61,Citation72]. A control scan with 99mTc-DTPA could show that the scan with 99mTc-DTPA-labeled doxorubicin loaded PEGylated liposomes represent the accumulation of the liposomes instead of free 99mTc-DTPA.
The same doxorubicin loaded PEGylated liposomes have been radiolabeled with 99mTc via the direct labeling method using BMEDA as a chelator to image pharmacokinetic and non-invasive image studies. They showed that the in vivo biodistribution and pharmacokinetics of 99mTc-labeled doxorubicin loaded PEGylated liposomes could be determined using scintigraphic imaging. In this study, the therapeutic effects were not examined, since only healthy animals were used. Nevertheless, they showed that this labeling method results in a radiochemical stable 99mTc-labeled product, which may be extended to radiolabeling with 186Re and 188Re [Citation5]. This method has been used in preclinical studies that show that 188Re-labeled PEG-liposomes inhibit the growth of human non-small cell lung carcinoma (NCL-H292) and glioma xenografts [Citation89,Citation90]. In addition, Soundararajan et al. labeled liposomal doxorubicin with 186Re, showing that the combination therapy was more effective in inhibiting growth of head and neck tumors than liposomal doxorubicin alone [Citation91]. PEGylated-liposomes were also radiolabeled with 177Lu. Based on preclinical dosimetric studies in mice with human tumor xenografts, the authors estimated that a high-radiation dose could be delivered to tumor lesions (114 mGy/MBq). Since, 177Lu, 186Re, and 188Re emit both ß-particles as well as γ-photons, labeling of liposomes with these radionuclides result directly in theranostic agents [Citation92].
The use of α-emitting radionuclides to deposit highly focused energy can cause efficient elimination of particularly single cells and micrometastases with limited toxicity to surrounding normal tissues. Immunoliposomes labeled with 213Bi have shown to be effective in mice with early-stage breast cancer metastases. Median survival times were similar to those obtained with antibody-mediated delivery of 213Bi. Although the tumor absorbed dose (1.9 ± 0.6 Gy) induces a therapeutic effect, the radiation dose in other organs was disturbing, e.g. the spleen (28 ± 16 Gy) [Citation58].
Proper evaluation of the potential risks of the radiotherapeutic liposomes labeled with high-energy ß- and α-emitters is mandatory. Dosimetry studies are essential to estimate the radiation doses to various non-target organs, such as liver and spleen, in which significant uptake of liposomes is expected. Liposomal delivery of high-energy ß- and α-emitters for therapeutic purposes merits further consideration prior to clinical implementation.
8. Conclusion
The developments in the field of liposomes lead to several different radiolabeling approaches, resulting in increased labeling efficiency, radiochemical purity, and stability in vitro and in vivo. Which method is optimal depends on the application, the choice of radionuclide and the type of liposome. In general, labeling of liposomes via a chelator incorporated in the lipid bilayer, results in efficient labeling with high-specific activity, good in vivo stability and unaltered in vivo behavior. Several types of cancer, infectious foci and inflammatory tissue have been successfully visualized using SPECT and PET imaging after administration of the radiolabeled liposomes. Studies with radiolabeled liposomes have provided important insights in the in vivo behavior and targeting properties of liposomal drug formulations. Most importantly, radiolabeled liposomes could be used to predict the therapeutic efficacy of drug-loaded liposomes. However, the theranostic applications of liposomes have not been clinically exploited yet. Non-invasive imaging of the in vivo targeting of the radiolabeled liposomes could predict the therapeutic efficacy of the liposomal drug formulation and could thus be used to steer treatment in the individual patient.
9. Expert opinion
The use of liposomes as drug delivery system intends to increase the efficacy of therapeutic agents by overcoming chemical, physical and biological hurdles that lead to inadequate drug delivery. Improved target site accumulation and reduced exposure of healthy tissue, may widen the therapeutic window of liposome-loaded drugs. The straightforward preparation, biodegradability and biocompatibility of liposomal preparations have bolstered a continuous progress in liposomal technology. This resulted in the development of several formulations for the delivery of anti-infective (amphotericin B), anti-inflammatory (prednisolone sodium phosphate (PEGylated)), and anticancer drugs (doxorubicin, cytarabine, and vincristine) [Citation93–Citation96].
