Abstract
The effects of salt stress on the contents of organic solutes and on the pattern of free amino acids were studied in leaves and roots of two maize genotypes, BR5033 (salt-tolerant) and BR5011 (salt-sensitive). In leaves and roots of salt-stressed plants, soluble amino-N increased with time when compared to the controls. Salt stress increased the soluble protein content only in leaves of BR5011. Salinity increased the content of the majority of the free amino acids in leaves and roots of genotypes studied. Results suggest the hypothesis of disturbances in translocation of N-containing compounds from shoot to root in the salt-sensitive genotype. Results also suggest that the accumulation of organic solutes, mainly in roots of BR5033, may have an important role in the tolerance of this genotype to salt stress.
Introduction
Plant salt tolerance is a complex phenomenon involving alterations in physiological and biochemical processes, which may result in morphological and developmental changes (Delauney and Verma Citation1993; Hare and Cress Citation1997). Although salt tolerance mechanisms are still not well understood (Hasegawa et al. Citation2000), ion absorption and the synthesis and accumulation of low molecular weight organic compounds present in the cytoplasm, and known as compatible solutes, have been considered as an important mechanism for osmotic adjustment in plants (Strange Citation2004). In addition to their role in osmotic adjustment, a number of other roles for these compounds have been hypothesized: lowering of cytoplasm Ψs for the maintenance of internal cell osmotic equilibrium; helping the ionic homeostasis between cells and external environment; contributing as an available source of carbon and nitrogen during limited growth and photosynthesis; and contributing to stabilization of proteins, protein complexes and membranes (Bohnert and Shen Citation1999; Bray et al. Citation2000). Additionally, they may also help controlling the pH in the cytosol and the detoxification of NH4 + excess during periods of stress (Gilbert et al. Citation1998; Mansour Citation2000). Current models suggest that small amounts of compatible solutes may also remove free radicals generated by secondary oxidative stress (Smirnoff and Cumbes Citation1989; Zhu Citation2002).
Nitrogen-containing compounds and polyhydroxylic compounds are among the most commonly compatible solutes accumulated in plants under stress conditions (Rabe Citation1990; Ashraf and Harris Citation2004). In maize, amino acid and carbohydrate accumulation has been studied in plants grown under water (Thakur and Rai Citation1982; Ranieri et al. Citation1989) and salt stress (Rodríguez et al. Citation1997; Azevedo Neto et al. Citation2004). Among the N-containing compounds, Pro accumulation has been widely studied in plant stress response. In addition, most of these studies were performed in photosynthetically active tissues and there is scarce information available for the root system, which is usually the first organ directly exposed to salt stress.
In higher plants, carbon and nitrogen metabolism are co-regulated (Ferrario-Méry et al. Citation1998). The interaction C to N involves reciprocal regulation between the pathways of C and N assimilation (Champigny and Foyer Citation1992). Consequently, the ability of higher plants to divert carbohydrates and nitrogenous compounds from precursors involved in biomass production toward those involved in solute accumulation which allow osmotic adjustment and turgor maintenance is important for understanding the responses of higher plants to salt stress (Binzel et al. Citation1985; Richardson and McCree Citation1985).
Therefore, the objective of this work was to evaluate the effects of salt stress on the contents of soluble amino-nitrogen, and soluble proteins, as well as on the pattern of leaf and root free amino acids of two maize genotypes differing in salt tolerance, expecting to contribute to the understanding of the physiological and biochemical mechanisms of salt tolerance.
Materials and methods
Plant material, growth and treatment conditions
Seeds of salt-tolerant (BR-5033) and a salt-sensitive (BR-5011) maize genotypes (Azevedo Neto et al. Citation2004), were sown in trays containing vermiculite and irrigated daily with distilled water. Four-day-old seedlings were transferred to trays containing aerated half-strength Hoagland nutrient solution. Nine days later, they were transferred to 3-l plastic pots containing aerated full-strength nutrient solution in the absence (control) or presence of 100 mmol l−1 NaCl (salt stress treatment). Salt treatment began on transplantation into plastic pots, and was obtained by adding NaCl to the nutrient solution at increments of 25 mmol l−1 every 24 h until reaching 100 mmol l−1 as final concentration. Plants were harvested one day before and 5, 10, 15, 20, and 25 days after salt started to be added, when leaves and roots were cut, frozen in liquid nitrogen, lyophilized, ground to a powder and kept in a freezer (−25°C) for further analyses. The experiment was carried out under glasshouse conditions. The mean temperature, air relative humidity and photosynthetic active radiation (measured at noon) were 27°C, 65% and 1200 µmol m−2 s−1, respectively.
