ABSTRACT
Introduction
Advances in the accessibility of manufacturing technologies and iPSC-based modeling have accelerated the overall progress of organs-on-a-chip. Notably, the progress in multi-organ systems is not progressing with equal speed, indicating that there are still major technological barriers to overcome that may include biological relevance, technological usability as well as overall accessibility.
Areas covered
We here review the progress in the field of multi-tissue- and body-on-a-chip pre and post- SARS-CoV-2 pandemic and review five selected studies with increasingly complex multi-organ chips aiming at pharmacological studies.
Expert opinion
We discuss future and necessary advances in the field of multi-organ chips including how to overcome challenges regarding cell diversity, improved culture conditions, model translatability as well as sensor integrations to enable microsystems to cover organ–organ interactions in not only toxicokinetic but more importantly pharmacodynamic and -kinetic studies.
1. Introduction
Due to political incentives, the utilization of animal testing in drug development is under increasing social scrutiny. Despite the biological parallels between humans and animals, there are significant limitations regarding the ethical implications and the precise predictability of human responses based on animal studies due to species-relating variabilities [Citation1]. This has propelled the scientific community to seek alternatives that are both ethically more justifiable and also better predict human physiology responses in the context of pharmacology and toxicology. The financial demands of animal-based experiments are substantial in industrial settings. This economic strain drives the pursuit of more cost-effective methods in drug testing, without compromising the robustness and reliability of the results. Industrial and academic researchers are, therefore, incentivized to innovate more economical approaches that can expedite the drug development process without incurring exorbitant costs. Nonetheless, the dissimilarities between human and animal biology raise critical questions about the validity of animal models in drug testing [Citation2]. While animals possess certain physiological resemblances to humans, these are often outweighed by species differences, casting doubt on the direct applicability of animal study results to human health outcomes [Citation2,Citation3]. Ethical considerations in animal testing have become a focal point of debate within the scientific community, and allowed the exploration of new methodologies that maintain scientific integrity while adhering to higher ethical standards, thereby reducing, or eliminating reliance on animal testing [Citation1].
Initially, two-dimensional (2D) primary human cell cultures were seen as a viable alternative to animal testing; however, soon proved overly simplistic and lacking the necessary complexity to accurately simulate the multifaceted nature of human biology and disease states [Citation4–6]. Similarly, three-dimensional (3D) cell-based models also faced criticism over the last years for their inability to fully capture the intricacies of human physiology in its entirety. They were deemed to be an improvement over 2D models but still insufficiently representative of the human body’s structural, biochemical and biophysical complexity [Citation4,Citation6]. In response to the limitations of homotypic cell model systems, researchers have shifted toward integrating different cell models, often combining two or more organ types as heterotypic co-cultures [Citation7,Citation8]. These hybrid models aim to enhance the complexity and realism of human biological responses, bridging the gap between traditional models and the dynamic nature of human biology [Citation9–11]. The concept of a ‘body on a chip’ represents the most groundbreaking advancement in drug testing using cell-based microfluidics as multi-organs-on-a-chip. This approach that goes back to 2000 with Ghanem and Shuler’s multi-cell approaches involving already principles to later construction of miniaturized versions of the human body using human-derived cells to create a compact and complex system for drug screening [Citation12]. This innovation still holds the promise of a more accurate simulation of human responses to pharmaceuticals 25 years down the road [Citation13,Citation14].
2. Areas covered
Here, we aim to cover the recent advancing developments of the multi-organ approaches and body-on-a-chip field based on two aspects. The first aspect is how the recent SARS-CoV-2 pandemic impacted the entire organ-on-a-chip field and its publishing activity toward areas interfacing with SARS-CoV-2 as a new phenotype in a variety of human ailments. In the second part, we chose the most advancing exemplary studies focusing on toxicological or pharmacological questions to demonstrate which direction the field is heading. For the sake of framing and transparency of our comprehensive review, in our opinion multi-organ- and body-on-a-chip systems should at least consider two different tissues and three different cells types relevant to the chosen biological or pharmacological question.
2.1. A bibliographical glimpse on the pre and post-pandemic development of body-on-a-chip field
After the historical introduction of soft lithography by George Whitesides and Younan Xia [Citation15] over two decades ago, the scientific community has been using this method to create a variety of new approaches in the realms of microfluidics [Citation16–18]. The term ‘organ-on-a-chip’ saw the light in the 2010’s with developments such as the first lung-on-a-chip by the Ingber group [Citation19] with their publication titeled ’Reconstituting organ-level lung functions on a chip’ that set new standards for cell-based functional biomimicry. Due to the inherent complexity of biological systems, a single organ is not able to fully represent the response of the system. Consequently, ‘body-on-a-chip’ or ‘multi-tissue (MT) chips’ have been developed to better mimic inter-organ interaction and communication, which is particularly interesting for pharmacological studies [Citation14,Citation20,Citation21]. By incorporating components like valves, micropumps, mixers, actuators, and biosensors a complex and specific cell culture environment can be further advanced and automated using ‘lab-on-a-chip’ technologies [Citation22]. Prior to the review of current progress and advances in the organ-on-a-chip field, we conducted literature research to analyze the progression of article and review outputs in these specific fields of microfluidic research in more detail (see for Scopus.com database queries).
Table 1. Scopus.com database query terms.
Results of the research in show the rate of original and review publications on the organ- and body-on-a-chip fields. When using SCOPUS to search for all terms found that are combined under the field of organ-on-a-chip, it is possible to see that in the last decade, the number of publications has been rising steadily and the amount of articles is consistently higher than the one of reviews in the same year. Starting in the year 2020 an increase in publications associated with SARS-CoV-2 was observable. Notable is that the first reviews were made in the year 2011 since the first study that used the term organ-on-a-chip was made in 2010 [Citation19]. Before, the scientific community was already prying into the field by using terms such as integrated microfluidic systems [Citation23–25]. On the contrary, the number of publications with the keywords body- and multi-tissue-on-a-chip is slowly increasing and shows a decline starting with the year 2020 with a small amount of approx. three SARS-CoV-2-related publications per year (). Comparing the publication rates of various organ models with body and multi-tissue model, it shows that body and multi-tissue systems are the models decreasing the most after 2020 () compared to more specific organ model areas. Notably, after the development of the first lung-on-a-chip systems [Citation19], the area of respiratory systems and alveolar barriers has been growing steadily [Citation26,Citation27], with a further increase after the year 2020, caused by an influx of efforts to combat the SARS-CoV-2 pandemic as demonstrated in [Citation28]. Also, the output of liver models is increasing after 2020, while the publication rate of kidney models stayed constant since 2016. It becomes visible that lung-on-a-chip models, which ranked as the 6th common publishing topic among on-a-chip systems, became the most extensively published model after 2020 because of the amount of SARS-CoV-2-related articles. While the number of publications in the field of liver-on-a-chip stayed constantly high, with 56 and 59 published articles before and after 2020, the term distribution among the other extensively researched organ-on-a-chip systems including liver, kidney, gut, and brain (), changed after the start of the pandemic in 2020. Overall, research in the organ-on-a-chip area has consistently grown over the last 14 years. Interestingly, efforts to build body-on-a-chip systems remained at a steady output level over these years, with a decline after 2020, which can be attributed to the new focus on pandemic health topics.
