Abstract
This study investigated the possible phytotoxicity induced by Phargmites australis on phenotypic and physiological parameters of recipient plants with identification of major inhibitors in the donor plant. This was achieved using aqueous extracts of different organs and root exudates of P. australis in laboratory and greenhouse experiments with Lactuca sativa as the model test plant. The observed reduced liquid imbibition and altered resource mobilization in seeds of L. sativa, in particular an insufficient carbohydrate supply, demonstrated that the onset of germination might be negatively affected by phytotoxicity. Dose-response studies pointed out that oxidative stress through reactive oxygen species production could potentially cause the observed germination and seedling growth reductions. The osmotic effects by mannitol solution on germination as well as growth and physiology at a level of −0.57 and −0.45 bar, respectively, demonstrated that the results from aqueous plant extracts were partially induced by the osmotic potential on and above those levels. Overall, the relative strength of inhibition on measured parameters was the highest in leaf extract, followed by rhizome, root, stem, and inflorescence. Root exudates of P. australis also had negative impacts by reducing germination and growth of plant. High-performance liquid chromatography (HPLC) analysis revealed gallic acid, a potent phytotoxin, as a major compound with an order of leaf >inflorescence>rhizome>root>stem.
Introduction
Ecological disruption due to the invasion of plants is of great concern to conservationists and land managers worldwide. Many studies have been conducted to identify the mechanisms of plant invasiveness such as life history characteristics, physiological properties, changes in genetics, resource competition, and in more recent years allelopathy (Pisula & Meiners Citation2010). Allelopathic potential is an important attribute to the success of an invasive species in natural ecosystems, particularly when the species produces novel biochemical weapons (Callaway & Ridenour Citation2004). Interactions associated with allelochemicals produced by invasive or native plant species have the potential to impact seed germination, seedling growth, development, and establishment of neighboring plant species, as well as of the same species, in both natural and agricultural systems (Dorning & Cipollini Citation2006; Lara-Núñez et al. Citation2006).
Most allelopathy studies are limited to seed germination and growth interference bioassays using aqueous extracts of invasive plants in the laboratory with very few of these studies carried out under more natural conditions (Rice Citation1984; Inderjit et al. Citation2001). In field conditions, several mechanisms of interference might be operative in sequence and simultaneously so it makes it difficult to separate the general interference effects from those of allelopathy. Furthermore, various authors speculate that seed germination may not be the primary site for allelopathic interactions (Stowe Citation1979; Lorenzo et al. Citation2010). Relatively little is known about the physiological and biochemical effects induced by allelochemical phytotoxicity in seed germination and seedling growth (Weir et al. Citation2004; Lorenzo et al. Citation2011) though that contributes to the fitness and survival of plants in competition with other species. In particular, phytotoxicity studies examining the seedling growth response with physiological effects have been identified as useful in understanding the mechanisms of actions (Inderjit & Dakshini Citation1995).
Phragmites australis, a ubiquitous wetland plant, has been considered as one of the most allelopathic and invasive species in the world (Uddin et al. Citation2012). However, the origin of this species is still unclear (Plut et al. Citation2011). It is a perennial graminaceous plant, 3 m tall, which reproduces mainly through rhizomes and, at low frequency, through seeds. It grows in all temperate zones of the world, especially North America, most of the countries in Europe, some part of Canada and Australia (Hocking et al. Citation1983; Kulmatiski et al. Citation2011), being especially common in south-eastern Australia (Morris et al. Citation2008). The distribution and abundance of P. australis have expanded over the last 150 years and in most areas forms dense monocultures (Saltonstall et al. Citation2005). Due to the impacts of P. australis invasions, habitats have been diminished or altered significantly for other flora and fauna causing loss of biodiversity and ecosystem functions (Mack et al. Citation2000). Several studies have identified chemicals within P. australis organs which have antialgal, antifungal, or antibacterial effects (Li & Hu Citation2005). Some chemicals produced by the decomposition of belowground organs of P. australis may be responsible for the die back of P. australis itself (Armstrong & Armstrong Citation1999), and photodegradation of secreted phytotoxins by P. australis executes severe phytotoxicity to other plant species (Rudrappa et al. Citation2009). Previous studies have shown that water extracts of P. australis organs have strong phytotoxic effects on germination and growth of other plant species (Rudrappa et al. Citation2007; Uddin et al. Citation2012). Identified phenolics such as gallic acid in P. asutralis root exudates showed inhibitory effects on the germination and growth of various seeds (Rudrappa et al. Citation2007; Uddin et al. Citation2012), but the physiological and biochemical effects of the extracts have been lacking. Generally, phytotoxic activity in nature depends on the release of a mixture of phytotoxins and joint effects of them rather than an individual chemical (Reigosa et al. Citation1999; Inderjit & Duke Citation2003). Hence, plant extracts with phytotoxins showing synergistic, additive, or antagonistic mixture effects were shown to have higher and longer lasting effects on target species than individual chemical (Inderjit et al. Citation2005; Koul & Walia Citation2009).
It is known that the standard germination process consists of several key phases that include imbibition, catabolism, anabolism, and finally radicle protrusion. Undisturbed reserve mobilization is an important requirement for germination during the catabolic process just after liquid imbibition (Kupidłowska et al. Citation2006). Rudrappa et al. (Citation2007) found that the root-secreted allelochemicals from P. australis deployed a reactive oxygen species (ROS) that is responsible for stress conditions on the affected plant. However, most of the studies focused on the individual organ that makes them difficult and assessment of phytotoxicity of that relative to other organs. Against this background, our study was designed to investigate the possible phytotoxicity emphasizing physiological effects of aqueous extracts of different organs of P. australis to find out the relative importance of phytotoxicity and root exudates of P. australis on the germination processes and subsequent growth of plants. In particular, we investigated the phytotoxic effects of P. australis on seed metabolic activities, namely liquid imbibition and resource mobilization as well as induction of oxidative stress by ROS production and their consequences on seedlings. In addition, we identified chemical compounds in different organs of P. australis.
Materials and methods
Plant materials and extraction
Whole, mature plants of P. australis were collected in January 2011 from natural stands adjacent to Cherry lake (37° 51′ 30″S, 144° 50′ 5″E), a coastal wetland in Altona, Melbourne, Australia. Individual organs (leaf, stem, rhizome, and root) and inflorescences (collected later in April 2011) were dried at 40°C in an oven until the weight was constant. Oven-dried plant samples were ground and sieved (0.5 mm) for experiments. To formulate extracts, 20 g dry ground material of each organ was submerged in 100 mL distilled water (20% extract) and agitated for 24 h on an orbital shaker (Orbital Mixer, EOM5, Ratek Instruments Pty. Ltd, Vic 3155, Australia) at room temperature. The extracts were filtered through cheese cloth, centrifuged at 10,000 rpm for 10 min (Beckman Avanti 30 High Speed Compact Centrifuge, 364105, Beckman Coulter Inc., USA), and sterilized (microfiltration with 0.22 µm pore filter). The treatment with extracts rather than individual chemical in our experiments was due to the satisfactory level of efficacy and became more ecologically realistic. pH of the extracts was determined with a pH meter (Pocket digital pH meter, 99559, Dick-Smith electronics, Australia) and osmotic potential (OP) was calculated in bar using the following equation: OP=Electrical Conductivity (EC)×−0.36 according to McIntyre (Citation1980). During experimental setup, the 20% base concentration was diluted with distilled water to obtain concentrations of 15, 10, 5, 2.5, and 1.25%. All extracts were buffered with 0.01 M sodium phosphate buffer and pH values of the extracts including distilled water (control) were adjusted to 6.5 with 1 N NaOH and 1 N HCl. We used a wide range of doses to ensure that they would encompass the lowest dose for an observable effect, as well as the highest dose for maximal effect for all organs (Belz et al. Citation2007). The OP of all extract concentrations in different organs was in the range of −0.048 to −1.27 bar. In bioassays such as that carried out here, OPs in this range could affect the germination and growth (Laterra & Bazzalo Citation1999), so we also evaluated the OP effects on the above-mentioned parameters of the test species.