Extract preparation
Lyophilized leaf (0.20 g) and root (0.15 g) powder were homogenized in a mortar and pestle with 4 ml of extraction buffer (100 mmol l−1 potassium phosphate buffer, pH 7.0, 0.1 mmol l−1 EDTA). The homogenate was filtered through muslin cloth and centrifuged at 16,000 g for 15 min. The supernatant fraction was used as crude extract for soluble proteins contents determinations. An aliquot (0.5 ml) of this supernatant was deproteinized with the same volume of a 100 g l−1 trichloroacetic acid solution and centrifuged at 12,000 g for 5 min. The supernatant fraction was used for soluble amino-N determination and amino acids partitioning.
Soluble amino-N and soluble proteins contents
Soluble amino-N was determined at 570 nm by the ninhydrin method (Yemm and Cocking Citation1955), using L-glycine as standard. Soluble proteins were determined at 595 nm by the protein-dye binding method (Bradford Citation1976), using bovine albumin as a standard. The experimental design was a completely randomized factorial, six (harvest periods)×two (genotypes)×two (salt levels) with five replicates. Each extract represented one replicate, which was assayed in duplicate. When F values of the ANOVA were statistically significant, the comparisons among treatments were made based on the means±standard deviation values.
Amino acids derivatization
Prior to derivatization, 250 µl of deproteinized crude extract was filtered through a 0.45 µm Millipore membrane filter. An aliquot (100 µl) of filtrate was dried in a freeze dryer, resuspended in 10 µl of methanol, triethylamine, and water (2:2:1 v/v) and dried again. The samples of amino acids were converted to their phenylthiocarbamyl derivates by addition of a 20 µl mixture of methanol, triethylamine, water, and phenylisothiocianate (PICT) (7:1:1:1 v/v). After drying, the samples were resuspended with 100 µl of Pico-Tag solvent and transferred to the injection vials.
Amino acids analysis
Amino acids were analyzed by HPLC, using the Waters Pico-Tag method. An aliquot (10 µl) of PICT-amino acids was injected in a Waters 088131 Pico-Tag column (3.5×250 mm) and eluted at a flow rate of 1 ml min−1 at 40°C as described in the Pico-Tag method (Cohen et al. Citation1989). The apparatus used was the Alliance HPLC System (Milford, MA, USA) consisting of the Waters 2690 separation module, a Waters 486 absorbance detector and Millennium 32 chromatography manager software. The PICT-amino acids were detected at 254 nm. Quantitative analysis was based on standard curves established prior to the analysis of samples and data expressed as µmol g−1 dry mass (DM).
Results
Soluble amino-N and soluble protein contents
The time courses of changes in soluble amino-N, and soluble proteins are given in . By day 10, salt stress increased the leaf amino-N content of the BR5033 genotype by nearly 38% in relation to the control plants and remained fairly constant thereafter (A). In leaves of BR5011, the values were 66% higher than in control plants on the 10th day from the start of the experiment, reached a maximum (227% of the control) on the 20th day and then decreased to 187% of the control by the end of the experimental period. In stressed roots, the soluble amino-N content of BR5033 was reduced by 24% of the control on the 5th day and increased thereafter, reaching 208% of the control at the end of experimental period (B). When the variations in root soluble amino-N contents of BR5011 were analyzed, an initial increase was observed in relation to the control by the 5th day. From this day on, to the end of the experimental period, it fluctuated slightly around a mean value of 44% higher than in control plants (B).
Figure 1. Time course of soluble amino-nitrogen (A and B) and soluble proteins (C and D) contents, in leaves and roots of salt-tolerant BR5033 (circles) and salt-sensitive BR5011 (triangles) maize genotypes grown under salt stress (100 mmol l-1 NaCl) conditions. Time represents days after the first harvest, which was made one day before the start of salt additions (day 0). Plants were subjected to 100 mmol l−1 NaCl for 21 days after completing the NaCl addition. Values indicate the mean±SD.
