Abstract
A hydroponics experiment was conducted to test the effects of sodium nitroprusside (SNP, a donor of NO) supplied with different concentrations on copper (Cu) toxicity in ryegrass seedlings (Lolium perenne L.). Excess Cu (200 µM) reduced chlorophyll content, resulting a decrease in photosynthesis. Cu stress induced the production of hydrogen peroxide (H2O2) and superoxide anion (O2• −), leading to malondialdehyde (MDA) accumulation. Furthermore, activities of antioxidant enzymes in Cu-treated seedlings such as superoxide dismutase (SOD), peroxidase (POD), and catalase (CAT) were decreased. In addition, Cu stress inhibited the uptake of K, Mg, Fe, and Zn and increased Ca content in roots. Moreover, in leaves of Cu-stressed seedlings, K, Fe, and Zn contents were decreased and the contents of Ca and Mg were not affected significantly. In Cu-treated seedlings, Cu concentration in roots was higher than in leaves. Addition of 50, 100, 200 µM SNP in Cu-mediated solutions increased chlorophyll content and photosynthesis, improved antioxidant enzyme activities, reduced Cu-induced oxidative damages, kept intracellular ion equilibrium under Cu stress, increased Cu concentration in roots and inhibited Cu accumulation in leaves. In particular, addition of 100 µM SNP had the best effect on promoting growth of ryegrass seedlings under Cu stress. However, the application of 400 µM SNP had no obvious alleviating effect on Cu toxicity in ryegrass seedlings.
Abbreviations | ||
CAT, | = | catalase; |
Cu, | = | copper; |
H2O2, | = | hydrogen peroxide; |
MDA, | = | malondialdehyde; |
NO, | = | nitric oxide; |
O2•−, | = | superoxide anion; |
POD, | = | peroxidase; |
ROS, | = | reactive oxygen species; |
SNP, | = | sodium nitroprusside; |
SOD, | = | superoxide dismutase. |
1. Introduction
Copper (Cu) is an essential micro nutrient element of plants (Losak et al. Citation2011), playing an important role in a number of physiological processes such as the photosynthetic and respiratory electron transport chains (Van Assche & Clijsters Citation1990) and as a cofactor or as a part of the prosthetic group of many key enzymes involved in different metabolic pathways, including adenosine triphosphate (ATP) synthesis (Harrison et al. Citation1999). However, as a kind of heavy metal, Cu is toxic even at very low concentration. Recently, Cu has become increasingly hazardous to plants due to the applications of fungicides, pesticides, fertilizers, animal dung, and so on (Kaplan Citation1999; Brahim & Mohamed Citation2011).
In plants, slightly higher level of Cu than optimal will be toxic, including inhibition of seed germination, disruption of nutrient uptake (Fernandes & Henriques Citation1991), reduction of chlorophyll contents, inhibition of some photosynthetic functions in leaves (Kevresan et al. Citation2001), destruction of the lipid and protein constituent in membrane (Maksymiec Citation1997), and damage on the functions of plasma membrane and tonoplast (Zhang et al. Citation2009). As an active redox metal, Cu is able to induce the overproduction of reactive oxygen species (ROS), such as hydrogen peroxide (H2O2), hydroxyl radical (•OH), and superoxide anion (O2• −) directly, which in turn lead to lipid peroxidation and oxidative stress (Zhang et al. Citation2009; Cui et al. Citation2010). The excess formation of ROS can oxidize various cellular components, resulting in lipid peroxidation, membrane leakage, and enzyme inactivation, which can finally lead to oxidative injury and alteration in cell structure (Romero-Puertas et al. Citation2007). To survive against oxidative stress, plants have evolved protective mechanisms of mitigating and repairing the ROS damages (Xu et al. Citation2010). These are specific but complex mechanisms involving morphological changes, physiological and biochemical adaptations, and so on. Mainly, the ROS-scavenging mechanisms are made by enzymes such as peroxidase (POD), superoxide dismutase (SOD), catalase (CAT), ascorbate peroxidase (APX) and glutathione reductase (GR), and non-enzymatic system consisting of glutathione (GSH) and ascorbic acid (Xu et al. Citation2010). Besides these, to counteract the toxicity of copper, plants have developed various strategies by exudation of organic acid, retention of copper in roots and immobilization in the cell wall (Hu et al. Citation2007).