Choice of target species
Lactuca sativa var. all the year-round (lettuce), commercially available, was used because of its sensitivity and common use in phytochemical bioassays. Lettuce is commonly used in studies aimed to find out the physiological effects of the plant extracts. The use of known susceptible species is useful for screening, and being a ‘known’ entity allows for easy comparison to other studies. In addition, lettuce is easily grown, minimizing the risk of observed growth differences being due to factors other than the treatments applied in the experiments. Though our previous studies showed phytotoxic effects of P. australis on associated species such as Rumex conglomeratus and Juncus pallidus, it also showed high variability among the measured parameters. Associated species may not be a reliable first-line test species in phytotoxicity studies due to the inherent genetic variability of wild populations.
Laboratory experiments
Effects of extracts on seed imbibition
Seeds of 100 mg were soaked in aqueous extracts of each organ at 5% concentration (OP ranged from −0.18 to −0.35 bar) or in distilled water (control) and the increase in fresh weight was determined at 8, 16, 24, and 32 h of imbibition. Treatments were arranged in a complete randomized design (CRD) with three replicates at 25°C in the dark in a growth chamber (Westinghouse, Electrolux home products, Australia).
Effects of extracts on seed reserve mobilization
Seeds of 100 mg were soaked in either aqueous extracts of each organ at 5% concentration or in distilled water (control) and maintained at 25°C in the dark in a growth chamber with three replicates. Seeds after imbibition of 8 and 32 h were collected and immediately processed for carbohydrate analysis as a measurement of reserve mobilization.
Effects of extracts on germination and seedling growth
Dose-response studies were conducted using seven extract concentrations (0, 1.25, 2.5, 5.0, 10, 15, and 20%) of each organ in Petri dishes (Techno-Plas, Australia). Each organ extract (5 mL) was placed into a sterile 9-cm Petri dish containing two sterile sheets of filter paper (Whatman No. 1). At least, three replicates [each having 25 surface sterilized seeds with 1.5% (v/v) sodium hypochlorite for 1 min and subsequent washed] were used for each treatment. Petri dishes were sealed with parafilm (Pechiney, Plastic Packaging Company, Menasha, WI 54952) then placed in polyethylene bags to prevent water loss by evaporation and to avoid contamination by fungi and bacteria. The prepared dishes were arranged in a CRD and placed in a growth chamber set to 25°C in dark condition. Germination was deemed to have occurred when the radicle protruded beyond the seed coat by at least 1 mm. Germinated seeds in all Petri dishes were counted daily at noon for 8 days, the point where cumulative germination leveled off in all treatments. Germination indices (total germination, speed of germination, coefficient of the rate of germination, speed of accumulated germination), physiological and biochemical parameters [hydrogen peroxide, electrolyte leakage, lipid peroxidation (LP), and dehydrogenase enzyme activity], and biometric characteristics (length and weight of radicle and hypocotyl) were measured at the end of the experiment.
Effects of OPs on germination and growth
Thirteen concentrations of mannitol were evaluated with the corresponding OP: 0, −0.11, −0.17, −0.22, −0.27, −0.37, −0.45, −0.57, −0.67, −0.77, −0.87, −0.97, and −1.30 bar. Mannitol was used due to its inert OP (Wardle et al. Citation1992). Each solution (5 mL) was added to the Petri dishes and followed the same procedures for germination study as well as measurement of biometric and physiological parameters as mentioned previously.
Greenhouse experiments
Spring buds of P. australis with rhizomes attached were collected in September 2011 from Cherry Lake (see above). Each living rhizome was cut to contain exactly one active node. Rhizomes were then replanted as soon as possible (within 6 h) in 7 L plastic pots lined with plastic watertight bags filled with 4 L potting mix. Sixteen replicates were used, half of which contained activated carbon (AC) (40 mL/L substrate) to reduce the allelopathic effect as AC has a high affinity to organic compounds (Hille & Den Ouden Citation2005). To all of the treatments, 1 g/L of mixed pelletised fertilizer (Pivot fertilizer-900; N-P-K: 16-8-9) was added bimonthly. Pots were kept in a natural lit greenhouse at 23±3°C and 12±2°C day/night temperature as well as watered regularly with an auto irrigation system to keep soil moist at a level of 55±5%. The lettuce seeds (50) were sown in each pot during the vegetative stage of P. australis. After 10 days, the plants were counted and harvested for biometric (root-shoot length and weight) and physiological parameters (electrolyte leakage and chlorophyll) measurement. A separate experiment was conducted to test the direct effect of AC only on the rhizomes of P. australis (one rhizome per pot) and lettuce (50 seeds per pot) There were five replicates per treatment (with and without carbon) for a total of 20 pots.
Allelochemicals identification with high-performance liquid chromatography (HPLC)
Dried and ground material of 400 mg of each organ passing through 0.5 mm was placed in a 15 mL eppendorf tube and extracted with 10 mL 50% acidified methanol (HCl was added to obtain a final concentration of 1.2 M). Samples were homogenized using the roller mixer in a cold room at 4°C for 24 h. Then, centrifuged at 10,000 rpm for 10 min at 4°C and the supernatants were collected. The extracts were filtered through a 0.2 µm Phenomenex RC membrane and then analyzed by HPLC. All standards (arbutin, gallic acid, 5-hydroxymethyl furfural, catechin, gentistic acid, chlorogenic acid, epicatechin, coumaric acid, ferulic acid, rutin, and phloridzin) and solvents were purchased from Sigma-Aldrich, Australia. The standard stock solutions were prepared by dissolving standards in milli-Q water to100 µg/mL. For the calibration curves, four additional concentrations (20, 40, 60, and 80 µg/mL) were prepared by the dilution of the stock solutions with water. A HPLC system (Shimadzu, Tokyo, Japan) equipped with a Kinetex PEP 5 µm 100 Å LC Column 250×4.6 mm was used. The mobile phase consisted of 0.1% phosphoric acid in water (mobile phase A) and 0.1% phosphoric acid in acetonitrile (mobile phase B) at flow rate 1 mL/min with a gradient elution program and a 65-min run-time. The gradient elution profile was: 0–30 min, 90:10 (A:B); 30.01–40 min with a linear decrease of A and increase of B to 50:50 (A:B); 40.01–45 min, 10:90 (A:B), 45–55 min with a linear increase of A and decrease of B to 50:50 (A:B), and then back to initial condition. There was a 5-min equilibration time between runs to allow the operating system to stabilize. The injection volume was 20 µL and the temperature was ambient. The analysis was monitored with a photodiode array (PDA) detector at the rate of 220 and 271 nm. All the chemical analyses were done in three replicates and the results were calculated using a standard curve. Confirmation of gallic acid was made by retention time as well as spiking the extracts with the standard of gallic acid in different concentrations on the basis of extracts peak absorbance. In addition, ultraviolet (UV) spectrums of the unknown peak of the extracts were compared to that of gallic acid as well.
Measurement of physiological and biochemical parameters
Carbohydrate concentration
The total amount of all sugars [total nonstructural carbohydrate (TNC), water soluble carbohydrate (WSC)] was measured using a phenol sulphuric acid method modified from Kabeya et al. (Citation2003). Sulfuric acid (0.4 N) and water extractions were made for TNC and WSC determination, respectively. Each sample (~6 mg) was placed in a 100 mL round-bottom flask with 50 mL acid for TNC and water for WSC and then refluxed for 1 h in a boiling water bath. The hot solution was filtered through Whatman No. 42 filter paper. The carbohydrate content of the cooled filtrate was determined spectrophotometrically at 485 nm using the above-mentioned method and glucose solution as a calibration standard at a concentration of 0–50 mg/L.