Figure 1. Number of original articles and reviews published between 2010 and 2023 in the organ-on-a-chip field. (a) Publication rate of articles and reviews with the terms organ, or body and multi tissue -on-a-chip over the last decade and a half (b) and detailed view on articles and reviews for the terms body- and multi tissue-organ-on-a-chip (red area represents publications containing the keywords for SARS-CoV-2). (c) Publications of common organ-on-a-chip models over the last 24 years. (d) Term distribution of the publication number of various organ-on-a-chip models before and after the year 2020. Yellow, colored background shows the division between pre- and post-pandemic research. Information was gathered through SCOPUS query displayed in .
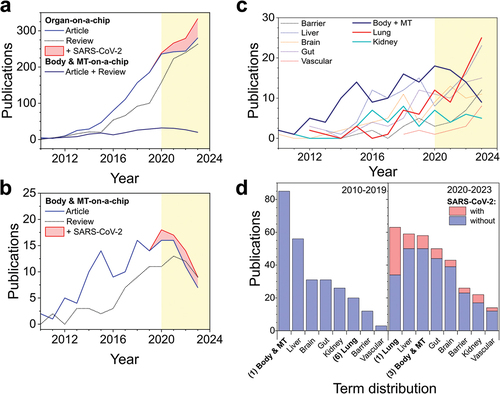
The simplest functional pharmacological organ systems for instance comprised endothelial and cardiac cell models to investigate the cardiotoxic effect of isoproterenol dosing [Citation29]. Early 3D approaches like the one introduced by Oleaga and coworkers investigated the impact of organ interconnection and divergent first pass effects of cyclophosphamide and terfenadine on cardiac beating rates [Citation30]. To advance the number of interconnected organoids, Skardal and coworkers interconnected lung, hepatic, and cardiac constructs to demonstrate how to also model unwanted off-target cardiotoxic events for a primary lung model exposed to bleomycin when adding a liver compartment [Citation31]. In turn, Oleaga and coworkers updated multi-organ chips by muscle and neuronal structures to compare known responses of five different drugs with data from animal experiments [Citation32]. Robotics as demonstrated by Nowak et al. can be exploited for further increase of the numbers of interconnected tissues (e.g. vascularized intestinal, hepatic, renal, cardiac, alveolar, dermal, neurovascular and cerebral tissue surrogates) by intermittent fluid coupling approaches, while not further increasing the complexity of individual tissue constructs [Citation33]. Another very outstanding preceding advancement in the field to use organ-on-a-chip technologies for translational cross-species studies was demonstrated by the Emulate team around Jang and Hamilton to prove that multi-species organ biochips are in fact very useful to prove the well-reviewed and poorly confirmed cross-species differences of cardiotoxic drugs [Citation34].
In the next section, we want to briefly highlight a total of five selected multi-organ studies that use distinct approaches from each other, as well as cells from different origins to create cohesive systems with increasing complexity, starting with two-organ systems and finishing on a perfused, modular six-organ system (). Notably, as a variety of reviews have already focused on general developments of the last decade in the field [Citation35–39] we only included reports from the last three years of biochip advances that in our opinion noteworthy instances due to their chip manufacturing and perfusion approaches, in order to provide a timely and very recent update of current advancements in the field of multi-organ and body-on-a-chip systems.
Table 2. Overview of the discussed papers.
2.2. Recent progress in multi-organ and body-on-a-chip technologies for toxicological and pharmacological applications
Similar to the early days of organs-on-a-chip various approaches have been reported recently, including combinations of several organs i.e. liver, kidney, heart, spleen, pancreas, the vascular system, brain as well as skin and musculoskeletal organoids in varying complexities ranging from 2D to 3D and even bio-printed tissue constructs. In a significant advancement for drug safety assessment, Yin and coworkers have developed a novel multi-organoids-on-a-chip system using human induced pluripotent stem cells (hiPSCs) for the cardiotoxic evaluation of anti-depressants (see ) [Citation40]. This innovative device enables the simultaneous evaluation of drug effects on different human organs, specifically focusing on the cardiac safety of anti-depressants post liver metabolism (also referred to as first-pass effect). The system features compartmentalized chambers, separated by a porous membrane, which house 3D human liver organoids in the upper chamber and cardiac organoids in the lower chamber, maintaining high organ model viability and organ-specific functions. Liver organoids, for instance, effectively produce albumin and urea, while the cardiac organoids demonstrate the beating function known to be a vital parameter in cardiotoxicity. Another key aspect is mimicking human metabolic processes, especially with the liver organoids due to CYP450 enzyme activity, which is crucial for drug metabolism. As proof-of-concept is exemplified with clomipramine, a common antidepressant, which undergoes metabolism in the liver organoids to form an active metabolite. This metabolite’s effects were then observed in the cardiac organoids, showing reduced viability and impaired cardiac functions, thereby indicating potential cardiotoxicity. This multi-organ-on-a-chip system represents a minimal viable product (MVP) platform aiming to revolutionize the way drug effectiveness and safety are assessed in vitro, offering a more accurate and human-relevant read-out obviating the use of animal in preclinical testing. Similarly, Shafagh and coworkers have developed a hepato-pancreatic organ-on-a-chip device that provides new insights into the complex metabolic interactions involved in prediabetic hyperglycemia [Citation41], which is characterized by aberrant glucose homeostasis, is a major risk factor for type 2 diabetes (see ). Notably, the authors developed a novel ‘synthetic heart’ approach utilizing a syringe-pump-based pneumatic actuation unit that precisely controls the perfusion of each tissue chamber essential to the understanding of liver metabolic interactions. Dynamics of insulin secretion by pancreatic islets and the hepatic response to insulin upon glucose challenge, eventually replicating physiological timescales in the absence of external hormone additions. Furthermore, leveraging activity data of 503 transcription factors revealed tissue-specific molecular networks, where liver and pancreatic islet cultures exhibited counter-regulatory transcriptional programs in response to high glucose levels. Efforts in automation and function integration for better organ model control capabilities within multi-organs-on-a-chip have been demonstrated as key for more natural response patterns. Both Yin and Shafagh’s [Citation40,Citation41] multi-tissue systems show how two organs can be cultured together on a chip while the first demonstrates a possibility for high-throughput toxicological analysis for multi-organ-on-a-chip systems, and the second shows a way to regulate flow regimes required for better approximation of chip models toward specific tissue microenvironments.