Nitric oxide (NO) is involved in the regulation of multiple responses to a variety of abiotic and biotic stresses in plants (Xiong et al. Citation2009). Recently, an increasing number of articles has reported the effects of exogenous NO on alleviating heavy metals, such as copper (Cu), nickel (Ni), aluminum (Al), and cadmium (Cd) (Wang & Yang Citation2005; Zhang et al. Citation2009; Mihailovic & Drazic Citation2011; Wang et al. Citation2013a). Furthermore, exogenous NO was described as an effective substance for alleviation of Cu toxicity in many plants, such as rice, tomato seedlings, wheat seeds, and so on (Yu et al. Citation2005; Hu et al. Citation2007; Zhang et al. Citation2009; Cui et al. Citation2010;). Various mechanisms were reported to contribute to NO-induced increase of Cu tolerance. Zhang et al. (Citation2009) detected that exogenous NO alleviated Cu toxicity by enhancing antioxidative system and increasing ATPase activities. Tewari et al. (Citation2008) reported that NO alleviates Cu toxicity by decreasing oxidative stress in the adventitious roots of Panax ginseng. In addition, the cytoprotective role for NO in plants has clearly been tested with DNA, lipids, proteins, and chlorophyll (Lamattina et al. Citation2003). However, NO is a reactive nitrogen species, and many studies have demonstrated that its effects on different cells are either protective or toxic, depending on its concentration and the position of action (Lamattina et al. Citation2003). Sodium nitroprusside (SNP) is often used as a NO donor in many studies. Its chemical reactions are mainly associated with the NO ligand (Coppens et al. Citation2002). In our previous study, it is indicated that the low concentrations of SNP alleviated Cd toxicity (Wang et al. Citation2013a), and the application of NO donor in field could also play roles in alleviating heavy toxicity (Xu et al. Citation2013a, Citation2013b). In this study, we investigated the effects of exogenous NO supplied with different concentrations on Cu toxicity and searched a proper SNP concentration to alleviate Cu stress in hydroponics.
As an important cool-season turf grass, perennial ryegrass can adapt well to temperate climates (Altpeter et al. Citation2000). It is commonly used for livestock, fiber products, improving soil contaminated with heavy metal, habitats for wildlife populations, recreation, and beautification (Beard Citation2002). Interestingly, there is an increasing evidence showing that perennial ryegrass (Lolium perenne L.) may play an important role in improving heavy metal contaminated soil (Arienzo et al. Citation2004; Kechavarzi et al. Citation2007). Wang et al. (Citation2013a) reported that most Cd accumulated in the roots of perennial ryegrass, and the application of exogenous NO inhibited Cd translocation from roots to shoots. Therefore, in this study, we investigated Cu toxicity on the growth of perennial ryegrass and the effects of exogenous NO on alleviating Cu toxicity. It is hypothesized that a proper concentration of NO could ameliorate Cu toxicity on perennial ryegrass and decrease Cu accumulation in the shoots.
2. Materials and methods
2.1. Plant material and culture conditions
Ryegrass seeds were sterilized with 5% sodium hypochlorite for 15 min and washed extensively with distilled water, then germinated on moist filter paper in the dark at 26°C for three days. Initially, seedlings of uniform size were transferred to plastic pots (volume 500 mL) filled with perlite (50 plants per pot) and watered with half-strength Hoagland nutrition solution for seven days. The seedlings were then watered with full-strength Hoagland solution. Three-week-old uniform seedlings were transferred into 1000 mL black plastic containers with 20 seedlings per container. The nutrient solution was renewed every two days.
The experimental design is given in . The treatments were arranged in a randomized block design with three replicates, giving a total of 18 containers.
Table 1. The experimental design of different concentrations SNP on the ryegrass under copper stress.
The experiment was carried out under a controlled environment chamber at 14/10 light/dark photoperiod and photon flux density 150 µmol m−2 s−1 at the leaf level, day/night temperature of 25/18°C and 65 ± 5% relative humidity. After two weeks of growth with the above conditions, the plants were harvested and the roots and shoots were separated and washed with 5 mM CaCl2 first and then repeatedly washed with deionized distilled water. For the estimation of plant dry matter, Cd and mineral nutrients content, the plants were dried at 80°C for 48 h. For the enzyme determination, fresh plant material was frozen in liquid nitrogen and stored at –70°C until use.