Hydrogen peroxide content
Hydrogen peroxide (H2O2) levels were measured according to Velikova et al. (Citation2000). Plantlets (100 mg) were homogenized with 5 mL of 0.1% trichloroacetic acid (TCA) and centrifuged at 12,000 rpm for 15 min. Then, 0.5 mL supernatant was mixed with 0.5 mL of a 10 mM phosphate buffer (pH 7.0) and 1 mL of 1 M potassium iodide (KI). The absorbance was measured at 390 nm and the amount of H2O2 was calculated using the extinction coefficient 0.28 µM−1 cm−1 (Singh et al. Citation2006).
Electrolyte leakage
Germinated seeds of 100 mg (control and treatment) were placed in 15 cm3 distilled water at room temperature in darkness. EC in the medium was measured with an EC meter after 2 h of incubation. Results were expressed as the percentage of total leakage from seedlings boiled for 20 min (Bogatek et al. Citation2006).
Lipid peroxidation
LP was measured in terms of malondialdehyde (MDA) content. The MDA level was used as an index of LP and was expressed as nmol g−1 fresh weight (f. wt) (Jambunathan Citation2010). About 200 mg of control and treated plantlets were homogenized with 4 mL of 0.1% TCA, and then centrifuged at 15,000 rpm for 15 min. Supernatant of 1 mL was mixed with 2 mL of 20% TCA and 2 mL of 0.5% thiobarbituric acid (TBA). The mixture was heated at 95°C in a fume hood and later cooled with running tap water. The absorbance was read at 532 and 600 nm using a spectrophotometer. A600 is the nonspecific absorbance and was subtracted from the values for A532. The MDA amount was calculated using an extinction coefficient of 155 mM−1cm−1.
Dehydrogenase enzyme activity
Lettuce plantlets (100 mg fresh weight) were washed, blotter-dried, and soaked in 5 mL of 2 g L−1 2,3,5-triphenyl tetrazolium chloride (TTC) at 37°C for 4 h in the dark. Thereafter, 0.5 mL of 1 M sulphuric acid was added to stop the reaction. The plantlets were removed, washed with distilled water, blotter-dried, and ground by mortar and pestle with 7 mL ethyl acetate. The extract was centrifuged at 20,000 rpm for 15 min (Sengar & Srivastava Citation1995). The resultant volume was increased to 7 mL with ethyl acetate due to loss by evaporation, and the absorbance was read at 485 nm with a spectrophotometer to measure formazan production as a measurement of dehydrogenase enzyme activity comparing it with percentage of control.
Chlorophyll
Chlorophyll a (Chl a), chlorophyll b (Chl b), and total chlorophyll were determined by placing about 15 mg of fresh leaf in 7 ml of N-N dimethylformamide (DMF) for 24 h in the dark at 4°C for extraction (Moran & Porath Citation1980). The absorbance of the extracts was measured spectrophotometrically at 664 and 647 nm and chlorophyll was determined following the equation proposed by Inskeep and Bloom (Citation1985).
Statistical analysis
All the experiments were conducted in a completely randomized design with at least three replicates. Data were analyzed by one way ANOVA using IBM SPSS statistics 20.0. Variance homogeneity was tested using Levenes's test and data were arcsine-square root transformed only for percentage of germination and natural log for carbohydrate using SPSS to compensate for variation within population. Significance tests were performed after applying Dunnett's two-sided test at the 0.05 probability level. Furthermore, results were subjected to linear regression analysis and the correlation coefficient values, evaluating the dependence of each measured variables on the concentration of the extracts, were calculated using Microsoft Excel 2010 according to Singh et al. (Citation2002). Coefficient values from regressions were also used to compare the relative strength of plant extracts on measured parameters (Pisula & Meiners Citation2010). ANOVA table of regression analysis gives the F statistic for testing the claim of whether a significant relationship exists. LC50 was measured by graphical estimation as the concentration of the extracts causing 50% mortality of the test seeds. An attempt has been made to differentiate the phytotoxic effects from osmotic effects by plant extracts using osmotically adjusted control values in developing calibration curves containing identical OP to the plant extracts used in this study. After that the various adjustments were made, and t-tests were used to perform the analysis.
Results
Seed imbibition
Water uptake by the seeds of lettuce during the first 8 h after treatment was rapid in all extracts, but no significant variations were observed at 8 and 16 h (). After 8 h, the rate of imbibition was slowed and control seeds gained more than 100% weight, this figure being lower in all extracts. By 24 h, leaf and rhizome extracts showed a significant difference compared to control. A more significant effect was observed at 32 h when leaf, rhizome, and root extracts showed a reduced imbibition compared to the control by 10, 9, and 6%, respectively, while there was no significant effect observed in inflorescence and stem extracts ().
![Figure 1. Effect of aqueous extracts (5%) of different organs of P. australis on liquid imbibitions of lettuce seeds expressed in fresh weight [mg. (100 mg seed)−1] throughout 32 h. Values are mean±standard error (n=3). ***, ** and * indicate significant differences from control at P ≤ 0.001, P ≤ 0.01 and P ≤ 0.05 respectively after Dunnett's test.](/cms/asset/be3cd973-007a-4fad-bb34-77004816d32b/tjpi_a_835879_f0001_b.jpg)
Carbohydrate metabolism
Leaf and rhizome extracts significantly affected the total carbohydrates both after 8 and 32 h, while the other organ extracts affected carbohydrate metabolism only at 32 h (). The extracts had profound effects on preventing the loss of TNC and the buildup of WSC. After 32 h, 31% of TNC had been metabolized in the control whereas leaf and rhizome extracts treatments showed six- and threefold lower carbohydrate metabolization, respectively. Leaf and rhizome extracts also significantly increased WSC by 23% compared to the control, while the increase was only 8% both in leaf and rhizome treatments.
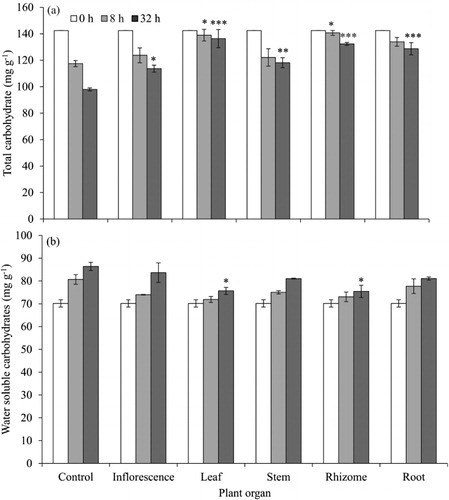
Germination, growth, and physiology
The dose and nature of different extracts of P. australis significantly affected germination, growth, and bio-physiological parameters of lettuce seeds (). The ANOVA table shows that the effect of each organ extract on each measured parameters significantly differed among concentrations. Furthermore, different extract concentrations had differential effects on the germination and growth of lettuce seeds (). The LC50 concentrations for germination of lettuce seeds were 4.7, 8.5, and 11.3% for leaf, root, and rhizome extracts, respectively (). In the case of inflorescence and stem extracts, the response in germination was insufficient to estimate LC50 values. In the leaf extract, no germination occurred at 15 and 20% concentrations, whereas other extracts showed higher germination percentages at these concentrations. The percentage germination of lettuce seeds was reduced at all concentrations except for the inflorescence extract. Decreased germination was coupled with increasing concentration, exhibiting a strong inverse relationship (). The inhibition threshold varied among organs but was less than 1.25% for leaf, rhizome, and root extracts compared to the germination percentage of control. In addition, the speed of germination was inversely related to all extract concentrations (). Leaf extract significantly reduced the speed of germination, ranging from 0.06 at 10% to 12.5 at control followed by rhizome, root, and stem extracts and finally the inflorescence extract. The subsequent growth of seedlings, measured in terms of radicle and hypocotyl length and weight, was significantly reduced at every concentration of leaf and rhizome extracts, whereas other organ extracts showed contrasting effects ( and ). In general, the inhibitory effect was greater on radicle growth () than on hypocotyl growth (data not shown). Radicle length was reduced by 49 and 34% in response to the lowest concentration (1.25%) of leaf and rhizome extracts, respectively.