In combination with stable and efficient radiolabeling procedures, liposomes could be excellent tools to optimize therapy with drug-loaded liposomes. By radiolabeling the liposomal formulation, the pharmacokinetics and targeting to the diseased tissues can be monitored noninvasively. Uptake of non-targeted liposomes in diseased tissue is primarily driven by the EPR effect, so these liposomes will non-specifically accumulate in tissue with increased vascular permeability, such as inflamed and cancerous tissue. PET and SPECT imaging allows for quantitative determination of the concentration and the residence time of the radiolabeled liposomes in the diseased tissue. Based on the imaging results, the liposomal formulation can be optimized for the particular application to maximize the exposure of the target tissue to the drug and to minimize the exposure of vulnerable healthy tissues. However, the need of a multidisciplinary approach to use radiolabeled liposomes in diagnosis might hamper the implementation of liposomes into the clinic.
While the therapeutic effects of drug-loaded liposomes and the imaging properties of radiolabeled liposomes are widely examined, the theranostic application of radiolabeled liposomes are barely exploited. Regardless of the promising results in animal studies, no radiolabeled drug-loaded liposomal formulation has been properly investigated in patients yet. The reason for the lack of clinical studies examining the potential of theranostic liposomes remains unclear. One of the reasons might be that the costs to develop a successful theranostic formulation are still too high. To gain interest of the pharmaceutical industry to produce new liposomal formulation, the production process should be profitable. Unfortunately, the limited application of theranostic liposomes results in a relatively small production scale, which makes it challenging to optimize the production process and lower the costs.
Besides costs, other factors need to be improved to make clinical studies more attractive to perform, such as batch to batch reproducibility, drug entrapment, specificity and (radio)chemical stability. Batch to batch variation should be minimized to obtain reliable and reproducible results. Currently, lipid dose and the amount of non-encapsulated drug in de liposomal solutions may still differ from batch to batch. This may affect circulation time, therapeutic efficacy and radiolabeling efficiency. Efficient drug loading is mandatory to allow for the administration of sufficient amount of drugs or, in case of radiotherapy, radiation dose to the diseased tissue. Hydrophilic drugs can be efficiently encapsulated when dissolved in the aqueous solution in which the liposomes are formed. Poorly soluble (hydrophobic) drugs can be efficiently encapsulated after preloading in an ionizable cyclodextrin, which enables its water solubility and permits encapsulation via the aqueous solution. In addition, hydrophilic drugs can also be encapsulated via active loading using weak acidic or basic amphiphilic drugs. However, active loading methods are not used to load lipophilic drugs into the lipid bilayer.
Another factor that could be improved is the stability of the liposomal formulation. Physical stability and reactivity after systemic administration has been much improved with the development of PEGylated liposomes. However, chemical stability is not assured, since hydrolysis of the ester bonds and oxidation of the acyl chains of the lipids can occur and it is not known how these processes affect radiolabeling of the liposomes. In addition, radiochemical stability of the radiolabeled drug-loaded liposomes should be assessed in a clinical setting, since only preclinical data is available. To allow for clinical studies with radiolabeled liposomes for radionuclide therapy, preclinical toxicity and dosimetry should be evaluated extensively first. Non-target organs, such as liver and spleen could be severely damaged since a significant amount of the PEGylated liposomes will accumulate in these organs.
There is still a lot to learn and to optimize the potential theranostic application of radiolabeled drug-loaded liposomes. Nevertheless, the results so far show promising results that emphasize the potential of radiolabeled liposomes to play a role in diagnosis of disease by tracking the liposomes and their content using imaging techniques, simultaneously with the delivery of their therapeutic content. However, there is a strong need for studies that could demonstrate whether the results found in animal studies translate to humans and whether clinical implementation would be feasible.
Article highlights
Liposomes can be efficiently radiolabelled to monitor their in vivo distribution after i.v. administration
Liposomes can be radiolabelled with radionuclides which allow for either PET (Ga-68, Cu-64, Zr-89) or SPECT (Tc-99m, In-111) imaging
Remote labeling methods using chelating agents incorporated in the lipid bilayer are preferred, because they allow efficient one-step labeling and reveal stable preparations
For theranostic purposes drug-loaded liposomes can be radiolabelled with radionuclides for imaging (In-111) and therapy (Y-90, Lu-177)
Radiolabeling the liposomes and/or the encapsulated drug can reveal important insights in the in vivo fate of liposomal drug formulations
This box summarizes key points contained in the article.