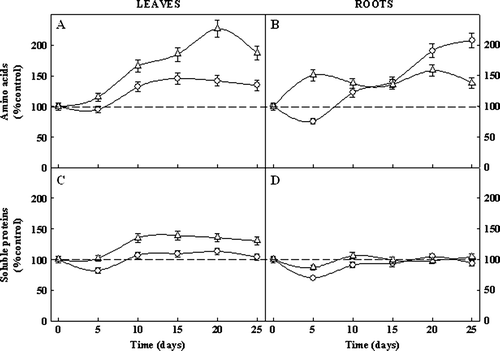
Salt stress did not induce substantial changes in soluble proteins content of the BR5033, except for a reduction of 18% in leaves (C) and 30% in roots (D) by the 5th day from the start of the experiment. Leaf soluble proteins content of BR5011 showed an increase of 35% of control on day 10 as a result of salt stress, and remained constant thereafter. However, when this genotype was subjected to salt stress, its root soluble proteins content was not affected by salinity (D).
Free amino acids
Salinity increased the content of the majority of the leaf () and root () free amino acids of both genotypes studied. Considering the average of the last two harvests (on days 20 and 25), salinity induced an average increase in most of the leaf free amino acids contents in relation to control plants. This increase was conspicuous for both salt-tolerant (BR5033) and salt-sensitive (BR5011) genotypes. The amino acids that showed the highest increases were alanine (Ala), serine (Ser), glycine (Gly), histidine (His), proline (Pro), aspartate (Asp), tyrosine (Tyr), and methionine (Met). During this same period, the largest decreases were observed for leucine (Leu) and isoleucine (Ile) ().
Table 1 Concentration (µmol g-1 dry mass) of free amino acids, in leaves of salt-tolerant (BR5033) and salt-sensitive (BR5011) maize genotypes grown under control (C) and salt stress (S) conditions. Values in parentheses are the percentage of control. Additional details are given in .
Table 2 Concentration (µmol g-1 dry mass) of free amino acids, in roots of salt-tolerant (BR5033) and salt-sensitive (BR5011) maize genotypes grown under control (C) and salt stress (S) conditions. Values in parentheses are the percentage of control. Additional details are given in .
Similar responses were observed for root free amino acids contents (). The largest increases in salt-tolerant and salt-sensitive genotypes were found for Ala, Ser, Gly, Arg, His, Leu, Asp, Tyr, Thr, and Val. In stressed roots of BR5033, only glutamate (Glu) presented a slight reduction, while in stressed roots of the BR5011 genotype, Glu and Ile were substantially reduced.
When the variations of Pro concentration along the experimental period were analyzed, it was observed that leaf Pro in the salt-tolerant genotype (BR5033) increased more in the saline treatment than in the control, especially after the 15th day from the start of the experiment. Its greatest increase in relation to the control occurred on the 20th day (60%), and then it decreased to 22% from that of the control by the end of the experimental period (). Leaf Pro concentration of the salt-sensitive genotype (BR5011) also increased more in the saline treatment than in the control, but this increase started sooner than in the salt-tolerant genotype. At the 5th day from the start of the experiment it was already 46% higher than in control plants, reaching its maximal difference on day 20 (157%), and finally decreasing to control level at the end of the experimental period ().
Root Pro content in BR5033 increased more in the stressed than in the control plants, especially after 10 days from the start of the experiment (). Therefore, Pro content in stressed roots was 81% higher than in control at the end of the experimental period. Although in BR5011 the root Pro content in the saline treatment was always higher than in the control, these differences were not significant, except on day 5, when Pro content in roots of the stressed plants was 121% higher than the control ().
Discussion
The salt-induced increase in soluble amino-N contents in leaves (A) and roots (B) of both genotypes suggests a role for these organic solutes in osmotic adjustment or in other mechanisms related to salt stress. The accumulation of free amino acids and other soluble N-containing compounds in plants grown under salt stress have been reported in several reviews (Rabe Citation1990; Mansour Citation2000).