2.2. Determination of chlorophyll content
The chlorophyll content was determined according to the method of Knudson et al. (Citation1997). Fresh ryegrass leaf (0.5 g) was extracted in 2 mL 95% ethanol for 24 h in the dark, and the extracted solution was analyzed. The amounts of chlorophyll a, b, and carotenoid were determined spectrophotometrically (SHIMADZU UV-2450, Kyoto, Japan), by reading the absorbance at 665, 649, and 470 nm. The chlorophyll content results are expressed as unit's mg per gram-fresh weight (mg g−1 FW).
2.3. Determination of plant growth, cadmium, and mineral element concentrations
At harvest, the roots and shoots were separated and oven dried for 30 min at 105°C, then at 70°C till the materials reach their constant weights. The dried tissues were weighed and grinded into powder for the determination of cadmium and mineral element concentrations, which was measured by flame atomic absorbance spectrometry (SHIMADZU AA-6300, Kyoto, Japan) after digested with mixed acid [HNO3/HClO4 (3:1, v/v)] (Wang et al. Citation2013a).
2.4. Determination of the superoxide anion (O2• −) generation rate
The production rate of O2• − was measured as described by Elstner and Heupel (Citation1976). Fresh leaf samples (0.2 g) were homogenized in 1 mL of 50 mM phosphate buffer (pH 7.8), and the homogenate was centrifuged at 10,000 ×g for 10 min. Then 0.5 mL of the supernatant was added to 0.5 mL, 50 mM phosphate buffer (pH 7.8), and 0.1 mL of 10 mM hydroxylamine hydrochloride. After 1 h reaction at 25°C, the mixture was added to 1 mL of 17 mM sulfanilamide, and 1 mL of 7 mM α-nahthylamine at 25°C for 20 min. The specific absorbance at 530 nm was determined. Sodium nitrite was used as a standard solution to calculate the production rate of O2• −.
2.5. Hydrogen peroxide (H2O2) determination
For determination H2O2 concentration, leaf tissue (0.2 g) was extracted with 3 mL of 0.1% (w/v) trichloroacetic acid (TCA) in an ice bath and centrifuged at 12,000 ×g for 15 min (Velikova et al. Citation2000). An aliquot (0.5 mL) of supernatant was added to 0.5 ml of phosphate buffer (pH 7.0) and 1 mL of 1 M KI. The absorbance of the mixture was read at 390 nm. H2O2 content was determined using the extinction coefficient 0.28 µM−1cm−1 and the amount expressed as µmol g−1 FW.
2.6. Determination of lipid peroxidation
Lipid peroxidation was determined by measuring malondialdehyde (MDA), a major thiobarbituric acid reactive species (TBARS), and product of lipid peroxidation (Heath & Packer Citation1968). Samples (0.2 g) are ground in 3 mL of trichloroacetic acid (0.1%, w/v). The homogenate was centrifuged at 10,000 ×g for 10 min and 1 mL of the supernatant fraction was mixed with 4 mL of 0.5% thiobarbituric acid (TBA) in 20% TCA. The mixture was heated at 95°C for 30 min, chilled on ice, and then centrifuged at 10,000 ×g for 5 min. The absorbance of the supernatant was measured at 532 nm. The value for non-specific absorption at 600 nm was subtracted. The amount of MDA was calculated using the extinction coefficient of 155 mM−1 cm−1 and expressed as nmol g−1 FW.
2.7. Determination of antioxidant enzymes
For extraction of antioxidative enzymes, leaves and roots were homogenized with 50 mM Na2HPO4-NaH2PO4 buffer (pH 7.8) containing 0.2 mM EDTA and 2% insoluble polyvinylpyrrolidone in a chilled pestle and mortar. The homogenate was centrifuged at 12,000 ×g for 20 min and the resulted supernatant was used for determining of enzyme activities. The whole extraction procedure was carried out at 4°C. All spectrophotometric analysis was conducted on a SHIMADZU UV-2450 spectrophotometer (Kyoto, Japan).