Figure 2. Overview on three multi-tissue on-a-chip models. (ai) Design of a liver-heart chip with a polycarbonate membrane between cell types. (aii) Seeding assay to create liver and heart organoids in unique compartments inside the chip. (aiii) Effect of 1 µM Clomipramine on the beating velocity of the heart spheroids after liver metabolism. (aiv) Effect of 1 µM Clomipramine on the Ca2+ ion production of the heart spheroids in different culture conditions. (bi) Seeding procedure of pancreatic islets and liver spheroids into a pneumatic chip. (bii) Overlap of differentially expressed genes in liver spheroids and pancreatic islets after high glucose exposure in chip. (ci) 3 organ-on-a-chip system developed for malaria studies together with a flow profile simulation of the system. (cii) Over-time hepatocyte morphology and viability assay (via CYP3A4 activity) while inside.
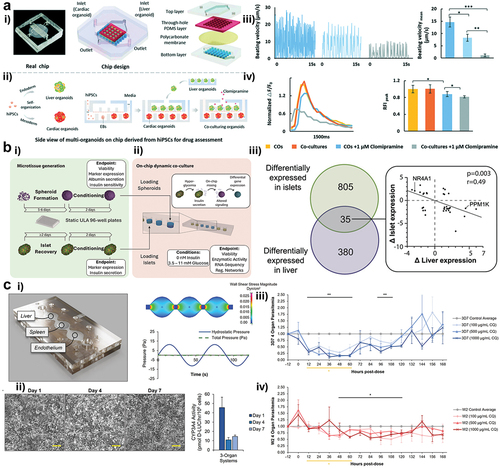
Rupar and colleagues developed a serum-free, multi-organ culture system to facilitate the study of the malaria-causing parasite Plasmodium falciparum [Citation42], aiming to advance therapeutic drug development (see ). The portrayed system shows the implementation of not only human cells but also parasites into a single multi organ-on-a-chip device, thus increasing its complexity and more closely bio-mimicking in vivo interactions. This system comprises four human organ constructs: hepatocytes (liver cells), splenocytes (spleen cells), endothelial cells, and recirculating red blood cells, accommodating the growth of both chloroquine-sensitive (3D7) and chloroquine-resistant (W2) strains of P. falciparum. Prior to parasite introduction, control experiments confirmed that the parasites could thrive under the same conditions as the human cells within the system. Using a home-brew serum-free medium called ‘Multi-Organ System Medium’ (MOSM), both P. falciparum strains were successfully cultured in this system, with regular monitoring of hematocrit and parasitemia levels over 14 days. Key experiments involved treating the 3D7 strain with chloroquine, resulting in a significant reduction in parasitemia, though with a resurgence after 5 days. In contrast, the W2 strain exhibited only a moderate decrease in parasitemia levels upon chloroquine treatment. Also, this parasitemia model can potentially enable the facile assessment of human off-target toxicity of anti-malarial treatments, rendering this advance valuable for determining therapeutic indices. Furthermore, Rajan et al. present a more complex and integrated multi-organoid system for parallel assessment of drug efficacy and toxicity across multiple iPSC-derived 3D tissue organoids [Citation43]. The system is constructed using a cost-effective, adhesive film-based microfluidic device (see ). This miniaturized platform requires less than 200 μL fluid volume and supports both matrix-based 3D cell culture and spheroid aggregate integration within an in-situ photo-cross linkable hyaluronic acid hydrogel encompassing liver, cardiac, and lung compartments with viabilities up to 21 days. The platform’s utility is validated through experiments investigating the liver metabolism of the prodrug capecitabine into 5-fluorouracil (5-FU) and, in turn, observing toxicity effects on lung and cardiac organoids. The authors also expanded system complexity to incorporate six humanized organoid constructs also including brain, vessels, and testes. After a 14-day incubation, multi-tissue interactions are demonstrated through the metabolism of the alkylating prodrug ifosfamide in the liver organoid resulting in the formation of neurotoxic chloroacetaldehyde. Notably, the replacement of the testicular compartment with female reproductive organoids would additionally allow sex-specific toxicological evaluations in the future to elaborate on gender and sex-relating treatment response differences. This system shows the complex co-culture of six different organ constructs, adding to its ability to closely represent in vivo interactions while also possessing low media and reagents volume requirements directed toward cost management.
Figure 3. Overview of a humanized multi-organ on-a-chip platform for drug metabolism and toxicity testing. (ai) Schematic designs for 3 and 6 integrated organoid systems. (aii) Pipetting assay schematics for a thiolated-hydrogel-based cell 3D culture. (aiii) Photography of a multi-organ system with external fluid flow control. (b) Viability study of the effects of ifosfamide on a 6-organoid system (bi) Counterstain of life (calcein AM) and death cells (ethidium homodimer 1) of all 6 organs in the system. condition 1 and 2 are treatment with 1 mM ifosfamide but in condition 2 the liver was removed before treatment. (bii) Quantification of life/dead staining, viability is shown as percentage. [Adapted with permission from [Citation43]].
![Figure 3. Overview of a humanized multi-organ on-a-chip platform for drug metabolism and toxicity testing. (ai) Schematic designs for 3 and 6 integrated organoid systems. (aii) Pipetting assay schematics for a thiolated-hydrogel-based cell 3D culture. (aiii) Photography of a multi-organ system with external fluid flow control. (b) Viability study of the effects of ifosfamide on a 6-organoid system (bi) Counterstain of life (calcein AM) and death cells (ethidium homodimer 1) of all 6 organs in the system. condition 1 and 2 are treatment with 1 mM ifosfamide but in condition 2 the liver was removed before treatment. (bii) Quantification of life/dead staining, viability is shown as percentage. [Adapted with permission from [Citation43]].](/cms/asset/f76e7807-d6aa-4088-926a-cb8639d8bbaf/iemt_a_2362183_f0003_oc.jpg)
A final study elaborated in this review was introduced by Ronaldson-Bouchard and coworkers that developed a multi-organ-on-a-chip (see ) to more accurately model human physiology’s complexity [Citation44], particularly addressing the challenge of functionally integrating different hiPSC-derived tissues while preserving their specific architectures and tissue-mimetic functions of heart, bone, liver, and skin tissue environment. The authors introduce a vascular barrier to investigate the impact of mixed media cultures – a common solution to the shared media problem in multi-organ systems – to prove that endothelialization allows tissue-specific individual maturation for 4–6 weeks. The chip’s effectiveness in maintaining mature tissue states was compared to the mixed-media chip without an endothelial barrier, thus representing common medium culture, as well as isolated cultures (tissues cultured individually with and without endothelial barriers). The utility of an InterOrgan tissue chip system for drug screening was demonstrated through studies on the multi-organ toxicity of doxorubicin, a very well-investigated liver-metabolizing drug compound model. Notably, the authors were able to detect early miRNA biomarkers of doxorubicin cardiomyopathy, consistent with recent clinical studies suggesting that vascular flow linkage provides a more physiological context than common mixed medium culture. Additionally, miRNA expression in vascular flow was assessed to mimic clinical serum measurements, with the InterOrgan perfusate showing higher consistency with clinical biomarkers compared to the Mixed model. In summary, the InterOrgan tissue chip demonstrates the importance of inter-organ communication, endothelial-mediated partitioning, and metabolic processing in treatment responses to doxorubicin. This platform shows significant promise for accurately modeling human physiology and improving drug development processes.