Declaration of interest
JM Metselaar is CEO of Enceladus Pharmaceuticals. The authors have no other relevant affiliations or financial involvement with any organization or entity with a financial interest in or financial conflict with the subject matter or materials discussed in the manuscript apart from those disclosed.
Additional information
Funding
References
- Bangham AD, Standish MM, Watkins JC. Diffusion of univalent ions across the lamellae of swollen phospholipids. J Mol Biol. 1965 Aug;13:238–252.
- Petersen AL, Hansen AE, Gabizon A, et al. Liposome imaging agents in personalized medicine. Adv Drug Deliv Rev. 2012 Oct;64:1417–1435.
- Bozzuto G, Molinari A. Liposomes as nanomedical devices. Int J Nanomedicine. 2015;10:975–999.
- Schiffelers RM, Banciu M, Metselaar JM, et al. Therapeutic application of long-circulating liposomal glucocorticoids in auto-immune diseases and cancer. J Liposome Res. 2006;16:185–194.
- Bao A, Goins B, Klipper R, et al. Direct 99mTc labeling of pegylated liposomal doxorubicin (Doxil) for pharmacokinetic and non-invasive imaging studies. J Pharmacol Exp Ther. 2004 Feb;308:419–425.
- Seo JW, Mahakian LM, Tam S, et al. The pharmacokinetics of Zr-89 labeled liposomes over extended periods in a murine tumor model. Nucl Med Biol. 2015 Feb;42:155–163.
- Zielhuis SW, Seppenwoolde J-H, Mateus VAP, et al. Lanthanide-loaded liposomes for multimodality imaging and therapy. Cancer Biother Radiopharm. 2006 Oct;21:520–527.
- Phillips WT, Goins BA, Bao A. Radioactive liposomes. Wiley Interdiscip Rev Nanomed Nanobiotechnol. 2009 Jan–Feb;1:69–83.
- Liu D, Mori A, Huang L. Role of liposome size and RES blockade in controlling biodistribution and tumor uptake of GM1-containing liposomes. Biochim Biophys Acta. 1992 Feb 17;1104:95–101.
- Chang H-I, Yeh M-K. Clinical development of liposome-based drugs: formulation, characterization, and therapeutic efficacy. Int J Nanomedicine. 2012;7:49–60.
- Allen TM, Cullis PR. Liposomal drug delivery systems: from concept to clinical applications. Adv Drug Deliv Rev. 2013 Jan;65:36–48.
- Wang X, Song Y, Su Y, et al. Are PEGylated liposomes better than conventional liposomes? A special case for vincristine. Drug Deliv. 2015 Jun;2:1–9.
- Jahn F, Jordan K, Behlendorf T, et al. Safety and efficacy of liposomal cytarabine in the treatment of neoplastic meningitis. Oncology. 2015;89:137–142.
- Cedervall T, Lynch I, Foy M, et al. Detailed identification of plasma proteins adsorbed on copolymer nanoparticles. Angewandte Chemie. 2007;46:5754–5756.
- Labarre D, Vauthier C, Chauvierre C, et al. Interactions of blood proteins with poly(isobutylcyanoacrylate) nanoparticles decorated with a polysaccharidic brush. Biomaterials. 2005 Aug;26:5075–5084.
- Lundqvist M, Stigler J, Elia G, et al. Nanoparticle size and surface properties determine the protein corona with possible implications for biological impacts. Proc Natl Acad Sci U S A. 2008 Sep 23;105:14265–14270.
- Johnstone SA, Masin D, Mayer L, et al. Surface-associated serum proteins inhibit the uptake of phosphatidylserine and poly(ethylene glycol) liposomes by mouse macrophages. Biochim Biophys Acta. 2001 Jul 2;1513:25–37.
- Chow T-H, Lin -Y-Y, Hwang -J-J, et al. Therapeutic efficacy evaluation of 111In-labeled PEGylated liposomal vinorelbine in murine colon carcinoma with multimodalities of molecular imaging. J Nucl Med. 2009 Dec;50:2073–2081.