SOD activity was assayed by measuring its ability to inhibit the photochemical reduction of nitroblue tetrazolium following the method of Stewart and Bewley (Citation1980). CAT activity was measured as the decline in absorbance at 240 nm due to the decrease of extinction of H2O2 according to the method of Patra et al. (Citation1978). POD activity was measured by the increase in absorbance at 470 nm due to guaiacol oxidation (Nickel & Cunningham Citation1969). APX activity was measured by the decrease in absorbance at 290 nm as ascorbate was oxidized (Nakano & Asada Citation1981).
2.8. Statistical analysis
All data presented here are the mean values of three independent experiments with three replicates. Statistical analyzes were performed by analysis of variance (ANOVA) using the SAS software. Differences between treatments were separated by the least significant difference test at a 0.05 probability level.
3. Results
3.1. Plant growth
The effects of different concentration of SNP on plant growth, expressed as plant height, fresh weight, dry weight, root volume, and root/shoot ratio are shown in . Cu exposure inhibited the growth of ryegrass significantly compared with CK, and the reduction of plant height, fresh weight, dry weight, root volume, and root/shoot ratio was 34.76%, 17.51%, 54.38%, 28.13%, and 33.33%, respectively. However, this inhibition was alleviated by the additions of 50, 100, and 200 µM SNP, especially 100 µM SNP. Compared with Cu treatment, the addition of 100 µM SNP increased plant height, fresh weight, dry weight, root volume, and root/shoot ratio by 49.36%, 15.92%, 71.52%, 36.96%, and 44.44%. When applying 400 µM SNP into Cu-treated solution, Cu-induced inhibition on plant growth was not mitigated. Compared with CK, 400 µM SNP reduced plant height, fresh weight, dry weight, root volume, and root/shoot ratio by 30.82%, 18.21%, 52.27%, 28.65%, and 29.63%.
Table 2. Effects of different concentrations of SNP supply on the growth of ryegrass seedlings under copper stress.
3.2. Chlorophyll content
Ryegrass seedlings treated with Cu showed a significant decrease in total chlorophyll, chl a, chl b, and car as compared with CK (). The applications of different concentrations of SNP changed the chlorophyll contents. Compared with Cu treatment, additions of 50, 100, and 200 µM SNP increased chlorophyll contents, especially at 100 µM SNP. 100 µM SNP increased total chlorophyll, chl a, chl b, and car by 50.34%, 53.00%, 41.85%, and 118.05%, respectively. However, the addition of 400 µM SNP had no alleviated effects on Cu-decreased chlorophyll contents.
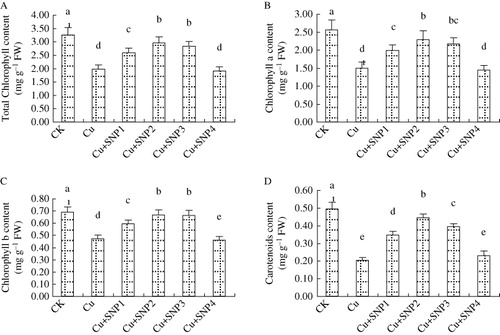
3.3. Photosynthesis and transpiration rates
In Cu-treated plants, photosynthesis and transpiration rates were decreased by 41.55% and 40.83% compared with CK (). The application of 100 µM SNP increased photosynthesis significantly, although additions of 50, 200, and 400 µM SNP had no effect on Cd-decreased photosynthesis. For the transpiration rate of ryegrass, 50, 100, and 200 µM SNP added in Cu-treated solution increased Cu-inhibited transpiration rate, especially at 100 µM SNP, and the treatment increased transpiration rate by 62.95% compared with Cu-treated plants. However, 400 µM SNP supply did not change Cu-decreased transpiration rate.
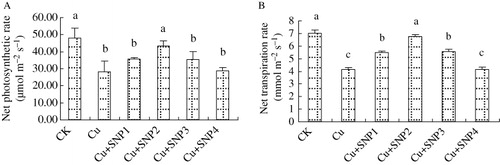
3.4. O2• − generation rate and H2O2 content
Compared with CK, Cu exposure alone significantly increased O2• − generation rate by 46.3%, 135.1% in leaves and roots, respectively (). Applications of exogenous NO had effects on O2• − generation rate in the leaves and roots. The additions of 50, 100, and 200 µM SNP inhibited Cu-induced O2• − generation rate significantly, especially at 100 µM SNP. As many as 400 µM SNP supplementation decreased Cu-increased O2• − generation rate in leaves, while it did not inhibit Cd-increased O2• − generation rate in roots.