The increase in soluble amino-N, especially in free amino acids content, is frequently related to protein degradation (Roy-Macauley et al. Citation1992) or inhibition of protein synthesis (Dhindsa and Cleland Citation1975). Considering that salt stress did not reduce the soluble proteins content in leaves and roots from both genotypes, the soluble amino-N accumulation, particularly in leaves of the sensitive genotype, could be the result of a reduced export of amino acids and amides (Tully et al. Citation1979). Alternatively, this increase may be a consequence of a blockage of oxidative respiration, causing a flux of organic acids to the pool of amino acids (Olmos and Hellin Citation1996).
The higher increase of soluble proteins in stressed leaves of the salt-sensitive genotype (C) are in agreement with Ashraf and O'Leary (Citation1999), who reported that the increase in the soluble protein as a result of salt stress was higher in the salt-sensitive spring wheat cultivar than in the salt-tolerant. It is well known that plants under stress may accumulate small molecular mass proteins that could be used as a source of storage nitrogen that could be mobilized after stress relief or removal (Singh et al. Citation1987). Additionally, these proteins could also have a role in osmotic adjustment (Mansour Citation2000; Ashraf and Harris Citation2004).
The free amino acids changes as a result of salt stress varied between genotypes and plant organs. Although salt stress had increased the free amino acids content in leaves of both genotypes, this increase was more conspicuous in salt-sensitive than in salt-tolerant genotype. These results support the hypothesis of disturbances in translocation of N-containing compounds from shoot to root in salt-sensitive genotype (Tully et al. Citation1979; Larsson Citation1992).
The strong increase of Gly and Ser contents in salt-stressed leaves (), mainly in salt-sensitive genotype, suggests an increase in the photorespiration rate (Di Martino et al. Citation2003), since both amino acids are strongly involved in the photorespiratory cycle (Miflin and Lea Citation1977). In C4 plants also, photorespiration and the synthesis of Gly and Ser are enhanced under low CO2 and high O2 conditions (Lawlor and Fock Citation1978; De Veau and Burris Citation1989; Dai et al. Citation1993). Salt-induced water stress with high light and temperatures may lead to such conditions within leaves due stomatal closure (Lawlor and Fock Citation1978; Dai et al. Citation1993; Yoshimura et al. Citation2004). The observation that transpiration is more reduced in salt-sensitive than in salt-tolerant genotype (Azevedo Neto et al. Citation2004) further supports this hypothesis.
Several researchers report that salinity increases Ala content in plants (Pulich Citation1986; Fougère et al. Citation1991; Gilbert et al. Citation1998; El-Shintinawy and El-Shourbagy Citation2001). Ala synthesis represents a major metabolic fate of Glu; over 30% of the Glu available for amino acid biosynthesis (other than glutamine) is used to sustain Ala synthesis in unadapted cells, whereas over 55% of the available Glu is used to sustain Ala synthesis in stress-adapted cells (Rhodes et al. Citation1986). The increase of Ala in stressed leaves () suggests that glycolysis and thus, pyruvate content and respiration, were increased to sustain the higher energy demand resulting from stress conditions or to provide carbon skeletons for the photorespiratory cycle (Di Martino et al. Citation2003). The salt-induced increases of Ala, Ser, and Gly contents in conjunction with the fact that salinity did not change the Glu content in both genotypes supports the idea of an increased utilization of Glu as substrate for transamination reactions under salinity conditions.
In response to the salt stress, changes in both Glu and Gly contents were found to be associated with Cys synthesis (El-Shintinawy and El-Shourbagy Citation2001). These three amino acids are precursors of glutathione, a molecule that plays a pivotal role in the antioxidative system (Noctor and Foyer Citation1998). Gly and Cys contents were higher in salt-stressed leaves of BR5011 than in BR5033. By considering that the increases in Gly and Cys contents were a result of a decrease in glutathione synthesis, these results corroborate the findings of Azevedo Neto et al. (Citation2006), in that the BR5033 genotype is more tolerant to salt-induced oxidative damage.