- Schipper ML, Iyer G, Koh AL, et al. Particle size, surface coating, and PEGylation influence the biodistribution of quantum dots in living mice. Small. 2009 Jan;5:126–134.
- Sheng Y, Yuan Y, Liu C, et al. In vitro macrophage uptake and in vivo biodistribution of PLA-PEG nanoparticles loaded with hemoglobin as blood substitutes: effect of PEG content. J Mater Sci Mater Med. 2009 Sep;20:1881–1891.
- Ning YM, He K, Dagher R, et al. Liposomal doxorubicin in combination with bortezomib for relapsed or refractory multiple myeloma. Oncology (Williston Park). 2007 Nov;21:1503–1508; discussion 11, 13, 16 passim.
- Hua S, Cabot PJ. Targeted nanoparticles that mimic immune cells in pain control inducing analgesic and anti-inflammatory actions: a potential novel treatment of acute and chronic pain conditions. Pain Physician. 2013 May–Jun;16:199–216.
- ElBayoumi TA, Torchilin VP. Tumor-targeted nanomedicines: enhanced antitumor efficacy in vivo of doxorubicin-loaded, long-circulating liposomes modified with cancer-specific monoclonal antibody. Clin Cancer Res. 2009 Mar 15;15:1973–1980.
- Iwase Y, Maitani Y. Octreotide-targeted liposomes loaded with CPT-11 enhanced cytotoxicity for the treatment of medullary thyroid carcinoma. Mol Pharm. 2011 Mar–Apr;8:330–337.
- Iwase Y, Maitani Y. Dual functional octreotide-modified liposomal irinotecan leads to high therapeutic efficacy for medullary thyroid carcinoma xenografts. Cancer Sci. 2012 Feb;103:310–316.
- Ahmad I, Allen TM. Antibody-mediated specific binding and cytotoxicity of liposome-entrapped doxorubicin to lung cancer cells in vitro. Cancer Res. 1992 Sep 1;52:4817–4820.
- Ahmad I, Longenecker M, Samuel J, et al. Antibody-targeted delivery of doxorubicin entrapped in sterically stabilized liposomes can eradicate lung cancer in mice. Cancer Res. 1993 Apr 1;53:1484–1488.
- Maruyama K. PEG-immunoliposome. Biosci Rep. 2002 Apr;22:251–266.
- Sercombe L, Veerati T, Moheimani F, et al. Advances and challenges of liposome assisted drug delivery. Front Pharmacol. 2015;6:286.
- Daemen T, Hofstede G, Ten Kate MT, et al. Liposomal doxorubicin-induced toxicity: depletion and impairment of phagocytic activity of liver macrophages. Int J Cancer. 1995 May 29;61:716–721.
- Rosenthal MS, Cullom J, Hawkins W, et al. Quantitative SPECT imaging: a review and recommendations by the focus committee of the society of nuclear medicine computer and instrumentation council. J Nucl Med. 1995 Aug;36:1489–1513.
- Van Der Have F, Vastenhouw B, Ramakers RM, et al. U-SPECT-II: an ultra-high-resolution device for molecular small-animal imaging. J Nucl Med. 2009 Apr;50:599–605.
- Lee SY, Jeon SI, Jung S, et al. Targeted multimodal imaging modalities. Adv Drug Deliv Rev. 2014 Sep 30;76:60–78.
- Allen TM, Hansen C. Pharmacokinetics of stealth versus conventional liposomes: effect of dose. Biochim Biophys Acta. 1991 Sep 30;1068:133–141.
- Unezaki S, Maruyama K, Takahashi N, et al. Enhanced delivery and antitumor activity of doxorubicin using long-circulating thermosensitive liposomes containing amphipathic polyethylene glycol in combination with local hyperthermia. Pharm Res. 1994 Aug;11:1180–1185.
- Srivatsan A, Chen X. Recent advances in nanoparticle-based nuclear imaging of cancers. Adv Cancer Res. 2014;124:83–129.
- McDougall IR, Dunnick JK, Goris ML, et al. In vivo distribution of vesicles loaded with radiopharmaceuticals: a study of different routes of administration. J Nucl Med. 1975 Jun;16:488–491.
- Caride VJ. Technical and biological considerations on the use of radiolabeled liposomes for diagnostic imaging. Int J Rad Appl Instrum B. 1990;17:35–39.