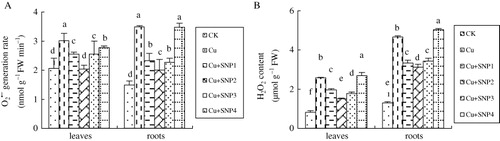
As shown in , compared with CK, Cu stress significantly increased H2O2 content by 216.40%, 261.91% in leaves and roots, respectively. Additions of 50, 100, and 200 µM SNP reduced Cu-caused H2O2 accumulation in leaves and roots, especially at 100 µM SNP. The addition of 100 µM SNP in the presence of Cu decreased H2O2 content by 41.97%, 33.07% in leaves and roots, compared with Cu treatment. However, 400 µM SNP added in Cu-treated solutions increased H2O2 content compared with Cu stress.
3.5. MDA content
MDA content was an index of oxidative damage induced by environmental stress (). In Cu-treated plants, MDA contents in leaves and roots were accumulated significantly, compared with CK. Additions of 50, 100, and 200 µM SNP inhibited Cu-caused MDA accumulation in leaves and roots, and the inhibition was more obvious with the addition of 100 µM SNP. Compared with Cu treatment, MDA contents in leaves and roots were decreased 35.22% and 36.89% in the presence of 100 µM SNP. However, 400 µM SNP supply did not reduce Cu-induced MDA accumulation in leaves and roots.
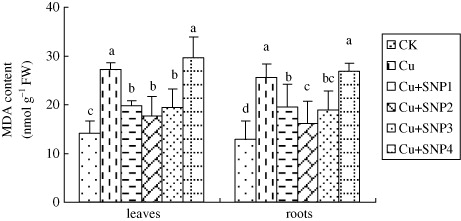
3.6. Antioxidant enzymes
As shown in , compares to CK, SOD activity in leaves and roots of Cu-treated plants were decreased by 49.50% and 53.02%. 50, 100, and 200 µM SNP added in Cd-treated solution increased Cu-inhibited SOD activity, in particular, 100 µM SNP increased it by 74.32% and 87.25% in leaves and roots respectively, compared with Cu-treated plants. However, the addition of 400 µM SNP did not increase Cd-decreased SOD activity.
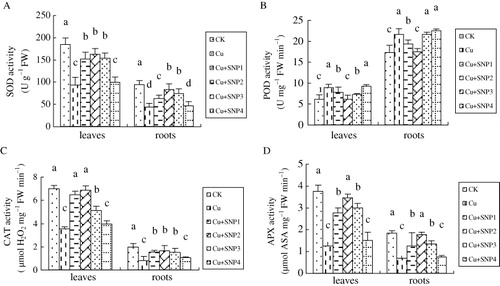
Cu-induced change of POD activity was different from SOD activity (). POD activity in leaves and roots of Cu-treated plants was increased significantly compared with CK. Cu-increased POD activity was decreased by the additions of 50, 100, and 200 µM SNP. The application of 400 µM SNP had no change on Cu-induced POD activity.
The changes of CAT and APX activities in leaves and roots in all treatments were similar to SOD activity ( and ). Cu stress inhibited the activities of CAT and APX in leaves and roots of ryegrass compared with CK. Additions of 50, 100, and 200 µM SNP alleviated Cu-decreased activities of CAT and APX, especially at 100 µM SNP. However, Cu-changed activities of CAT and APX were not affected by the addition of 400 µM SNP.
3.7. Mineral element contents
showed mineral element contents in shoots and roots of ryegrass. In shoots, compared with CK, Cu exposure decreased K, Mg, Fe, and Zn by 62.69%, 14.98%, 36.79%, and 27.13%, while Ca content was increased by 100.00%. Applications of 50, 100, and 200 µM SNP improved contents of K, Mg, Fe, and Zn and decreased Ca content, compared with Cu treatment, especially at 100 µM SNP. The addition of 100 µM SNP increased K, Mg, Fe, and Zn content by 122.48%, 16.27%, 65.05%, and 42.57% and decreased Ca content by 39.63%. Compares with Cu-treated plants, the addition of 400 µM SNP increased contents of Ca and Fe, but it had no effect on contents of K, Mg, and Zn.