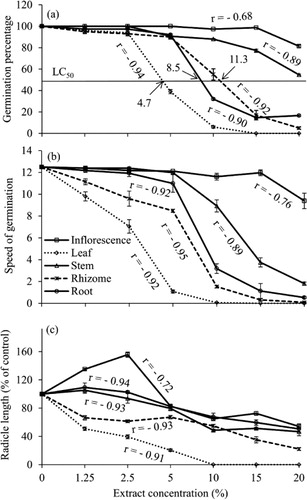
Table 1. Results of two way ANOVA (F-ratios) showing the effects of different organ materials (M) of P. australis, extract concentration (C) and their interaction (M×C) on germination indices, biometric, and biochemical parameters in lettuce.
The H2O2 content of plantlets increased in response to aqueous extracts of P. australis compared to the control and the increase was concentration dependent (). A significant effect was observed in the leaf extract, followed by rhizome, root, inflorescence, and stem extracts. The increase was significant even at 1.25% concentration of the leaf extract or higher for other organs extracts. At 10% concentration of leaf and rhizome extracts, there was nearly a 10- and 6-fold increase in the H2O2 content compared to the control, respectively. The dehydrogenase activity in treated plantlets was investigated using TTC reduction measurement. Lettuce plantlets treated with different extracts had significantly reduced TTC to formazan compared to the control. Both leaf and root extracts decreased the dehydrogenase enzyme activity even at 1.25% concentration and all extracts significantly reduced enzyme activity at 2.5% or higher (). The highest inhibition (80%) was observed in the 10% leaf extract followed by rhizome (76%) and root (69%) extracts. There was an inverse correlation between H2O2 production and enzyme activity (r=−0.69). There was a distinct decline in dehydrogenase enzyme activity with increasing concentration of P. australis extracts; in particular, the leaf extract exhibited a higher inhibition than extracts from other organs.
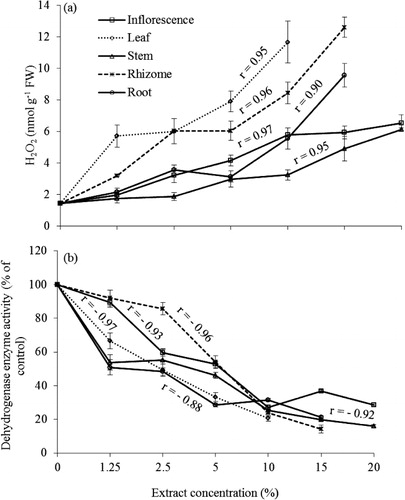
All aqueous extracts caused significant cell membrane injury measured by ion leakage from plantlets of lettuce as indicated by accumulative EC in the bathing solution with increasing concentration (). The root extract treatments showed highest ion leakage (23%) followed by leaf (16%) and rhizome (14%) extracts at 10% concentration. The aqueous extracts significantly increased the MDA content in plantlets of lettuce with increasing concentration with the exception of the 1.25% concentration (p<0.05) (). MDA was most abundant in plantlets exposed to leaf extracts (fourfold) compared to the control at 10% concentration followed by the rhizome, root, stem and inflorescence extracts. Increased MDA contents corresponded to the LP that caused membrane damage. There was also a strong positive correlation between ROS as H2O2 and LP (r=0.88). In the leaf extract, the rise of MDA content with increasing concentration was observed to be steep. Enhanced LP and electrolyte leakage resulting in loss of membrane integrity were among the key factors to determine cellular injury. LP was related to decreasing membrane permeability and our results showed a strong positive correlation between LP and cell membrane injury (r=0.62). In some cases, data were not available for high concentration of leaf, rhizome, and root extracts due to no germination and small amount of plant sample.
OP effects
The OP of all organ extracts ranged from −0.05 to −1.27 bar at concentrations of 1.25–20% with highest values in root and lowest values in inflorescence extracts (Table S1 Footnote1). There were significant differences in OP among plant organs for the same extract concentration. Among those, the extracts at 10% had an OP lower than −0.506 bar except leaf (−0.62 bar) and root (−0.63 bar) extracts. Our experimental results showed that there was no significant effect on germination percentage until −0.45 bar but effects existed on seedling development at −0.45 bar and plant physiology at −0.27 bar and afterward (). OPs affected growth and physiology more than germination. Additional increase of OP from −0.77 to −1.30 bar inhibited germination significantly and even no germination (). On comparing these results with the results of aqueous plant extracts, it may be seen that the observed inhibition of leaf and rhizome extracts was more severe than the osmotic effects alone, and therefore the inhibitory effects were most likely due to phytotoxins present in the extracts (). In addition, the inhibition of germination and growth in some cases, such as inflorescence, stem, and root extracts, might have been the results only for OPs but those extracts had some physiological effects through phytotoxicity ().
Table 2. Effect of osmotic potential at various concentrations on germination, growth, and physiological parameters of lettuce. Values are mean±standard error (n=3).
Table 3. Results of paired t-tests showing the effects of different organ of P. australis and extract concentration considering osmotically adjusted control on germination indices, biometric and physiological parameters in lettuce.
Root exudates-mediated effects in greenhouse
P. australis root exudates had strong negative effects on germination, growth, and physiological parameters of lettuce in the greenhouse ( and ). When grown with P. australis, germination percentage and root length and weight were inhibited by 30, 39, and 82%, respectively (). AC treatment significantly increased germination, root length, and total biomass although this was not the case for all parameters measured, i.e. shoot length, electrolyte leakage, and total chlorophyll ( and ). There was a significant negative effect on membrane integrity of lettuce roots grown with P. australis but no significant effect on total chlorophyll (). The overall effect of AC on lettuce in competition with P. australis was positive but all measurements were not significant ( and ). AC had no significant direct effect on total biomass of P. australis and lettuce when they were grown alone (all p's>0.5).
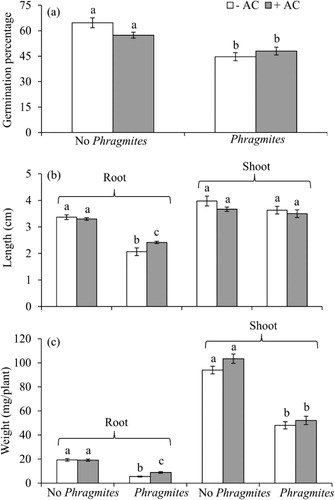
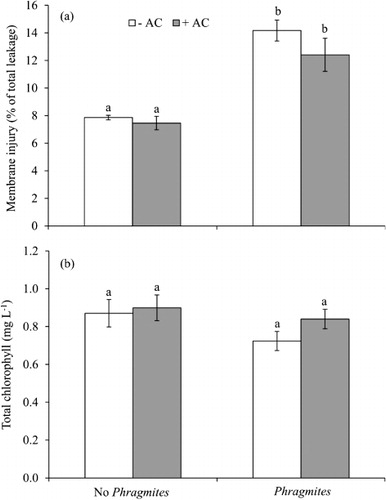
Allelochemicals identification
HPLC analysis revealed the presence of gallic acid with a detectable level among 11 polyphenolics in all organs of P. australis. The HPLC chromatograms of all standards and extracts have been evaluated to confirm the presence of gallic acid on the basis of retention time, spiking, and UV spectrum (Figures S1–S3Footnote1). The concentration in dry weight (DW) basis was maximum in leaf extracts (0.441 µg/mg) followed by inflorescence (0.075 µg/mg), rhizome (0.041 µg/mg), root (0.039 µg/mg), and minimum in stem (0.029 µg/mg). The spiked chromatogram of gallic acid with all extracts confirmed the evidence of the compound (Figures S1 and S2). Some observed peaks before and after gallic acid might be some unidentified compounds from organs involved in the combined phytotoxic activity.