The increased leaf () and root () Arg contents in both genotypes by salt stress are in agreement with that observed by other authors (Mattioni et al. Citation1997; Santa-Cruz et al. Citation1998, Citation1999). A possible explanation for Arg accumulation during phosphorus deficiency has arisen from the work by Rabe and Lovatt (Citation1984), which may be applicable to other stresses. They reported that Arg accumulation during stress was the result of de novo synthesis, which serves to detoxify ammonia accumulation under periods of reduced growth (Rabe and Lovatt Citation1984). Such an explanation may be extensive to His, which also increased after stress, and, like Arg, is related to nitrogen storage (Rabe Citation1990). By considering that the synthesis of nitrogenous compounds containing at least two amino groups may be induced in response to salt stress and that these compounds may serve as important nitrogen sources for metabolic pathways (Gilbert et al. Citation1998), our results suggest that Arg and His accumulation may, at least in part, be involved in the ammonia detoxification process.
In stressed leaves, the amino acids that underwent the largest decrease were mostly the end products of their amino acid families: Ile (aspartato family) and Leu (pyruvate family). Threonine and pyruvate are, respectively, biosynthetic precursors of these amino acids, which are regulated by a common enzyme, the acetolactate sintase, which is cooperatively inhibited by Leu and Val (Miflin and Lea Citation1977). By considering that pyruvate content was not limitative, and that salt stress increased Thr content (), the decrease in isoleucine and leucine () could be the result of a salt induced inhibition of acetolactate sintase activity, as hypothesized by El-Shintinawy and El-Shourbagy (Citation2001). Changes in Ile and Leu contents have also been associated with protein synthesis or protein degradation (Raggi Citation1994; Di Martino et al. Citation2003); thus Ile and Leu reductions may indicate an increase in protein synthesis. At least for BR5011, this hypothesis is supported, since salinity increased soluble proteins in leaves of this genotype.
Pro accumulation occurs in response to several biotic and abiotic stresses resulting, mainly, from an increased synthesis and/or inhibited oxidation of this amino acid (Hare and Cress Citation1997). Our results show that the increases in Pro contents by salt stress were smaller than those observed in the other amino acids. These results may be related to Glu content that did not change in leaves () and decreased in roots (). Glu is a common substrate for the biosynthesis of several amino acids (Miflin and Lea Citation1977). Enzymes of different biosynthetic pathways may be competing for substrate and, therefore, the accumulation of a certain amino acid may depend, at least in part, on a delicate balance among enzyme activities and substrate availability (Vance and Zaerr Citation1990).
It has been suggested that Pro accumulation in response to extreme temperatures and salinity could be due to a disturbance in tissue water status comparable with that observed during drought stress (Lacerda et al. Citation2003; Silva et al. Citation2003). Although this is a plausible explanation for temperature and salt stress, it does not necessarily explain the quick Pro accumulation during the other stressful conditions, such as pathologies, anoxia and nutrient deficiencies (Rabe Citation1990). A more common denominator among the stresses may be the fact that they result in a reduced growth rate. Thus, Rabe (Citation1990) suggested that the maintenance of nitrogen absorption and reduction processes in conjunction with a reduced growth rate leads to ammonia accumulation and subsequent detoxification by sequestering it into certain nitrogenous compounds, such as Pro, Arg, and His.
Several explanations for free amino acids accumulation in plants under salt stress have been suggested. These include increase of amino acids synthesis, inhibition of amino acids degradation, inhibition of protein synthesis and protein degradation (Dhindsa and Cleland Citation1975; Ranieri et al. Citation1989; Roy-Macauley et al. Citation1992; Mansour Citation2000). Our results suggest that the increase of de novo synthesis and/or inhibition of amino acids degradation were the prevalent mechanism for the accumulation of most free amino acids. The observation that salinity increased soluble amino acids content in leaves and roots, in conjunction with soluble protein results that do not indicate protein degradation, supports this hypothesis.
Although salt stress has increased the content of most amino acids, the pattern of amino acids did not show substantial differences between control and stressed plants during the experimental period. Pro, Thr, Arg, Ser, Asp, and Gly accounted for more than 80% of total amino acids in leaves, and more than 60% in roots. Pro was the preponderant amino acid and comprised 20–35% of the total pool, regardless of genotype, organ or treatment considered. Therefore, it could be hypothesized that Pro, Thr, Arg, Ser, Asp, and Gly were the amino acids that most contributed for the decrease in cellular osmotic potential in both leaves and roots of the studied genotypes.