In roots, Cu stress inhibited the uptake of K, Fe, and Zn significantly and had no obvious effect on Ca and Mg uptake. Compared with Cu treatment, the additions of 50, 100, 200, and 400 µM SNP reduced K uptake, especially at 100 µM SNP. Applications of 50, 100, and 200 µM SNP inhibited Cu-increased Ca content, while the application of 400 µM SNP had no effect on Cu-induced uptake of Ca. For the uptake of Mg, Fe and Zn, SNP supplementation increased Cu-inhibited absorption, especially at 100 µM SNP.
3.8. Cu concentration
As shown in , Cu exposure increased Cu concentration in roots and leaves of ryegrass significantly and most Cu was located in roots. In roots, compared with Cu treatment, 50, 100, and 200 µM SNP added in Cu-treated solutions improved Cu content, while the application 400 µM SNP decreased Cu accumulation. In shoots, additions of 50, 100, 200, and 400 µM SNP reduced Cu accumulation compared with Cu-stressed plants, especially at 100 µM SNP, and the reduction induced by 100 µM SNP was 47.05%.
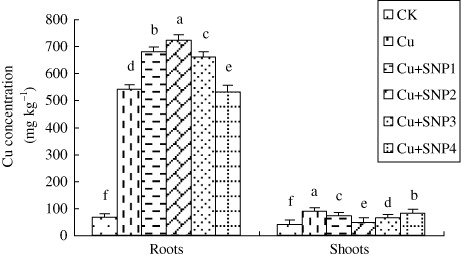
4. Discussion
As a crucial gaseous signaling molecule in plants, NO plays significant roles in regulating many key physiological processes (Besson-Bard et al. Citation2008). In recent studies, NO has been established as a key messenger molecule in the resistant responses in plants against heavy metals, including arsenic (Singh et al. Citation2009), cadmium (Wang et al. Citation2013a), and copper (Zhang et al. Citation2009). According to Qiao and Fan (Citation2008), NO can provoke both beneficial and harmful effects depending on the concentration and location of NO in plant cells. This type of dual function of NO as a potent oxidant or effective antioxidant generally depends on the status of the environments (Beligni & Lamattina Citation1999). Our previous study reported that the alleviation effects of NO on Cu stress in ryegrass needed a proper concentration and the lower concentration of NO had a higher protective effect on Cd toxicity (Wang et al. Citation2013a). In this experiment, different SNP concentrations were applied in Cu-treated growth medium. We investigated the physiological characteristics of ryegrass under Cu stress with applying different SNP concentration, aiming at searching for a proper concentration of NO on alleviating Cu toxicity effectively.
Cu-induced growth inhibition was reflected by the decrease in plant growth, fresh weight, dry weight, root volume, and root/shoot ratio (). Inhibition of growth in ryegrass plants might be the result of Cu-caused alteration of fundamental metabolic processes, for example, oxidative damage, nutrient uptake and photosynthesis. Similar results were reported by Maksymiec et al. (Citation1995) on runner plants and Sheldon and Menzies (Citation2005) about Rhodes grass. However, Cu-induced inhibition was significantly alleviated by the lower concentrations of NO. The mitigation effect of lower concentration of NO might be that NO induced the improvement of in photosynthesis by increasing chlorophyll content ( and ), counteracted oxidative damage by decreasing the generation of ROS () and increased the uptake of nutrient elements (). When the higher NO concentration was applied, the mitigation effect was not obvious. The results demonstrated the nature of NO on plants under Cu stress was dual, which was consistent with the reports of Tu et al. (Citation2003) and Wang et al. (Citation2013a).
Table 3. Effects of different concentrations of SNP supply on mineral elements (Fe, Mn, Zn) and Cu contents in ryegrass under copper stress.