Discussion
The observed seed imbibition results suggest that phenolics concentration may play an important role in water uptake as leaf, rhizome, and root extracts of P. australis contain higher amounts of total phenolics and specific chemical, gallic acid than stem and inflorescence extracts (Uddin et al. Citation2012). Some studies report that the extracts of alfalfa, kudzu, and black mustard had inhibitory effects on seed imbibition (Chon et al. Citation2004; Rashid et al. Citation2010a; Tawaha & Turk Citation2003). Seed imbibition, the first phase of germination, is an important step to germination and if water uptake does not reach a critical level, seeds will not germinate. Delays in reaching this critical moisture content and subsequent transition to the second phase of germination can significantly disrupt metabolism and initiation of germination (Bewley Citation1997). Decreased carbohydrate metabolism in the second phase of the germination process may affect plants growing under stress conditions and specifically may affect total carbohydrate degradation and soluble sugar accumulation (Dubey & Singh Citation1999). The results obtained from our studies on reserve mobilization are in close agreement with the findings of Lara-Núñez et al. (Citation2009) showing that the lechate of Sicyos deppei caused a delay of starch degradation and sucrose hydrolysis which is related to the mobilization of reserve carbohydrates. Thus, the phytotoxic effects on carbohydrate mobilization might negatively influence cellular respiration (Kraus & Lambers Citation2001) and therefore the overall germination process (Fritz & Braun Citation2006).
Dose-response relationships are the most effective ways to study the potential effects of phytotoxins (Belz et al. Citation2007). In general, a gradual decline was observed in germination, radicle length, and weight with increasing concentration, indicating dose-response effects. Our observations showed that the LC50 concentration of the leaf extract was lower than for the other organs extracts indicating that leaf contributes strong phytotoxicity than others. LC50, a useful tool predicting the effects of a potential toxin in the ecosystem, may serve as a benchmark for subsequent studies evaluating the phytotoxic effects of P. australis and other plants (Batish et al. Citation2002; Uddin et al. Citation2012). The findings regarding speed of germination showed that they are the most sensitive index compared to other germination indices for assessing the phytototoxic effect and such type of findings are well documented in other studies (Chiapusio et al. Citation1997; Rashid et al. Citation2010a). In addition, radicle length and biomass were inhibited more than hypocotyl length and biomass parameters, a common finding in phytotoxicity studies (Burgos & Talbert Citation2000). In some cases, the ‘hormesis’ phenomenon, characterized by low-dose stimulation and high-dose inhibition, has been observed significantly by the inflorescence extract in radicle length development and phytotoxins are known to induce hormesis as well (Duke et al. Citation2006). Although it is often assumed that the response of seeds or seedlings to plant extracts is due to allelopathy entirely but our results regarding the inhibition of germination indices and phenotypic characteristics with OP values higher than −0.45 bar indicate that the effects caused by extracts might be partially a negative osmotic effect. Comparing the results of the extracts and the mannitol solution with same OPs showed that some effects of mannitol was lower than those of the extracts indicate that the activity of the some extracts is not only the effects of OP alone, it includes the effects by phytotoxins. Some extract concentrations such as inflorescence, stem, and root were not appreciably more effective in retarding germination, growth, and physiological activity than were mannitol solutions of comparable OPs. The germination, growth, and physiological activity were not depressed significantly at lower concentrations of mannitol solution, but the significant inhibition occurred as the concentrations increased. The inhibition by extracts with high OPs is substantially overestimated regardless of the osmotic effects as is shown clearly in our study and the findings of Wardle et al. (Citation1992). According to Anderson and Loucks (Citation1966), the effects by the extracts with OP no greater than −0.506 bar might be considered as a phytotoxic effect due to presence of toxic compounds in extracts and our results go beyond other findings in the literature indicating a significant effects on physiological activity of the tested species. So, the findings in our study demonstrate that the inhibition attributable to the osmotic influence of the extracts must be considered during the assessment of phytotoxicity and allelopathic potential of plant extracts in water.
ROS are normally produced within plants at very low levels in cells, but their production may be enhanced under biotic and abiotic stresses. In particular H2O2, one major species of ROS, leads to oxidative damage causing LP and cellular damage such as electrolyte leakage and pigment degradation (Batish et al. Citation2006). ROS generation in the exposed plants to some phytotoxins has been recently proposed as one of the modes of action for plant growth inhibition (Weir et al. Citation2004). Our results in H2O2 production are compatible with other phytotoxicity studies where it has been reported that phytotoxins and aqueous extracts of allelopathic plants may cause oxidative damage through the production of ROS and subsequent damage of cellular systems in target plants (Singh et al. Citation2006; Sinha & Saxena Citation2006). The increase of ROS might be directly produced through acute damage of phytotoxins on cells of treated plants and they showed more sensitivity with increasing concentrations indicating that concentration of phytotoxins in ecosystem might be an important factor in demonstrating the allelopathic effects.
In addition, the decrease of dehydrogenase enzyme activity might be a reflection of cell damage due to extract exposure (Hong et al. Citation2008); it may be an index of cell vitality. The decrease of cellular activity upon exposure to different extracts implies an interference with energy metabolism involved in the synthesis of macromolecules which results in reduced plant growth. Singh et al. (Citation2002) demonstrated that respiratory ability of seedlings might be hampered with exposure to phytotoxins. Increased conductivity indicates that cellular membrane disruption results in excessive solute leakage. These results correspond with earlier findings that some phytotoxins cause ion leakage from plant tissue (Singh et al. Citation2006) leading to the induction of oxidative stress (Dayan et al. Citation2000). Bogatek et al. (Citation2006) found that sunflower extracts increase LP with increasing MDA in mustard plantlets. Increased MDA content with increasing extract concentration supports the idea that seed germination might be affected through membrane LP (Bogatek et al. Citation2006; Uddin et al. Citation2012). To avoid the adverse effects by ROS, the cells might utilize energy to retain the expression of some protective genes and synthesis of antioxidant enzymes and compounds (Hong et al. Citation2008) such as catalase and ascorbic peroxidases, which were not studied in this study. A wide range of OPs was used in our experiment linked with plant extract concentrations, to determine whether physiological inhibition resulted from limited water availability. We found that OPs had a potential effect on physiology even at a value of −0.27 bar and afterward but the variability of the inhibitory effects regarding electrolyte leakage, dehydrogenase enzyme activity, and MDA content between solution of mannitol and extracts of similar OP explained that they are not only for osmotic stress but rather phytotoxic effects of the extracts. Additionally, we can point out one of the best characterized phytotoxic mechanisms induced by extracts of P. australis is the direct inhibition of photosystem II (PSII) components that was found in our previous studies (Uddin et al. Citation2012).
The greenhouse study more closely approximated natural conditions, demonstrating that P. australis greatly inhibited germination and suppressed the growth of lettuce seedlings. This experiment showed a substantial reduction of root length, biomass, and several ecophysiological parameters. These results are consistent with several studies which found that P. australis lowered the root biomass of endemic Eriocaulon carsonii due to belowground competition (Davies et al. Citation2010) and showed negative effects on the germination and establishment of Arabidopsis thaliana in vivo conditions while conversely showing a positive effect under AC treatment (Rudrappa et al. Citation2007). Our previous study found that P. australis rhizosphere and infested soil contained total phenolics with a concentration of 1.17 and 1.26 mg g−1, respectively, that might be enough for an inhibitory effect on the associated plant species (Uddin et al. Citation2012). This greenhouse study coupled with our previous results confirms the phytotoxic potential of P. australis for competitive effects on other plant species.