The results of soluble amino acids in salt-stressed leaves and roots of both genotypes suggest that the accumulation of these solutes, mainly in roots of BR5033, may have an important role in the tolerance of this genotype to salt stress. Considerable variations in the accumulation of soluble proteins and N-containing compounds have been shown among crop species differing in salt tolerance. In addition, the physiological and biochemical roles of these compounds in salt tolerance are still quite speculative. Although the information regarding organic solute's role in adaptation of plants to salinity are insufficient to warrant the conclusion that they are associated with salt tolerance, this does not preclude its role as physiological markers of salt tolerance in the breeding programs of some crop species.
Acknowledgements
We thank the Conselho Nacional de Desenvolvimento Científico e Tecnológico (CNPq) and Coordenação de Aperfeiçoamento de Pessoal de Nível Superior (CAPES) for financial support over the years.
References
- Ashraf , M and Harris , PJC . 2004 . Potential biochemical indicators of salinity tolerance in plants . Plant Sci. , 166 ( 1 ) : 3 – 16 .
- Ashraf , M and O'Leary , JW . 1999 . Changes in soluble proteins in spring wheat stressed with sodium chloride . Biol Plant. , 42 ( 1 ) : 113 – 117 .
- Azevedo Neto , AD , Prisco , JT , Enéas-Filho , J , Lacerda , CF , Silva , JV , Costa , PHA and Gomes-Filho , E . 2004 . Effects of salt stress on plant growth, stomatal response and solute accumulation of different maize genotypes . Braz J Plant Physiol. , 16 ( 1 ) : 31 – 38 .
- Azevedo Neto , AD , Prisco , JT , Enéas-Filho , J , Abreu , CEB and Gomes-Filho , E . 2006 . Effect of salt stress on antioxidative enzymes and lipid peroxidation in leaves and roots of salt-tolerant and salt-sensitive maize genotypes . Environ Exp Bot. , 56 ( 1 ) : 87 – 94 .
- Binzel , ML , Hasegawa , PM , Handa , AK and Bressan , RA . 1985 . Adaptation of tobacco cells to NaCl . Plant Physiol. , 79 ( 1 ) : 118 – 125 .
- Bohnert , HJ and Shen , B. 1999 . Transformation and compatible solutes . Sci Hortic. , 78 ( 1–4 ) : 237 – 260 .
- Bradford , MM . 1976 . A rapid and sensitive method for the quantitation of microgram quantities of protein utilizing the principle of protein-dye binding . Anal Biochem. , 72 ( 1–2 ) : 248 – 254 .
- Bray EA , Bailey-Serres J , Weretilnyk E. 2000 . Biochemistry and molecular biology of plants . Rockville : ASPP . Chapter 22, Responses to abiotic stresses . p. 1158 – 1203 .
- Champigny , M-L and Foyer , CH . 1992 . Nitrate activation of cytosolic protein kinases diverts photosynthetic carbon from sucrose to amino acid biosynthesis . Plant Physiol. , 100 ( 1 ) : 7 – 12 .
- Cohen , SA , Meys , M and Tarvin , TL . 1989 . The Pico Tag Method. A manual of advanced techniques for amino acid analysis , Bedford, MA : Millipore .
- Dai , Z , Ku , MSB and Edwardk , CE . 1993 . C4 Photosynthesis: The CO2 concentrating mechanism and photorespiration . Plant Physiol. , 103 ( 1 ) : 83 – 90 .
- Delauney , AJ and Verma , DPS . 1993 . Proline biosynthesis and osmoregulation in plants . Plant J. , 4 ( 2 ) : 215 – 223 .
- De Veau , EJ and Burris , JE . 1989 . Photorespiratory rates in wheat and maize as determined by 18O-labeling . Plant Physiol. , 90 ( 2 ) : 500 – 511 .
- Dhindsa , RS and Cleland , RE . 1975 . Water stress and protein synthesis . Plant Physiol. , 55 ( 4 ) : 781 – 788 .