- McDougall IR, Dunnick JK, Goris ML, et al. In vivo distribution of vesicles loaded with radiopharmaceuticals: a comparison of different routes of administration. Scott Med J. 1975 Jan;20:39.
- Oku N, Tokudome Y, Tsukada H, et al. In vivo trafficking of long-circulating liposomes in tumour-bearing mice determined by positron emission tomography. Biopharm Drug Dispos. 1996 Jul;17:435–441.
- Marik J, Tartis MS, Zhang H, et al. Long-circulating liposomes radiolabeled with [18F]fluorodipalmitin ([18F]FDP). Nucl Med Biol. 2007 Feb;34:165–171.
- Caride VJ, Taylor W, Cramer JA, et al. Evaluation of liposome-entrapped radioactive tracers as scanning agents. Part 1: organ distribution of liposome (99mTc-DTPA) in mice. J Nucl Med. 1976 Dec;17:1067–1072.
- Henriksen JR, Petersen AL, Hansen AE, et al. Remote loading of (64)Cu(2+) into liposomes without the use of ion transport enhancers. ACS Appl Mater Interfaces. 2015 Oct 21;7:22796–22806.
- Mauk MR, Gamble RC. Preparation of lipid vesicles containing high levels of entrapped radioactive cations. Anal Biochem. 1979 Apr 15;94:302–307.
- Beaumier PL, Hwang KJ. An efficient method for loading indium-111 into liposomes using acetylacetone. J Nucl Med. 1982 Sep;23:810–815.
- Tilcock C, Yap M, Szucs M, et al. PEG-coated lipid vesicles with encapsulated technetium-99m as blood pool agents for nuclear medicine. Nucl Med Biol. 1994 Feb;21:165–170.
- Chang M-Y, Seideman J, Sofou S. Enhanced loading efficiency and retention of 225Ac in rigid liposomes for potential targeted therapy of micrometastases. Bioconjug Chem. 2008 Jun;19:1274–1282.
- Gabizon A, Papahadjopoulos D. Liposome formulations with prolonged circulation time in blood and enhanced uptake by tumors. P Natl Acad Sci USA. 1988 Sep;85:6949–6953.
- van der Geest T, Laverman P, Gerrits D, et al. Comparison of three remote radiolabelling methods for long-circulating liposomes. J Control Release. 2015 Dec 28;220:239–244.
- Woodle MC. Gallium-67-labeled liposomes with prolonged circulation - preparation and potential as nuclear imaging agents. Nucl Med Biol. 1993 Feb;20:149–155.
- Bao A, Goins B, Klipper R, et al. A novel liposome radiolabeling method using 99mTc-“SNS/S” complexes: in vitro and in vivo evaluation. J Pharm Sci. 2003 Sep;92:1893–1904.
- Dams ET, Oyen WJ, Boerman OC, et al. Technetium-99m-labeled PEG-liposomes for the detection of infection and inflammation. J Nucl Med. 1999 May;40:15p–15p.
- Bao A, Goins B, Klipper R, et al. 186Re-liposome labeling using 186Re-SNS/S complexes: in vitro stability, imaging, and biodistribution in rats. J Nucl Med. 2003 Dec;44:1992–1999.
- Phillips WT, Goins B, Bao A, et al. Rhenium-186 liposomes as convection-enhanced nanoparticle brachytherapy for treatment of glioblastoma. Neuro Oncol. 2012 Apr;14:416–425.
- Chen L-C, Chang C-H, Yu C-Y, et al. Biodistribution, pharmacokinetics and imaging of (188)Re-BMEDA-labeled pegylated liposomes after intraperitoneal injection in a C26 colon carcinoma ascites mouse model. Nucl Med Biol. 2007 May;34:415–423.
- Petersen AL, Binderup T, Rasmussen P, et al. 64Cu loaded liposomes as positron emission tomography imaging agents. Biomaterials. 2011 Mar;32:2334–2341.
- Seo JW, Zhang H, Kukis DL, et al. A novel method to label preformed liposomes with 64Cu for positron emission tomography (PET) imaging. Bioconjug Chem. 2008 Dec;19:2577–2584.