Typical symptoms of Cu toxicity were yellowing and drying on the leaves of plants (Brahim & Mohamed Citation2011). The reduction of chlorophyll content was detected in ryegrass under Cu stress in this study (), which was accompanied by the decrease in photosynthesis and transpiration (). The decline in chlorophyll content is believed to be due to: (1) inhibition of enzymes associated with chlorophyll biosynthesis (John et al. Citation2009); (2) inhibition of uptake and transportation of other metal elements such as Mn, Zn, and Fe by antagonistic effects (Jayakumar et al. Citation2009; John et al. Citation2009). Similar decrease in chlorophyll content under Cu stress was reported in Atriplex halimus (Brahim & Mohamed Citation2011) and mangrove plant seedlings (Zhang et al. Citation2007). In this study, it was demonstrated that the application of exogenous NO at lower concentration alleviated Cu-induced decrease in chlorophyll content. The enhancement of uptake and transportation of metal elements such as Mg, Zn, and Fe () might contribute to chlorophyll synthesis. In addition, Chen et al. (Citation2010) detected that NO-mediated stability and integrity of the subcellular structure under Cd stress contributed to its effective role in preventing Cd-induced leaf chlorosis and inhibition of photosynthesis in barely seedlings. Our results indicated that NO-mediated recovery of chlorophyll contents played a role in the enhancement of photosynthesis and transpiration in ryegrass, which might be responsible for the increase of Cu tolerance.
As an active redox metal, Cu is able to induce the overproduction of ROS, such as hydrogen peroxide (H2O2), hydroxyl radical (•OH), and superoxide anion (O2• −) directly, which in turn lead to lipid peroxidation and oxidative stress (Polle & Schützendübel Citation2004; Zhang et al. Citation2009). We investigated the involvement of these molecules, such as O2• −, H2O2, and MDA in this experiment. Results of this study also showed that Cu induced over accumulation of O2• −, H2O2, and MDA in leaves and roots (), leading to the oxidative injury in ryegrass and inhibiting plant growth. Cu-caused oxidative damage was demonstrated by Zhang et al. (Citation2009) and Vantová et al. (Citation2013). However, addition of NO alleviated H2O2 and O2• − under Cu stress, and the influence protected cell membrane from peroxiding and decreased the accumulation of MDA (). Many studies reported that NO inhibited the plant from oxidation damage by regulating general mechanisms for cellular redox homeostasis and promoting the transformation of O2• − to H2O and O2 and also by enhancing the H2O2-scavenging enzyme activities (Lamattina et al. Citation2003). In this experiment, results demonstrated that exogenous NO improved the Cu-inhibition on SOD, POD, CAT, and APX activities (), which played a role in alleviating Cu-induced oxidative damage. Two mechanisms which may explain NO protective action against oxidative damage have been widely reported. Firstly, NO might detoxify ROS directly, such as superoxide anion, to form peroxyntrite, which is less toxic and thus limit cellular damage (Martinez et al. Citation2000). Secondly, NO could function as a signaling molecule, which activates the cellular antioxidant system (Lamattina et al. Citation2003). However, the effects of NO depend on its location and concentration. Beneficial reactions counteract oxidative and nitrosative stresses, while damaging reactions, due to high levels of NO, cause oxidative and nitrosative damage and cell wall (Siddiqui et al. Citation2011). In the present study, the application of high NO concentration did not reduce Cu-induced ROS damage, even produced more toxic effects in ryegrass seedlings.
To cope with ROS and alleviate their toxic effects, plants possess several antioxidative systems. In the present experiment, activities of SOD, CAT, and APX in ryegrass leaves and roots were decreased under Cu stress, while POD activity was increased. This result indicated that POD induction might play an important role in ryegrass tolerance to Cu stress. There are different reports on activities of these enzymes under Cu stress (Zhang et al. Citation2009; Brahim & Mohamed Citation2011). The difference indicated that the influence of Cu stress on the antioxidant enzymes was very complex and related to the plant treatment time, plant tissues, plant species, and genotypes. It was interesting that activities of scavenging enzymes (SOD, POD, CAT, and APX) were higher in NO-treated seedlings than Cu-stressed seedlings (). It has been known that antioxidant system plays an important role in plant tolerance to stress conditions, which is based on the fact that the activity of one or more of these enzymes or antioxidant substances in general increase in plants when exposed to stressful condition and these enhances are related to increased stress tolerance (Fecht-Christoffers et al. Citation2003). Lamattina et al. (Citation2003) reported that NO might regulate the expression of antioxidative genes to stimulate the relative enzyme activities. NO-mediated increase in one or more of these antioxidant enzymes contributed to the enhancement of antioxidative ability. Such results suggested that the protective effects of NO on oxidative damage were partly related to its role in up-regulating antioxidative ability. However, the increase in enzyme activities was less by high level of NO, as a result that the alleviation of NO on ROS damage was not obvious.