- Di Martino , C , Delfine , S , Pizzuto , R , Loreto , F and Fuggi , A . 2003 . Free amino acids and glycine betaine in leaf osmoregulation of spinach responding to increasing salt stress . New Phytol. , 158 ( 3 ) : 455 – 463 .
- El-Shintinawy , F and El-Shourbagy , MN . 2001 . Alleviation of changes in protein metabolism in NaCl-stressed wheat seedlings by tiamine . Biol Plant. , 44 ( 4 ) : 541 – 545 .
- Ferrario-Méry , S , Valadier , M-H and Foyer , CH . 1998 . Overexpression of nitrate reductase in tobacco delays drought-induced decreases in nitrate reductase activity and mRNA . Plant Physiol. , 117 ( 1 ) : 293 – 302 .
- Fougère , F , Le Rudulier , D and Streeter , JG . 1991 . Effects of salt stress on amino acid, organic acid, and carbohydrate composition of roots, bacteroids, and cytosol of alfalfa (Medicago sativa L.) . Plant Physiol. , 96 ( 4 ) : 1228 – 1236 .
- Gilbert , GA , Gadush , MV , Wilson , C and Madore , MA . 1998 . Amino acid accumulation in sink and source tissues of Coleus blumei Benth. during salinity stress . J Exp Bot. , 49 ( 318 ) : 107 – 114 .
- Hare , PD and Cress , WA . 1997 . Metabolic implications of stress-induced proline accumulation in plants . Plant Growth Regul. , 21 ( 2 ) : 79 – 102 .
- Hasegawa , PM , Bressan , RA , Zhu , J-K and Bohnert , HJ . 2000 . Plant cellular and molecular responses to high salinity . Annu Rev Plant Physiol Mol Biol. , 51 : 463 – 499 .
- Lacerda , CF , Cambraia , J , Oliva , MAO , Ruiz , HA and Prisco , JT . 2003 . Solute accumulation and distribution during shoot and leaf development in two sorghum genotypes under salt stress . Environ Exp Bot. , 49 ( 2 ) : 107 – 120 .
- Larsson , M . 1992 . Translocation of nitrogen in osmotically stressed wheat seedlings . Plant Cell Environ. , 15 ( 4 ) : 447 – 453 .
- Lawlor , DW and Fock , H . 1978 . Photosynthesis, respiration, and carbon assimilation in water-stressed maize at two oxygen concentrations . J Exp Bot. , 29 ( 3 ) : 579 – 593 .
- Mansour , MMF . 2000 . Nitrogen containing compounds and adaptation of plants to salinity stress . Biol Plant. , 43 ( 4 ) : 491 – 500 .
- Mattioni , C , Lacerenza , NG , Troccoli , A , De Leonardis , AM and Di Fonzo , N . 1997 . Water and salt stress-induced alterations in proline metabolism of Triticum durum seedlings . Physiol Plant. , 101 ( 4 ) : 787 – 792 .
- Miflin , BJ and Lea , PJ . 1977 . Amino acid metabolism . Annu Rev Plant Physiol. , 28 : 299 – 329 .
- Noctor , G and Foyer , CH . 1998 . Ascorbate and glutathione: Keeping active oxygen under control . Annu Rev Plant Physiol Plant Mol Biol. , 49 : 249 – 279 .
- Olmos , E and Hellin , E . 1996 . Mechanisms of salt tolerance in a cell line of Pisum sativum: Biochemical and physiological aspects . Plant Sci. , 120 ( 1 ) : 37 – 45 .
- Pulich , WM Jr . 1986 . Variations in leaf soluble amino acids and ammonium content in subtropical seagrasses related to salinity stress . Plant Physiol. , 80 ( 1 ) : 283 – 286 .
- Rabe , E . 1990 . Stress physiology: The functional significance of the accumulation of nitrogen-containing compounds . J Hortic Sci. , 65 ( 2 ) : 231 – 243 .
- Rabe , E and Lovatt , C . 1984 . De novo arginine biosynthesis in leaves of phosphorus-deficient Citrus and Poncirus species . Plant Physiol. , 76 ( 3 ) : 747 – 752 .