Figure 4. (a) Design of a multi-organ platform with shared fluid dynamics. (ai) final assembled platform. (aii) top and bottom views of the platform. (aiii) Schematic for modular connections of various multi-organ platforms. (aiv) alternative design for mono- and bi-organ cultures. (av) Computational simulation of shear stress on the system. (b) Cardiac tissues after maturation, (bi) α-actin alignment. (bii) Capture rate (biii) Excitation threshold. (biv) force response depending on calcium concentration. (c) Liver tissues after maturation (ci) Immunohistochemical staining images, (cii) Albumin secretion. (di) Immunohistochemical staining of engineered bone slices. (dii) Bone density over time. (ei) Immunohistochemical staining of engineered skin slices. (eii) Skin barrier function measured via TEER. [Adapted with permission from [Citation38]].
![Figure 4. (a) Design of a multi-organ platform with shared fluid dynamics. (ai) final assembled platform. (aii) top and bottom views of the platform. (aiii) Schematic for modular connections of various multi-organ platforms. (aiv) alternative design for mono- and bi-organ cultures. (av) Computational simulation of shear stress on the system. (b) Cardiac tissues after maturation, (bi) α-actin alignment. (bii) Capture rate (biii) Excitation threshold. (biv) force response depending on calcium concentration. (c) Liver tissues after maturation (ci) Immunohistochemical staining images, (cii) Albumin secretion. (di) Immunohistochemical staining of engineered bone slices. (dii) Bone density over time. (ei) Immunohistochemical staining of engineered skin slices. (eii) Skin barrier function measured via TEER. [Adapted with permission from [Citation38]].](/cms/asset/7d773708-1ef0-4e00-8eda-e340b21f52e8/iemt_a_2362183_f0004_oc.jpg)
To briefly conclude this recent update section, the post-pandemic evolution in multi-organ-on-a-chip and/or body-on-a-chip systems over the last three years still focused on toxicological aspects of tissue–tissue crosstalk. Hopefully, these advances will also find applications in pharmacodynamics and pharmacokinetic studies as pioneered by the Shuler group with their multi-cellular PDPK modeling approaches in the near future [Citation32,Citation45,Citation46].
3. Expert opinion
3.1. Bias towards lung-oriented toxicology is caused by the emergence of COVID-19 disease
With the rise of SARS-CoV-2, the landscape of fundamental research on individual organ models drastically changed (see also ). This can be clearly seen by the rise of lung-on-chip models during and after this period [Citation26–28,Citation28,Citation47]. During this period, the focus was laid on the toxicological impact of a SARS-CoV-2 infection, and the methods used were shaped mainly by those commonly available, ranging from easy-to-use-cell viability staining to standard cytometry and omics approaches. As a direct consequence of these trends, the obviously smaller body-on-chip community was continuing toxicological screening applications, as the level of complexity potentially did not provide fast enough insights into tissue–tissue interactions relating to the study SARS-CoV-2 itself as well as drug candidates.
Even though the earliest studies on multi-tissue-chip culture were born from the idea of modeling absorption and biodistribution throughout multiple organ compartments. For a variety of reasons, the field moved over the last two decades toward toxicological screening with three key aspects we want to discuss in more detail. Firstly, for proper pharmacological and pharmacokinetic studies, the requirement to spatially identify compounds on a chip demands a very limiting set of analytical techniques that often show poor compatibility with silicone-based chips (i.e. solvent extraction methods). Spatial mass spectroscopy (MS), conventional MS, and HPLC are mostly incompatible with the miniaturization approach of microfluidics and the related sample volumes [Citation48]. Moreover, this equipment needs highly laborious methods and protocol optimizations for each analyte, being significantly more resource-heavy compared to easily available microscopical live/dead staining approaches currently in use for body-on-a-chip applications in the toxicology field. Secondly, establishing body-on-a-chip models is labor-intensive and requires highly trained staff, which was evidently also not possible during the pandemic due to legal restrictions. Notably, science fields relying heavily on laboratory wet lab work were affected more than other research fields [Citation49]. The third reason we believe explains the slower progress on multi-organ models over other chip fields is based on research incentives given by funding, publishing and regulatory bodies. After 2020, the funding and publishing threshold on projects relating to the SARS-Cov-2 pandemic was lower, for instance, for lung models compared to body and multi-tissue-on-a-chip systems [Citation50,Citation51]. Considering the drug approval process, first the efficacy of a drug is tested using in vitro studies prior to any toxicological study. With FDAs gaining confidence in supporting the use of microsystems in the drug approval process, the development of models for both tox and efficacy testing gained more momentum recently [Citation52]. However, this development gives incentive to the community to focus more on toxicological studies because of the evident easier conduct.
In summary, the circumstances coming from the SARS-CoV-2 crisis, resource availability, and the related changes in research incentives nurtured the development of toxicology-focused organ-on-a-chip systems. This, in turn, decreases the amount of pharmacological body-on-a-chip studies performed, which might give various new insights on tissue interplay based on the equipment used.