- Lingappa M, Song H, Thompson S, et al. Immunoliposomal delivery of 213Bi for alpha-emitter targeting of metastatic breast cancer. Cancer Res. 2010 Sep 1;70:6815–6823.
- Laverman P, Dams ETM, Oyen WJG, et al. A novel method to label liposomes with 99mTc by the hydrazino nicotinyl derivative. J Nucl Med. 1999 Jan;40:192–197.
- Goins B, Bao A, Phillips WT. Techniques for loading technetium-99m and rhenium-186/188 radionuclides into pre-formed liposomes for diagnostic imaging and radionuclide therapy. Methods Mol Biol. 2010;606:469–491.
- Dams ET, Oyen WJ, Boerman OC, et al. 99mTc-PEG liposomes for the scintigraphic detection of infection and inflammation: clinical evaluation. J Nucl Med. 2000 Apr;41:622–630.
- Boerman OC, Laverman P, Oyen WJG, et al. Radiolabeled liposomes for scintigraphic imaging. Prog Lipid Res. 2000 Sep;39:461–475.
- Ogawa M, Uchino R, Kawai A, et al. PEG modification on (111)In-labeled phosphatidyl serine liposomes for imaging of atherosclerotic plaques. Nucl Med Biol. 2015 Mar;42:299–304.
- Lin YY, Kao HW, Li JJ, et al. Tumor burden talks in cancer treatment with PEGylated liposomal drugs. PLoS One. 2013;8:e63078.
- Ogawa M, Umeda IO, Kosugi M, et al. Development of 111In-labeled liposomes for vulnerable atherosclerotic plaque imaging. J Nucl Med. 2014 Jan;55:115–120.
- Turker S, Erdogan S, Ozer AY, et al. Scintigraphic imaging of radiolabelled drug delivery systems in rabbits with arthritis. Int J Pharm. 2005 May 30;296:34–43.
- Moghimi SM, Szebeni J. Stealth liposomes and long circulating nanoparticles: critical issues in pharmacokinetics, opsonization and protein-binding properties. Prog Lipid Res. 2003 Nov;42:463–478.
- Jensen GM, Bunch TH. Conventional liposome performance and evaluation: lessons from the development of Vescan. J Liposome Res. 2007;17:121–137. .
- Presant CA, Turner AF, Profitt RT. Potential for improvement in clinical decision-making: tumor imaging with in-111 labeled liposomes results of a phase ii-iii study. J Liposome Res. 1994 Jan;4:985–1008.
- Khalifa A, Dodds D, Rampling R, et al. Liposomal distribution in malignant glioma: possibilities for therapy. Nucl Med Commun. 1997 Jan;18:17–23.
- Kubo A, Nakamura K, Sammiya T, et al. Indium-111-labelled liposomes: dosimetry and tumour detection in patients with cancer. Eur J Nucl Med. 1993 Feb;20:107–113.
- Harrington KJ, Mohammadtaghi S, Uster PS, et al. Effective targeting of solid tumors in patients with locally advanced cancers by radiolabeled pegylated liposomes. Clin Cancer Res. 2001 Feb;7:243–254.
- Morgan JR, Williams LA, Howard CB. Technetium-labelled liposome imaging for deep-seated infection. Brit J Radiol. 1985;58:35–39.
- Boerman OC, Oyen WJ, Storm G, et al. Technetium-99m labelled liposomes to image experimental arthritis. Ann Rheum Dis. 1997 Jun;56:369–373.
- Metselaar JM, Storm G. Liposomes in the treatment of inflammatory disorders. Expert Opin Drug Deliv. 2005 May;2:465–476.
- Metselaar JM, Van Den Berg WB, Holthuysen AE, et al. Liposomal targeting of glucocorticoids to synovial lining cells strongly increases therapeutic benefit in collagen type II arthritis. Ann Rheum Dis. 2004 Apr;63:348–353.
- Ozbakir B, Crielaard BJ, Metselaar JM, et al. Liposomal corticosteroids for the treatment of inflammatory disorders and cancer. J Control Release. 2014 Sep 28;190:624–636.
- Laverman L, Boerman B, Oyen O, et al. Liposomes for scintigraphic detection of infection and inflammation. Adv Drug Deliv Rev. 1999 May 4;37:225–235.