- Raggi , V . 1994 . Changes in free amino acids and osmotic adjustment in leaves of water-stressed bean . Physiol Plant. , 91 ( 3 ) : 427 – 434 .
- Ranieri , A , Bernardi , R , Lanese , P and Soldatini , GF . 1989 . Changes in free amino acid content and protein pattern of maize seedlings under water stress . Environ Exp Bot. , 29 ( 3 ) : 351 – 357 .
- Rhodes , D , Handa , S and Bressan , RA . 1986 . Metabolic changes associated with adaptation of plant cells to water stress . Plant Physiol. , 82 ( 4 ) : 890 – 903 .
- Richardson , SG and McCree , KJ . 1985 . Carbon balance and water relations of sorghum exposed to salt and water stress . Plant Physiol. , 79 ( 4 ) : 1015 – 1020 .
- Rodríguez , HG , Roberts , JKM , Jordan , WR and Drew , MC . 1997 . Growth, water relations, and accumulation of organic and inorganic solutes in roots of maize seedlings during salt stress . Plant Physiol. , 113 ( 3 ) : 881 – 893 .
- Roy-Macauley , H , Zuily-Fodil , Y , Kidric , M , Thi , ATP and Silva , JV . 1992 . Effect of drought stress on proteolytic activities in Phaseolus and Vigna leaves from sensitive and resistant plants . Physiol Plant. , 85 ( 1 ) : 90 – 96 .
- Santa-Cruz , A , Acosta , M , Rus , A and Bolarin , MC . 1999 . Short-term salt tolerance mechanisms in differentially salt tolerant tomato species . Plant Physiol Biochem. , 37 ( 1 ) : 65 – 71 .
- Santa-Cruz , A , Perez-Alfocea , F , Caro , M and Acosta , M . 1998 . Polyamines as short-term salt tolerance traits in tomato . Plant Sci. , 138 ( 1 ) : 9 – 16 .
- Silva , JV , Lacerda , CF , Costa , PHA , Enéas-Filho , J , Gomes-Filho , E and Prisco , JT . 2003 . Physiological responses of NaCl stressed cowpea plants grown in nutrient solution supplemented with CaCl2 . Braz J Plant Physiol. , 15 ( 2 ) : 99 – 105 .
- Singh , NK , Bracken , CA , Hasegawa , PM , Handa , AK , Buckel , S , Hermodson , MA , Pfankoch , F , Regnier , FE and Bressan , RA . 1987 . Characterization of osmotin. A thaumatin-like protein associated with osmotic adjustment in plant cells . Plant Physiol. , 85 ( 2 ) : 529 – 536 .
- Smirnoff , N and Cumbes , QJ . 1989 . Hydroxyl radical scavenging activity of compatible solutes . Phytochemistry , 28 ( 4 ) : 1057 – 1060 .
- Strange , K . 2004 . Cellular volume homeostasis . Adv Physiol Edu. , 28 ( 4 ) : 155 – 159 .
- Thakur , PS and Rai , VK . 1982 . Dynamics of amino acid accumulation of two differentially drought resistant Zea mays cultivars in response to osmotic stress . Environ Exp Bot. , 22 ( 2 ) : 221 – 226 .
- Tully , RE , Hanson , AD and Nelsen , CE . 1979 . Proline accumulation in water-stressed barley leaves in relation to translocation and the nitrogen budget . Plant Physiol. , 63 ( 3 ) : 518 – 523 .
- Vance , NC and Zaerr , JB . 1990 . Analysis by high-performance liquid chromatography of free amino acids extracted from needles of drought-stressed and shaded Pinus ponderosa seedlings . Physiol Plant. , 79 ( 1 ) : 23 – 30 .
- Yemm , EW and Cocking , EC . 1955 . The determination of amino-acids with ninhydrin . Analyst. , 80 ( 948 ) : 209 – 213 .
- Yoshimura , Y , Kubota , F and Ueno , O . 2004 . Structural and biochemical bases of photorespiration in C4 plants: Quantification of organelles and glycine decarboxylase . Planta. , 220 ( 2 ) : 307 – 317 .
- Zhu , J-K . 2002 . Salt and drought stress signal transduction in plants . Annu Rev Plant Biol. , 53 : 247 – 273 .