3.2. Translation from body-on-a-chip to human body responses
3.2.1. Supplementation of culture media for multiple organs
Fabricating a body-on-a-chip system is a complex technological endeavor that merges intricate engineering with advanced biological and tissue engineering knowledge [Citation13]. This quest for better emulation of human physiology is hampered still by the availability of novel and accessible fabrication technologies in the academic sector. Progress in additive manufacturing and bioprinting, as demonstrated within the highlighted studies found in this article, has significantly contributed to the successful acceleration of highly complex prototypes aiming at Body-on-a-Chip approaches [Citation37,Citation53]. The main engineering challenge, as outlined in , may still lie in 2024 onwards in accurately replicating the diverse and interconnected systems of the human body on a micro-scale while also considering the biological niche and the physiological response of whole organisms. From a biological perspective, a frequent bottleneck can be found in the fact that when developing single-organ cultures, all parameters, such as nutrients and oxygen levels and compositions, can be easily optimized. As more organ models are integrated into a single chip-based system, growth conditions may not be optimal for a collective of different tissue models. A common condition that interferes between organs is media composition, which leads to time-consuming media benchmarking experiments and results in compromising the health of every organoid simultaneously. Common cell culture media contains high amounts of poorly described serum (5–10%) [Citation54] and glucose [Citation55] that maintain cells in a constant growth state by providing high amounts of growth factors and a constant energy source. In humans, growth factors are only expressed when needed [Citation56], and common glucose levels in the blood range from 3.9 to 5.6 mmol/L [Citation57,Citation58]. To better translate findings from body-on-a-chip platforms into humans, it is imperative to further advance the field of serum-free low glucose culture [Citation42,Citation54].
3.2.2. Characterization of iPSCs differentiation as another bottleneck
Utilizing human-derived cells in disease models addresses the issue of translational accuracy. This ensures that the drug response observed in the model is more representative of potential human reactions, reducing the uncertainty associated with animal models [Citation3,Citation59]. Body-on-a-chip approaches allow for the exploration of disease-specific models, such as cancer or malaria, alongside standard drug screening [Citation42,Citation60]. This creates opportunities for a more thorough evaluation of drug efficacy and safety across a range of diseases with tissue-specific read-outs unprecedented for non-chip-related in vitro technologies such as spheroids, organoids, and pellet cultures. Incorporating induced pluripotent stem cells (iPSCs) into the body-on-a-chip framework enables for the first time personalized drug screening similar to what has proven useful for organoid approaches [Citation61,Citation62]. This is particularly significant for conditions like cancer or sex-specific drug responses, where treatment can be customized based on the unique cellular profile of each individual patient. Since the discovery of the Yamanaka factors to induce somatic cells into pluripotency [Citation63], technology in the area has advanced rapidly. The creation of iPSCs has become routinary, and its methodology has been greatly expanded and improved [Citation64]. Access to pluripotent cells nowadays seems only hindered by its price tag. Working with iPSCs implies high costs coming from specialized media, precise factors or growth matrices needed for re-differentiation of the cells into the required lineage [Citation65]. Furthermore, after weeks and months of complicated differentiations, the cells must be characterized and tested to confirm their full phenotype which involves expensive machinery and methodologies such as mass-spectroscopy and fluorescence-activated cell sorting [Citation66].
3.3. Multi-organs-on-a-chip may benefit from lab-on-a-chip principles of automation
3.3.1. Sensor integration
The most common biosensors employed in organ systems are electrochemical and optical types, such as those measuring barrier integrity, neuronal activity, oxygen consumption, cytokine release, and contractility [Citation35,Citation67–69]. However, it is noteworthy that a broader range of bio- and microsensors exists, yet to be fully integrated into these platforms; however, the applied science of integration is often less rewarding than pushing the frontiers of sensing with novel chemistries and read-out principles. The future of multi-organ-on-a-chip research also hinges on developing strategies to effectively monitor key organ functions, particularly in multi-organ and body-on-a-chip systems that are directed more toward the fields of toxico- and pharmacokinetics. Each tissue and organ type may require a specific biosensing strategy, tissue-specific read-out parameters, anti-biofouling strategies and specificity toward target molecules, further complicating the development of these intricate systems [Citation70]. The variety of bio- and microsensors available is vast, with new, promising sensor types being continually developed [Citation71]. Noteworthy is among the observable discrepancies in what ‘long-term’ means in biosensing compared to organ bioengineering, the challenge to motivate electrical engineers and biologists to embrace the high interdisciplinarity of sensor-integrated organs- and body-on-a-chip systems. Moving beyond the established, simpler methodologies will be essential for making in vitro human physiology modeling more accurate, reliable, and scalable, leveraging multi-modal sensing capabilities. As the community advances, the integration of diverse biosensors into organ-on-a-chip models will significantly improve the drug development process by enhancing our understanding of how close human in vitro physiology is with respect to the actual human tissue and body response.
3.3.2. Fluidic control for high throughput applications may again be enabled by lab-on-a-chip principles
In line with these principles, the further integration of other lab-on-a-chip technologies, including pneumatic actuators, degassers, and even micropumps, will allow for more precise control over this highly complex multi-organ microenvironment. Though extensively studied, in high throughput applications microfluidic control has not yet met its full potential [Citation72]. This technological barrier, in turn, led to the case that many systems still rely on the use of either hydrostatic flow, syringe pumps, or peristaltic pumps to drive a more dynamic cultivation routine, which all have limitations when considering the physiology of the human cardiovascular system. Further functions integration can significantly reduce dead volumes as well as the real total volume of the medium used when adding up the dead volumes of the platform periphery (i.e. meters of peristaltic tubes or 50 mL conical tubes as medium reservoirs for easier fluid management). Even though this may not seem a big obstacle, reducing dead volumes can speed up the reactivity and responsiveness of multi-organ models as being demonstrated for miniaturized analysis systems and cell-based microfluidics alike [Citation73].
3.3.3. Future trajectory and potential
The exploration of pharmacodynamics (PD), pharmacokinetics (PK), and pharmacokinetic-pharmacodynamic (PKPD) relationships using multi-organ chips (MOC) represents a frontier in pharmacological research. Despite their potential to revolutionize drug discovery and development, these aspects are not frequently studied within multi-organ platforms due to various challenges including system complexity, read-out challenges, scalability and throughput, as well as standardization and validation. Addressing these challenges will be crucial for harnessing the full potential of MOC systems in pharmacological research. Advancements in tissue engineering and (induced pluripotent) stem cell technology can lead to more sophisticated models that accurately mimic the physiological interactions between different organ systems. Utilizing induced pluripotent stem cells (iPSCs) could allow for patient-specific models, providing deeper insights into individual variations in drug metabolism and response. Incorporating real-time, noninvasive sensors within MOC systems can facilitate continuous monitoring of drug concentration, metabolic products, and biomarker release. The development of miniaturized and analytically more relevant biosensors compatible with the microenvironment of a chip is essential for detailed PK and PD analysis. Developing modular chip designs will enable scalable production and parallel testing of multiple drug candidates. Automation and microfluidics integration can further enhance throughput, making MOC platforms more viable for comprehensive PKPD studies. Establishing standardized protocols for the fabrication, operation, and analysis of chip systems by animal-free and synthetic media will improve reproducibility and comparability of results. Collaborative efforts between academic institutions, regulatory bodies, and the pharmaceutical industry are necessary to validate MOC models against established in vivo and in vitro data. Fostering collaborations between engineers, biologists, pharmacologists, and data scientists can accelerate the development of chip platforms that are both physiologically relevant and technically advanced. Sharing knowledge and methodologies across disciplines will drive innovation in MOC design and application. Engaging with regulatory agencies early in the development process will ensure that platform technologies meet the necessary criteria for use in drug testing and approval processes. Regulatory guidance can also incentivize further research and development in PD, PK, and PKPD studies using MOCs. Lastly, incorporating technologies into study curriculum, workshops and trainings of students enrolled in pharmaceutical sciences and related fields as well as professionals will update and prepare the current next generation of researchers to effectively utilize these platforms as disruptive technologies in pharmaceutical sciences similar to the compelling progress happening in the toxicological fields to date.