The presence of gallic acid in root exudates of P. australis was previously reported by Rudrappa et al. (Citation2007), but no relative abundance among organs was studied in details. The present study confirmed the rank of abundance among different organs. The effects of the extracts might be a combined role of several other unidentified phytotoxins with the known one. Our results also show that leaf extracts had higher inhibitory effect than other organs. Higher amount of total phenolic compounds (Uddin et al. Citation2012) and specific compound, gallic acid, in the leaves explain the stronger inhibition of leaves when compare to other extracts. More recently, Weidenhamer et al. (Citation2013) contradicted the findings of Rudrappa et al. (Citation2007) and Bains et al. (Citation2009) stating that gallic acid was not present in the high concentration in rhizosphere soil as well as in leaf and rhizome with a concluding remarks that gallic acid might be not a primary explanation for invasion of P. australis. Weidenhamer et al. (Citation2013) reported that leaves and rhizomes of P. australis contained 20−28 µg/g FW and trace amount of gallic acid, respectively, whereas Bains et al. (Citation2009) found 2075–8302 µg/g FW in rhizome sample of invasive population. By contrast, the concentration of gallic acid in our leaf and rhizome samples that were approximately 12- and 13-fold higher, respectively, than those reported by Weidenhamer et al. (Citation2013), whereas rhizome had a concentration of 12.57 µg/g FW gallic acid that were 0.151% of the maximum concentration found by Bains et al. (Citation2009). In light of our results combined with others, it can be said that the concentration and production of allelochemicals in soil and plant organ depends on the density, growth, and phenology of alleopathic plant, habitat, soil type, climate, pattern of cultivation, and agricultural practices (Inderjit & Dakshini Citation1995; Yaber Grass & Leicach Citation2011). In an advanced concept in alleopathy, a biogeographical approach has been considered as crucial for understanding the invasion process (Hierro et al. Citation2005).
Considering the overall results, it seems evident that leaf extract has higher phytotoxicity than other organs. This could explain how leaves may contribute the maximum level of phytotoxin and the associated effects. First, we may consider partially the ‘enemy release hypothesis’ that explains a way of success of invasive species (Colautti et al. Citation2004; Occhipinti et al. Citation2011). Generally, leaves are the main target organs of any plant for herbivores and as the leaves of P. australis contain high amounts of phytotoxins, it is thought to gain a substantial advantage. Because P. australis populations are no longer directly suppressed by herbivores and consequently, they obtain a competitive advantage over natives that enables the plant to be more invasive attaining higher biomass production. This concept is supported by Hendricks et al. (Citation2011) who suggested that phenolic compounds in leaf might be involved in chemical defense of P. australis against herbivores and neighboring plants. In addition, Chludil et al. (Citation2012) reported that leaves of Lupinus angustifolias as with most species of Fabaceae are capable of producing chemical defenses against herbivores and pathogenic microorganisms that facilitate the biological invasion of those species. Secondly, P. australis-dominated ecosystems produce large volumes of above and belowground biomass (Park & Blossey Citation2008). Total biomass production of more than 200 t ha−1 have been recorded (Engloner Citation2009) making P. australis one of the largest biomass producers in aquatic ecosystems. The worldwide distribution and the extremely large areas covered by P. australis stands may have a considerable effect on the accumulation of phytotoxins through decomposition in wetlands. Deposition of phytotoxins on this scale may actually create a ‘leading edge’ of plant death and damage (Cruz-Ortega et al. Citation2002; Rashid et al. Citation2010b). In addition, our field observations indicate that most of the wetlands occupied by P. australis have a thick bed of residue especially leaves and we suspect that most phytotoxins are released into the soil through decomposition of leaf as it decomposes readily. Some long-term invaded wetlands in Australia have no other plant species recorded within the stands of P. australis compared to newly invaded sites that are floristically more diverse. The absence of other species in long-established stands may be due to the formation of more toxic and persistent breakdown products over time, mainly from accumulation of leaf materials and breakdown compounds.
In conclusion, our studies conferred that P. australis has phytotoxic effects on germination processes and seedling development of plant species, lettuce combined with osmotic effects of aqueous plant extracts by a range of −0.45 to −1.30 bar. Initial inhibition of imbibition by seeds reflects the phytotoxic effects that alter the ability of the seeds reaching the critical water level to increase metabolism and for initiation of germination. Consequently, alterations in reserve carbohydrate (TNC and WSC) mobilization could negatively affect germination and root cell expansion. Increasing ROS as H2O2 in plantlets enhanced LP in the cells, further strengthened the extent of membrane injury as electrolyte leakage and decreased dehydrogenase enzyme activity. Considering all results, leaf extracts had the greatest inhibitory effects among all organs followed by rhizome, root, stem, and inflorescence. These correlated and combined effects of aqueous extracts of P. australis cause oxidative stress in cells of impacted seedlings that can result in cell death and ultimately inhibit the growth of plants and may lead to plant death. P. australis may appear to exude phytotoxins that might have substantial negative effects on the germination and growth of lettuce. Again, this study confirms the presence of gallic acid in different organs of P. australis but further studies are required to identify more in details about the known and unknown compounds and their phytotoxicity. In addition, whether the induction of oxidative stress associated with the increased defense mechanisms by antioxidant enzyme activity in the cells of the plant persists is a question to be studied in further research. This study provides support for investigating the hypothesis that P. australis may exert allelopathic effects that facilitate invasion, and to identify specific further experiments that would be required to further examine the hypothesis of allelopathic effects.
Supplemental_Content.doc
Download MS Word (709 KB)Acknowledgments
This study was funded by an International Postgraduate Research Scholarship (IPRS), Australia to the first author. The authors thank all laboratory technicians in the ecology group in Victoria University, Melbourne for their kind help in sample collection from the field and for technical support associated with all experiment settings and parameter assessments. Special thanks go to Joseph Pelle, Instrumental Technician, Victoria University, Melbourne and Hung Luu, Phenomenex, Melbourne, Australia for HPLC analysis. All fieldworks were carried out under the regulations of Melbourne Water, Victoria, Australia.
Notes
1. Supplemental Content may be viewed online at http://dx.doi.org/10.1080/17429145.2013.835879
References
- Anderson RC, Loucks OL. 1966. Osmotic pressure influence in germination tests for antibiosis. Science. 152:771–773. 10.1126/science.152.3723.771
- Armstrong J, Armstrong W. 1999. Phragmites die-back: toxic effects of propionic, butyric and caproic acids in relation to pH. New Phytol. 142:201–217. 10.1046/j.1469-8137.1999.00395.x
- Bains G, Sampath Kumar A, Rudrappa T, Alff E, Hanson TE, Bais HP. 2009. Native plant and microbial contributions to a negative plant-plant interaction. Plant Physiol. 151:2145–2151. 10.1104/pp.109.146407
- Batish DR, Singh HP, Kohli RK, Saxena DB, Kaur S. 2002. Allelopathic effects of parthenin against two weedy species, Avena fatua and Bidens pilosa. Environ Exp Bot. 47:149–155. 10.1016/S0098-8472(01)00122-8
- Batish DR, Singh HP, Setia N, Kaur S, Kohli RK. 2006. 2-Benzoxazolinone (BOA) induced oxidative stress, lipid peroxidation and changes in some antioxidant enzyme activities in mung bean (Phaseolus aureus). Plant Physiol Biochem. 44:819–827. 10.1016/j.plaphy.2006.10.014
- Belz RG, Velini ED, Duke SO. 2007. Dose/response relationships in allelopathy research. In: Fujii Y, Hiradate S, editors. Allelopathy: new concepts and methodologies. Enfield (NH): Science Publishers; p. 3–29.