- Laverman P, Brouwers AH, Dams ET, et al. Preclinical and clinical evidence for disappearance of long-circulating characteristics of polyethylene glycol liposomes at low lipid dose. J Pharmacol Exp Ther. 2000 Jun;293:996–1001.
- Emmetiere F, Irwin C, Viola-Villegas NT, et al. (18)F-labeled-bioorthogonal liposomes for in vivo targeting. Bioconjug Chem. 2013 Nov 20;24:1784–1789.
- Jensen ATI, Binderup T, Andresen TL, et al. PET imaging of liposomes labeled with an [¹⁸F]-fluorocholesteryl ether probe prepared by automated radiosynthesis. J Liposome Res. 2012 Dec;22:295–305.
- Helbok A, Decristoforo C, Dobrozemsky G, et al. Radiolabeling of lipid-based nanoparticles for diagnostics and therapeutic applications: a comparison using different radiometals. J Liposome Res. 2010 Sep;20:219–227.
- Locke LW, Mayo MW, Yoo AD, et al. PET imaging of tumor associated macrophages using mannose coated 64Cu liposomes. Biomaterials. 2012 Nov;33:7785–7793.
- Petersen AL, Henriksen JR, Binderup T, et al. In vivo evaluation of PEGylated (64)Cu-liposomes with theranostic and radiotherapeutic potential using micro PET/CT. Eur J Nucl Med Mol Imaging. 2016 May;43:941–952.
- Abou DS, Thorek DLJ, Ramos NN, et al. (89)Zr-labeled paramagnetic octreotide-liposomes for PET-MR imaging of cancer. Pharm Res. 2013 Mar;30:878–888.
- Chow T-H, Lin -Y-Y, Hwang -J-J, et al. Diagnostic and therapeutic evaluation of 111In-vinorelbine-liposomes in a human colorectal carcinoma HT-29/luc-bearing animal model. Nucl Med Biol. 2008 Jul;35:623–634.
- Koukourakis MI, Koukouraki S, Fezoulidis I, et al. High intratumoural accumulation of stealth liposomal doxorubicin (Caelyx) in glioblastomas and in metastatic brain tumours. Br J Cancer. 2000 Nov;83:1281–1286.
- Laverman P, Boerman OC, Storm G, et al. (99m)Tc-labelled stealth liposomal doxorubicin (Caelyx) in glioblastomas and metastatic brain tumours. Br J Cancer. 2002 Feb 12;86:659–661.
- Lin L-T, Chang C-H, Yu H-L, et al. Evaluation of the therapeutic and diagnostic effects of PEGylated liposome-embedded 188Re on human non-small cell lung cancer using an orthotopic small-animal model. J Nucl Med. 2014 Nov;55:1864–1870.
- MacKay JA, Deen DF, Szoka FC Jr. Distribution in brain of liposomes after convection enhanced delivery; modulation by particle charge, particle diameter, and presence of steric coating. Brain Res. 2005 Feb 28;1035:139–153.
- Soundararajan A, Bao A, Phillips WT, et al. [(186)Re]Liposomal doxorubicin (Doxil): in vitro stability, pharmacokinetics, imaging and biodistribution in a head and neck squamous cell carcinoma xenograft model. Nucl Med Biol. 2009 Jul;36:515–524.
- Phillips WT, Bao A, Brenner AJ, et al. Image-guided interventional therapy for cancer with radiotherapeutic nanoparticles. Adv Drug Deliv Rev. 2014 Sep 30;76:39–59.
- Dawidczyk CM, Kim C, Park JH, et al. State-of-the-art in design rules for drug delivery platforms: lessons learned from FDA-approved nanomedicines. J Control Release. 2014 Aug 10;187:133–144.
- Chamberlain MC. Neurotoxicity of intra-CSF liposomal cytarabine (DepoCyt) administered for the treatment of leptomeningeal metastases: a retrospective case series. J Neurooncol. 2012 Aug;109:143–148.
- Barratt G, Legrand P. Comparison of the efficacy and pharmacology of formulations of amphotericin B used in treatment of leishmaniasis. Curr Opin Infect Dis. 2005 Dec;18:527–530.
- Gabizon A, Shmeeda H, Barenholz Y. Pharmacokinetics of pegylated liposomal doxorubicin: review of animal and human studies. Clin Pharmacokinet. 2003;42:419–436.