4. Conclusion
From the reviewed literature, it is evident that recent technological and biological advancements are enabling more complex multi-organ models which better mimic human physiological responses. The integration of various organs such as the liver, heart, kidney, and pancreas into a single microfluidic platform has led to enhanced tissue-specific toxicities and improved drug safety assessments and was influenced by the necessity to develop lung models to investigate virus infectious diseases.
However, the field faces challenges that need to be addressed to fulfill the potential of these systems fully. As we move forward, it is crucial that the research community addresses these challenges through continued innovation, collaboration, and a balanced approach to both toxicological and pharmacokinetic studies. The ultimate goal is to create highly accurate and representative human body-on-a-chip systems that can significantly reduce the reliance on animal models and streamline the drug development process, leading to safer and more effective treatments for human diseases.
Article highlights
Multi-organ models gain complexity and start to better resemble tissue-specific toxicities.
Biological advances are driven by better tissue-mimetics.
A revitalized focus toward lung-oriented toxicology studies based on urgent requirements arising from the SARS-CoV-2 pandemic.
Technological advances aim at better recapitulation of physiological fluid dynamic behavior in multi-tissue chips using also defined media compositions to manage incompatibilities arising from multi-cell coculture approaches.
Declaration of interest
The authors have no relevant affiliations or financial involvement with any organization or entity with a financial interest in or financial conflict with the subject matter or materials discussed in the manuscript. This includes employment, consultancies, honoraria, stock ownership or options, expert testimony, grants or patents received or pending, or royalties.
Reviewer disclosures
Peer reviewers on this manuscript have no relevant financial or other relationships to disclose.
Acknowledgments
The authors acknowledge TU Wien Bibliothek for financial support through its Open Access Funding Programme.
Additional information
Funding
References
- Ingber DE. Is it time for reviewer 3 to request human organ chip experiments instead of animal validation studies? Adv Sci. 2020;7(22). doi: 10.1002/advs.202002030
- Leenaars CHC, Kouwenaar C, Stafleu FR, et al. Animal to human translation: a systematic scoping review of reported concordance rates. J Transl Med. 2019;17(1):223. doi: 10.1186/s12967-019-1976-2
- McGonigle P, Ruggeri B. Animal models of human disease: challenges in enabling translation. Biochem Pharmacol. 2014;87(1):162–171. doi: 10.1016/j.bcp.2013.08.006
- Jensen C, Teng Y. Is it time to start transitioning from 2D to 3D cell culture? Front Mol Biosci. 2020;7. doi: 10.3389/fmolb.2020.00033
- Wang T, Wang X, Zhuang Y, et al. A systematic evaluation of quenching and extraction procedures for quantitative metabolome profiling of HeLa carcinoma cell under 2D and 3D cell culture conditions. Biotechnol J. 2023;18(5):18. doi: 10.1002/biot.202200444
- Fontoura JC, Viezzer C, dos Santos FG, et al. Comparison of 2D and 3D cell culture models for cell growth, gene expression and drug resistance. Mater Sci Eng C. 2020;107:110264. doi: 10.1016/j.msec.2019.110264
- Ponmozhi J, Dhinakaran S, Varga-Medveczky Z, et al. Development of skin-on-A-Chip platforms for different utilizations: factors to Be considered. Micromachines (Basel). 2021;12(3):294. doi: 10.3390/mi12030294
- Ponmozhi J, Dhinakaran S, Kocsis D, et al. Models for barrier understanding in health and disease in lab-on-a-chips. Tissue Barr. 2023;12(2). doi: 10.1080/21688370.2023.2221632
- Zhou D, Chen L, Ding J, et al. A 3D engineered scaffold for hematopoietic progenitor/stem cell co-culture in vitro. Sci Rep. 2020;10(1):11485. doi: 10.1038/s41598-020-68250-5
- Vis MAM, Ito K, Hofmann S. Impact of culture medium on cellular interactions in in vitro co-culture systems. Front Bioeng Biotechnol. 2020:8. doi: 10.3389/fbioe.2020.00911
- Paschos NK, Brown WE, Eswaramoorthy R, et al. Advances in tissue engineering through stem cell-based co-culture. J Tissue Eng Regen Med. 2015;9:488–503. doi: 10.1002/term.1870
- Ghanem A, Shuler ML. Characterization of a perfusion reactor utilizing mammalian cells on microcarrier beads. Biotechnol Prog. 2000;16(3):471–479. doi: 10.1021/bp000047o
- Driver R, Mishra S. Organ-on-a-chip technology: an in-depth review of recent advancements and future of whole body-on-chip. BioChip J. 2023;17:1–23. doi: 10.1007/s13206-022-00087-8
- Dehne E-M, Marx U. Human body-on-a-chip systems. Organ-on-a-chip. Elsevier; 2020. p. 429–439. doi: 10.1016/B978-0-12-817202-5.00013-9
- Xia Y, Whitesides GM. Soft lithography. Ann Rev Mater Sci. 1998;28:153–184. doi: 10.1146/annurev.matsci.28.1.153
- Battat S, Weitz DA, Whitesides GM. An outlook on microfluidics: the promise and the challenge. Lab Chip. 2022;22:530–536. doi: 10.1039/D1LC00731A
- Zhang Y, Duan H, Li G, et al. Construction of liquid metal-based soft microfluidic sensors via soft lithography. J Nanobiotechnology. 2022;20:246. doi: 10.1186/s12951-022-01471-0
- Ferrari E, Nebuloni F, Rasponi M, et al. Photo and soft lithography for organ-on-chip applications. Organ-on-a-Chip: Methods and Protocols. 2022:1–19. doi: 10.1007/978-1-0716-1693-2_1
- Huh D, Matthews BD, Mammoto A, et al. Reconstituting organ-level lung functions on a chip. Sci (1979). 2010;328:1662–1668. doi: 10.1126/science.1188302