- Bewley JD. 1997. Seed germination and dormancy. The Plant Cell. 9:1055. 10.1105/tpc.9.7.1055
- Bogatek R, Gniazdowska A, Zakrzewska W, Oracz K, Gawronski S. 2006. Allelopathic effects of sunflower extracts on mustard seed germination and seedling growth. Biol Plant. 50:156–158. 10.1007/s10535-005-0094-6
- Burgos NR, Talbert RE. 2000. Differential activity of Allelochemicals from secale cereale in seedling bioassays. Weed Sci. 48:302–310. 10.1614/0043-1745(2000)048[0302:DAOAFS]2.0.CO;2
- Callaway RM, Ridenour WM. 2004. Novel weapons: invasive success and the evolution of increased competitive ability. Front Ecol Environ. 2:436–443. 10.1890/1540-9295(2004)002[0436:NWISAT]2.0.CO;2
- Chiapusio G, Sanchez A, Reigosa M, Gonzalez L, Pellissier F. 1997. Do germination indices adequately reflect allelochemical effects on the germination process? J Chem Ecol. 23:2445–2453. 10.1023/B:JOEC.0000006658.27633.15
- Chludil HD, Leicach SR, Corbino GB, Barriga LG, Vilariño MdP. 2012. Genistin and quinolizidine alkaloid induction in L. angustifolius aerial parts in response to mechanical damage. J Plant Interact. 8:117–124. 10.1080/17429145.2012.672660
- Chon SU, Nelson C, Coutts J. 2004. Osmotic and autotoxic effects of leaf extracts on germination and seedling growth of alfalfa. Agron J. 96:1673–1679. 10.2134/agronj2004.1673
- Colautti RI, Ricciardi A, Grigorovich IA, MacIsaac HJ. 2004. Is invasion success explained by the enemy release hypothesis? Ecol Lett. 7:721–733. 10.1111/j.1461-0248.2004.00616.x
- Cruz-Ortega R, Ayala-Cordero G, Anaya AL. 2002. Allelochemical stress produced by the aqueous leachate of Callicarpa acuminata: effects on roots of bean, maize, and tomato. Physiol Plant. 116:20–27. 10.1034/j.1399-3054.2002.1160103.x
- Davies RJP, Mackay DA, Whalen MA. 2010. Competitive effects of Phragmites australis on the endangered artesian spring endemic Eriocaulon carsonii. Aquat Bot. 92:245–249. 10.1016/j.aquabot.2009.12.003
- Dayan FE, Romagni JG, Duke SO. 2000. Investigating the mode of action of natural phytotoxins. J Chem Ecol. 26:2079–2094. 10.1023/A:1005512331061
- Dorning M, Cipollini D. 2006. Leaf and root extracts of the invasive shrub, Lonicera maackii, inhibit seed germination of three herbs with no autotoxic effects. Plant Ecol. 184:287–296. 10.1007/s11258-005-9073-4
- Dubey R, Singh A. 1999. Salinity induces accumulation of soluble sugars and alters the activity of sugar metabolising enzymes in rice plants. Biol Plant. 42:233–239. 10.1023/A:1002160618700
- Duke SO, Cedergreen N, Velini ED, Belz RG. 2006. Hormesis: is it an important factor in herbicide use and allelopathy? Outlooks Pest Manag. 17:29–33.
- Engloner AI. 2009. Structure, growth dynamics and biomass of reed (Phragmites australis) – a review. Flora. 204:331–346. 10.1016/j.flora.2008.05.001
- Fritz JI, Braun R. 2006. Ecotoxicological effects of benzoxazinone allelochemicals and their metabolites on aquatic nontarget organisms. J Agric Food Chem. 54:1105–1110. 10.1021/jf050917n
- Hendricks LG, Mossop HE, Kicklighter CE. 2011. Palatability and chemical defense of Phragmites australis to the marsh periwinkle snail Littoraria irrorata. J Chem Ecol. 37:838–845. 10.1007/s10886-011-9990-8
- Hierro JL, Maron JL, Callaway RM. 2005. A biogeographical approach to plant invasions: the importance of studying exotics in their introduced and native range. J Ecol. 93:5–15. 10.1111/j.0022-0477.2004.00953.x
- Hille M, Den Ouden J. 2005. Charcoal and activated carbon as adsorbate of phytotoxic compounds – a comparative study. Oikos. 108:202–207. 10.1111/j.0030-1299.2005.13482.x
- Hocking PJ, Finlayson CM, Chick AJ. 1983. The biology of Australian weeds. 12. Phragmites australis (Cav.) Trin. ex Steud. Aust Inst Agr Sci J. 49:123–132.
- Hong Y, Hu H-Y, Li F-M. 2008. Physiological and biochemical effects of allelochemical ethyl 2-methyl acetoacetate (EMA) on cyanobacterium Microcystis aeruginosa. Ecotoxicol Environ Saf. 71:527–534. 10.1016/j.ecoenv.2007.10.010
- Inderjit, Dakshini K. 1995. On laboratory bioassays in allelopathy. Bot Rev. 61:28–44. 10.1007/BF02897150
- Inderjit, Duke SO. 2003. Ecophysiological aspects of allelopathy. Planta. 217:529–539. 10.1007/s00425-003-1054-z
- Inderjit, Kaur M, Foy CL. 2001. On the significance of field studies in allelopathy. Weed Technol. 15:792–797. 10.1614/0890-037X(2001)015[0792:OTSOFS]2.0.CO;2
- Inderjit, Weston LA, Duke SO. 2005. Challenges, achievements and opportunities in allelopathy research. J Plant Interact. 1:69–81. 10.1080/17429140600622535
- Inskeep WP, Bloom PR. 1985. Extinction coefficients of chlorophyll a and b in N,N-Dimethylformamide and 80% acetone. Plant Physiol. 77:483–485. 10.1104/pp.77.2.483
- Jambunathan N. 2010. Determination and detection of reactive oxygen species (ROS), lipid peroxidation, and electrolyte leakage in plants. Plant Stress Tolerance. 639:291–297.
- Kabeya D, Sakai A, Matsui K, Sakai S. 2003. Resprouting ability of Quercus crispula seedlings depends on the vegetation cover of their microhabitats. J Plant Res. 116:207–216. 10.1007/s10265-003-0089-3
- Koul O, Walia S. 2009. Comparing impacts of plant extracts and pure allelochemicals and implications for pest control. CAB Rev Perspect Agric Vet Sci Nutri Nat Res. 4:1–30.
- Kraus E, Lambers H. 2001. Leaf and root respiration of Lolium perenne populations selected for contrasting leaf respiration rates are affected by intra- and interpopulation interactions. Plant Soil. 231:267–274. 10.1023/A:1010336632553
- Kulmatiski A, Beard KH, Meyerson LA, Gibson JR, Mock KE. 2011. Nonnative Phragmites australis Invasion into Utah Wetlands. West N Am Nat. 70:541–552. 10.3398/064.070.0414
- Kupidłowska E, Gniazdowska A, Stępień J, Corbineau F, Vinel D, Skoczowski A, Janeczko A, Bogatek R. 2006. Impact of sunflower (Helianthus annuus L.) extracts upon reserve mobilization and energy metabolism in germinating mustard (Sinapis alba L.) seeds. J Chem Ecol. 32:2569–2583. 10.1007/s10886-006-9183-z
- Lara-Núñez A, Romero-Romero T, Ventura JL, Blancas V, Anaya AL, Cruz-Ortega R. 2006. Allelochemical stress causes inhibition of growth and oxidative damage in Lycopersicon esculentum Mill. Plant Cell Environ. 29:2009–2016. 10.1111/j.1365-3040.2006.01575.x
- Lara-Núñez A, Sánchez-Nieto S, Luisa Anaya A, Cruz-Ortega R. 2009. Phytotoxic effects of Sicyos deppei (Cucurbitaceae) in germinating tomato seeds. Physiol Plant. 136:180–192. 10.1111/j.1399-3054.2009.01228.x
- Laterra P, Bazzalo ME. 1999. Seed-to-seed allelopathic effects between two invaders of burned Pampa grasslands. Weed Res. 39:297–308. 10.1046/j.1365-3180.1999.00146.x
- Li F-M, Hu H-Y. 2005. Isolation and characterization of a novel antialgal allelochemical from Phragmites communis. Appl Environ Microbiol. 71:6545–6553. 10.1128/AEM.71.11.6545-6553.2005
- Lorenzo P, Palomera-Pérez A, Reigosa MJ, González L. 2011. Allelopathic interference of invasive Acacia dealbata Link on the physiological parameters of native understory species. Plant Ecol. 212:403–412. 10.1007/s11258-010-9831-9
- Lorenzo P, Pazos-Malvido E, Reigosa MJ, González L. 2010. Differential responses to allelopathic compounds released by the invasive Acacia dealbata Link (Mimosaceae) indicate stimulation of its own seed. Aust J Bot. 58:546–553. 10.1071/BT10094
- Mack RN, Simberloff D, Mark Lonsdale W, Evans H, Clout M, Bazzaz FA. 2000. Biotic invasions: causes, epidemiology, global consequences, and control. Ecol Appl. 10:689–710. 10.1890/1051-0761(2000)010[0689:BICEGC]2.0.CO;2
- McIntyre DS. 1980. Basic relationships for salinity evaluation from measurements on soil solution. Aust J Soil Res. 18:199–206. 10.1071/SR9800199
- Moran R, Porath D. 1980. Chlorophyll determination in intact tissues using N,N-dimethylformamide. Plant Physiol. 65:478–479. 10.1104/pp.65.3.478
- Morris K, Boon PI, Raulings EJ, White SD. 2008. Floristic shifts in wetlands: the effects of environmental variables on the interaction between Phragmites australis (Common Reed) and Melaleuca ericifolia (Swamp Paperbark). Mar Freshwat Res. 59:187–204. 10.1071/MF07072
- Occhipinti A, Atsbaha Zebelo S, Capuzzo A, Maffei M, Gnavi G. 2011. Chrysolina herbacea modulates jasmonic acid, cis-(+)-12-oxophytodienoic acid, (3R,7S)-jasmonoyl-l-isoleucine, and salicylic acid of local and systemic leaves in the host plant Mentha aquatica. J Plant Interact. 6:99–101. 10.1080/17429145.2010.545282
- Park MG, Blossey B. 2008. Importance of plant traits and herbivory for invasiveness of Phragmites australis (Poaceae). Am J Bot. 95:1557–1568. 10.3732/ajb.0800023
- Pisula NL, Meiners SJ. 2010. Relative allelopathic potential of invasive plant species in a young disturbed woodland. J Torrey Bot Soc. 137:81–87. 10.3159/09-RA-040.1
- Plut K, Paul J, Ciotir C, Major M, Freeland JR. 2011. Origin of non-native Phragmites australis in North America, a common wetland invader. Fund Appl Limnol/Archiv für Hydrobiologie. 179:121–129. 10.1127/1863-9135/2011/0179-0121
- Rashid MH, Asaeda T, Uddin MN. 2010a. The allelopathic Potential of Kudzu (Pueraria montana). Weed Sci. 58:47–55. 10.1614/WS-09-106.1
- Rashid MH, Asaeda T, Uddin MN. 2010b. Litter-mediated allelopathic effects of kudzu (Pueraria montana) on Bidens pilosa and Lolium perenne and its persistence in soil. Weed Biol Manage. 10:48–56. 10.1111/j.1445-6664.2010.00366.x
- Reigosa MJ, Sánchez-Moreiras A, González L. 1999. Ecophysiological approach in allelopathy. Crit Rev Plant Sci. 18:577–608. 10.1016/S0735-2689(99)00392-5
- Rice EL. 1984. Allelopathy. 2nd ed.Orlando, FL: Academic Press.
- Rudrappa T, Bonsall J, Gallagher JL, Seliskar DM, Bais HP. 2007. Root-secreted allelochemical in the noxious weed Phragmites australis deploys a reactive oxygen species response and microtubule assembly disruption to execute rhizotoxicity. J Chem Ecol. 33:1898–1918. 10.1007/s10886-007-9353-7
- Rudrappa T, Seok Choi Y, Levia DF, Legates DR, Lee KH, Bais HP. 2009. Phragmites australis root secreted phytotoxin undergoes photo-degradation to execute severe phytotoxicity. Plant Signal Behav. 4:6:506–513. 10.4161/psb.4.6.8698
- Saltonstall K, Burdick D, Miller SBS. 2005. Native and Introduced Phragmites: Challenges in Identification, Research, and Management of the Common Reed. National Estuarine Research Reserve Technical Report Series. Greenland (NH): National Estuarine Research Reserve System.
- Sengar R, Srivastava H. 1995. Effect of DTNB on glutamate dehydrogenase activity in root and shoot extracts of maize seedlings. Biol Plant. 37:147–151. 10.1007/BF02913011
- Singh HP, Batish DR, Kaur S, Arora K, Kohli RK. 2006. A Pinene inhibits growth and induces oxidative stress in roots. Ann Bot. 98:1261–1269. 10.1093/aob/mcl213
- Singh HP, Batish DR, Kaur S, Ramezani H, Kohli RK. 2002. Comparative phytotoxicity of four monoterpenes against Cassia occidentalis. Ann Appl Biol. 141:111–116. 10.1111/j.1744-7348.2002.tb00202.x
- Sinha S, Saxena R. 2006. Effect of iron on lipid peroxidation, and enzymatic and non-enzymatic antioxidants and bacoside-A content in medicinal plant Bacopa monnieri L. Chemosphere. 62:1340–1350. 10.1016/j.chemosphere.2005.07.030
- Stowe LG. 1979. Allelopathy and its influence on the distribution of plants in an illinois old-field. J Ecol. 67:1065–1085. 10.2307/2259228
- Tawaha A, Turk M. 2003. Allelopathic effects of black mustard (Brassica nigra) on germination and growth of wild barley (Hordeum spontaneum). J Agron Crop Sci. 189:298–303. 10.1046/j.1439-037X.2003.00047.x
- Uddin M, Caridi D, Robinson R. 2012. Phytotoxic evaluation of Phragmites australis: an investigation of aqueous extracts of different organs. Mar Freshwat Res. 63:777–787. 10.1071/MF12071
- Velikova V, Yordanov I, Edreva A. 2000. Oxidative stress and some antioxidant systems in acid rain-treated bean plants: protective role of exogenous polyamines. Plant Sci. 151:59–66. 10.1016/S0168-9452(99)00197-1
- Wardle D, Nicholson K, Ahmed M. 1992. Comparison of osmotic and allelopathic effects of grass leaf extracts on grass seed germination and radicle elongation. Plant Soil. 140:315–319. 10.1007/BF00010609
- Weidenhamer JD, Li M, Allman J, Bergosh RG, Posner M. 2013. Evidence does not support a role for gallic acid in Phragmites australis invasion success. J Chem Ecol. 39:323–332.
- Weir TL, Park S-W, Vivanco JM. 2004. Biochemical and physiological mechanisms mediated by allelochemicals. Curr Opin Plant Biol. 7:472–479. 10.1016/j.pbi.2004.05.007
- Yaber Grass MA, Leicach SR. 2011. Changes in Senecio grisebachii pyrrolizidine alkaloids abundances and profiles as response to soil quality. J Plant Interact. 7:175–182.