- Skardal A, Aleman J, Forsythe S, et al. Drug compound screening in single and integrated multi-organoid body-on-a-chip systems. Biofabrication. 2020;12(2):025017. doi: 10.1088/1758-5090/ab6d36
- Jia X, Yang X, Luo G, et al. Recent progress of microfluidic technology for pharmaceutical analysis. J Pharm Biomed Anal. 2022;209:114534. doi: 10.1016/j.jpba.2021.114534
- Azizipour N, Avazpour R, Rosenzweig DH, et al. Evolution of biochip technology: a review from lab-on-a-chip to organ-on-a-chip. Micromachines (Basel). 2020;11:599. doi: 10.3390/mi11060599
- O’Neill AT, Monteiro-Riviere NA, Walker GM. Characterization of microfluidic human epidermal keratinocyte culture. Cytotechnology. 2008;56:197–207. doi: 10.1007/s10616-008-9149-9
- Chang R, Nam J, Sun W. Direct cell writing of 3D microorgan for in vitro pharmacokinetic model. Tissue Eng Part C Methods. 2008;14:157–166. doi: 10.1089/ten.tec.2007.0392
- Kimura H, Yamamoto T, Sakai H, et al. An integrated microfluidic system for long-term perfusion culture and on-line monitoring of intestinal tissue models. Lab Chip. 2008;8:741. doi: 10.1039/b717091b
- Jain A, Barrile R, van der Meer A, et al. Primary human lung alveolus‐on‐a‐chip model of intravascular thrombosis for assessment of therapeutics. Clin Pharmacol Ther. 2018;103:332–340. doi: 10.1002/cpt.742
- Huang D, Liu T, Liao J, et al. Reversed-engineered human alveolar lung-on-a-chip model. Proc Natl Acad Sci, USA. 2021;118(19):118. doi: 10.1073/pnas.2016146118
- Faley SL, Boghdeh NA, Schaffer DK, et al. Gravity-perfused airway-on-a-chip optimized for quantitative BSL-3 studies of SARS-CoV-2 infection: barrier permeability, cytokine production, immunohistochemistry, and viral load assays. Lab Chip. 2024;24(6):1794–1807. doi: 10.1039/D3LC00894K
- Lai BFL, Huyer LD, Lu RXZ, et al. InVADE: integrated vasculature for assessing dynamic events. Adv Funct Mater. 2017;27(46):27. doi: 10.1002/adfm.201703524
- Oleaga C, Riu A, Rothemund S, et al. Investigation of the effect of hepatic metabolism on off-target cardiotoxicity in a multi-organ human-on-a-chip system. Biomaterials. 2018;182:176–190. doi: 10.1016/j.biomaterials.2018.07.062
- Skardal A, Murphy SV, Devarasetty M, et al. Multi-tissue interactions in an integrated three-tissue organ-on-a-chip platform. Sci Rep [Internet]. 2017 [cited 2023 Nov 13];7(1):1–16. Available from: https://www.nature.com/articles/s41598-017-08879-x
- Oleaga C, Bernabini C, Smith AS, et al. Multi-organ toxicity demonstration in a functional human in vitro system composed of four organs. Sci Rep [Internet]. 2016 [cited 2023 Nov 13];6(1):1–17. Available from: https://www.nature.com/articles/srep20030
- Novak R, Ingram M, Marquez S, et al. Robotic fluidic coupling and interrogation of multiple vascularized organ chips. Nat Biomed Eng. 2020;4:407–420. doi: 10.1038/s41551-019-0497-x
- Jang K-J, Otieno MA, Ronxhi J, et al. Reproducing human and cross-species drug toxicities using a liver-chip. Sci Transl Med. 2019;11(517):11. doi: 10.1126/scitranslmed.aax5516
- Rothbauer M, Ertl P. Emerging biosensor trends in organ-on-a-chip. In: Bahnemann J Grünberger A, editors. Microfluidics in biotechnology. Cham: Springer; 2020. p. 343–354.
- Rothbauer M, Rosser JM, Zirath H, et al. Tomorrow today: organ-on-a-chip advances towards clinically relevant pharmaceutical and medical in vitro models. Curr Opin Biotechnol. 2019;55:81–86. doi: 10.1016/j.copbio.2018.08.009
- Rothbauer M, Eilenberger C, Spitz S, et al. Recent advances in additive manufacturing and 3D bioprinting for organs-on-a-chip and microphysiological systems. Front Bioeng Biotechnol [Internet]. 2022 [cited 2023 Apr 3];10. Available from:/pmc/articles/PMC8891807/
- Adam Kratz SR, Höll G, Schuller P, et al. Latest trends in biosensing for microphysiological organs-on-a-chip and body-on-a-chip systems. Biosensors (Basel) [Internet]. 2019 [cited 2023 Apr 3];9:110. Available from: https://www.mdpi.com/2079-6374/9/3/110/htm
- Vunjak-Novakovic G, Ronaldson-Bouchard K, Radisic M. Organs-on-a-chip models for biological research. Cell. 2021;184:4597–4611. doi: 10.1016/j.cell.2021.08.005
- Yin F, Zhang X, Wang L, et al. HiPSC-derived multi-organoids-on-chip system for safety assessment of antidepressant drugs. Lab Chip [Internet]. 2021 [cited 2023 Nov 13];21:571–581. Available from: https://pubs.rsc.org/en/content/articlehtml/2021/lc/d0lc00921k
- Zandi Shafagh R, Youhanna S, Keulen J, et al. Bioengineered pancreas–liver crosstalk in a microfluidic coculture chip identifies human metabolic response signatures in prediabetic hyperglycemia. Adv Sci. 2022;9. doi: 10.1002/advs.202203368
- Rupar MJ, Sasserath T, Smith E, et al. Development of a human malaria-on-a-chip disease model for drug efficacy and off-target toxicity evaluation. Sci Rep [Internet]. 2023 [cited 2023 Nov 15];13(1):1–14. Available from: https://www.nature.com/articles/s41598-023-35694-4
- Rajan SAP, Aleman J, Wan M, et al. Probing prodrug metabolism and reciprocal toxicity with an integrated and humanized multi-tissue organ-on-a-chip platform. Acta Biomaterialia. 2020;106:124–135. doi: 10.1016/j.actbio.2020.02.015
- Ronaldson-Bouchard K, Teles D, Yeager K, et al. A multi-organ chip with matured tissue niches linked by vascular flow. Nat Biomed Eng. 2022;6:351–371. doi: 10.1038/s41551-022-00882-6
- Sung JH, Esch MB, Shuler ML. Integration of in silico and in vitro platforms for pharmacokinetic–pharmacodynamic modeling. Expert Opin Drug Metab Toxicol. 2010;6:1063–1081. doi: 10.1517/17425255.2010.496251
- McAleer CW, Long CJ, Elbrecht D, et al. Multi-organ system for the evaluation of efficacy and off-target toxicity of anticancer therapeutics. Sci Transl Med [Internet]. 2019 [cited 2023 Nov 15];11. Available from: https://www.science.org
- Si L, Bai H, Rodas M, et al. A human-airway-on-a-chip for the rapid identification of candidate antiviral therapeutics and prophylactics. Nat Biomed Eng. 2021;5:815–829. doi: 10.1038/s41551-021-00718-9
- Spandana T, Beeraka NM, Hemanth Vikram PR, et al. Technical advances in the chiral separation of anti-diabetic drugs using analytical and bio-analytical methods: a comprehensive review. Curr Anal Chem. 2022;18:1057–1069. doi: 10.2174/1573411018666220820101237
- Myers KR, Tham WY, Yin Y, et al. Unequal effects of the COVID-19 pandemic on scientists. Nat Human Behav [Internet]. 2020 [cited 2024 Apr 19];4(9):880–883. Available from: https://www.nature.com/articles/s41562-020-0921-y
- Shan J, Ballard D, Vinson DR. Publication non grata: the challenge of publishing non-COVID-19 research in the COVID era. Cureus [Internet]. 2020 [cited 2024 Apr 19];12. Available from: https://pubmed.ncbi.nlm.nih.gov/33312801/
- Riccaboni M, Verginer L, Naudet F. The impact of the COVID-19 pandemic on scientific research in the life sciences. PLOS ONE [Internet]. 2022 [cited 2024 Apr 19];17(2):e0263001. Available from: https://journals.plos.org/plosone/article?id=10.1371/journal.pone.0263001
- Natu R, Herbertson L, Sena G, et al. A systematic analysis of recent technology trends of microfluidic medical devices in the United States. Micromachines (Basel). 2023;14:1293. doi: 10.3390/mi14071293
- Nothdurfter D, Ploner C, Coraça-Huber DC, et al. 3D bioprinted, vascularized neuroblastoma tumor environment in fluidic chip devices for precision medicine drug testing. Biofabrication. 2022;14:035002. doi: 10.1088/1758-5090/ac5fb7
- Ghasemi N, Bandehpour M, Ranjbari J. Optimization of key factors in serum free medium for production of human recombinant GM-CSF using response surface methodology. Iran J Pharm Res. 2019;18:146–156. doi: 10.22037/ijpr.2020.112322.13681
- Aldoss A, Lambarte R, Alsalleeh F. High-glucose media reduced the viability and induced differential pro-inflammatory cytokines in human periodontal ligament fibroblasts. Biomolecules. 2023;13:690. doi: 10.3390/biom13040690
- Werner S, Grose R. Regulation of wound healing by growth factors and cytokines. Physiol Rev. 2003;83:835–870. doi: 10.1152/physrev.2003.83.3.835
- Güemes M, Rahman SA, Hussain K. What is a normal blood glucose? Arch Dis Child. 2016;101:569–574. doi: 10.1136/archdischild-2015-308336
- Iyer VV, Yang H, Ierapetritou MG, et al. Effects of glucose and insulin on HepG2‐C3A cell metabolism. Biotechnol Bioeng. 2010;107(2):347–356. doi: 10.1002/bit.22799
- Heinonen T. Better science with human cell-based organ and tissue models. Altern Lab Animals. 2015;43(1):29–38. doi: 10.1177/026119291504300107
- Zhai J, Li C, Li H, et al. Cancer drug screening with an on-chip multi-drug dispenser in digital microfluidics. Lab Chip [Internet]. 2021 [cited 2023 Nov 20];21:4749–4759. Available from: https://pubs.rsc.org/en/content/articlehtml/2021/lc/d1lc00895a
- Nicholson MW, Ting C-Y, Chan DZH, et al. Utility of iPSC-derived cells for disease modeling, drug development, and cell therapy. Cells. 2022;11:1853. doi: 10.3390/cells11111853
- Park S, Gwon Y, Khan SA, et al. Engineering considerations of iPSC-based personalized medicine. Biomater Res. 2023;27:67. doi: 10.1186/s40824-023-00382-x
- Okita K, Ichisaka T, Yamanaka S. Generation of germline-competent induced pluripotent stem cells. Nature. 2007;448:313–317. doi: 10.1038/nature05934
- Wang H, Yang Y, Liu J, et al. Direct cell reprogramming: approaches, mechanisms and progress. Nat Rev Mol Cell Biol. 2021;22:410–424. doi: 10.1038/s41580-021-00335-z
- Suzuka J, Tsuda M, Wang L, et al. Rapid reprogramming of tumour cells into cancer stem cells on double-network hydrogels. Nat Biomed Eng. 2021;5:914–925. doi: 10.1038/s41551-021-00692-2
- O’Shea O, Steeg R, Chapman C, et al. Development and implementation of large-scale quality control for the European bank for induced pluripotent stem cells. Stem Cell Res. 2020;45:101773. doi: 10.1016/j.scr.2020.101773
- Vala M, Robelek R, Bocková M, et al. Real-time label-free monitoring of the cellular response to osmotic stress using conventional and long-range surface plasmons. Biosens Bioelectron. 2013;40:417–421. doi: 10.1016/j.bios.2012.07.020
- Modena MM, Chawla K, Misun PM, et al. Smart cell culture systems: integration of sensors and actuators into microphysiological systems. ACS Chem Biol. 2018;13:1767–1784. doi: 10.1021/acschembio.7b01029
- Ungerböck B, Charwat V, Ertl P, et al. Microfluidic oxygen imaging using integrated optical sensor layers and a color camera. Lab Chip. 2013;13:1593. doi: 10.1039/c3lc41315b
- Szunerits S, Pagneux Q, M’Barek YB, et al. Do not let electrode fouling be the enemy of bioanalysis. Bioelectrochemistry. 2023;153:108479. doi: 10.1016/j.bioelechem.2023.108479
- Esmaeilzadeh AA, Yaseen MM, Khudaynazarov U, et al. Recent advances on the electrochemical and optical biosensing strategies for monitoring microRNA-21: a review. Anal Methods. 2022;14:4449–4459. doi: 10.1039/D2AY01384C
- Iakovlev AP, Erofeev AS, Gorelkin PV. Novel pumping methods for microfluidic devices: a comprehensive review. Biosensors (Basel). 2022;12:956. doi: 10.3390/bios12110956
- Rothbauer M, Charwat V, Bachmann B, et al. Monitoring transient cell-to-cell interactions in a multi-layered and multi-functional allergy-on-a-chip system. Lab Chip. 2019;19:1916–1921. doi: 10.1039/C9LC00108E