Ion balance inside the cell is closely related to plant adaptation to environmental stress (CitationWang et al. 2013b). In this study, excess Cu led to a deficiency of K, Mg, Fe, and Zn in shoots and K, Fe, and Zn in roots (), which may be a cause and a consequence of growth inhibition. However, exogenous NO promoted the uptake and translocation of these mineral elements under Cu stress (). K+ is an essential element that plays vital roles in various aspects of plant cell growth and metabolism and is needed in large quantities (Zhu et al. Citation1998). NO-increased absorption of K might play an important role in promoting the growth of ryegrass. Mg is an essential element for chlorophyll biosynthesis, and NO-promoted content of Mg in shoots might account for the increase of chlorophyll content. NO has high affinity toward the Fe-containing active sites of many proteins and thus participates in the regulation of Fe transport in plants (Ramirez et al. Citation2010), as a result that Fe content was increased by the addition of NO. It is well known that Zn is required for the synthesis of auxin (IAA), and exogenous NO promoted Zn uptake, which might account for the promotion of plant growth under Cu stress. Notable, Cu stress increased Ca content in shoots and did not affect it in roots. The reason might be that a mechanism of maintaining ion balance in plant existed. The change of exogenous NO on Ca content in shoots and roots might play a vital role in mediating intracellular ion equilibrium under Cu stress.
In this study, a majority of Cu accumulated in roots, while a small number was located in shoots (). Plant root is a tissue contacting with heavy-metal ions in soil directly. Many studies reported that a majority of heavy-metal ions was accumulated in roots (Hattab et al. Citation2009; Wang Citation2013a). Addition of low concentration of NO increased Cu accumulation in roots and decreased its content in shoots effectively (), which indicated that NO inhibited excess Cu transferred to shoots. Xiong et al. (Citation2009) pointed out that low accumulation of heavy metal in the aboveground parts of plants is the principal defense to counteract its toxicity. Fecht-Christoffers et al. (Citation2003) indicated that NO, as a recognized regulator of protein activation by S-nitrosylation, might inactivate heavy mental by modification of phytochelatins or some other ligands containing SH-group. Therefore, NO-induced low Cu concentration in ryegrass leaves contributed to the increase of Cu tolerance.
5. Conclusions
Our results demonstrated that in the hydroponics experiment, the low concentrations of NO provided the resistance toward Cu and had an ameliorating effect on Cu-stressed ryegrass, while high concentration of NO did not alleviate Cu toxicity effectively. Our study proved that exogenous NO at low concentrations increased Cu tolerance in ryegrass grown in Cu-mediated nutrient solution by (1) increasing chlorophyll content and photosynthesis, (2) maintaining intracellular ion equilibrium under Cu stress and decreased Cu translocation from roots to shoots, and (3) improving antioxidant enzyme activities and protecting against Cu-induced oxidative stress. The results may have a potential value on repairing heavy metal contamination and increasing grass production.
Acknowledgments
The authors thank English Lecturer Mr Stuart Craig MA (England, Taishan University of china), and lecturer Xiujuan Wang (College of Foreign Languages, Shandong Agricultural University) for their critical reading and revision of the manuscript. Great thanks were given to Pingping Yang, College of Animal Science Technology, Shandong Agricultural University, China, for her supplying instruments and patient guidance. Special acknowledgments are given to the editors and reviewers.
References
- Altpeter F, Xu JP, Ahmed S. 2000. Generation of large number of independently transformed fertile perennial ryegrass (Lolium perenne L.) plants of forage and turf-type cultivars. Mol Breed. 6:519–528. doi:10.1023/A:1026589804034
- Arienzo M, Adamo P, Cozzolino V. 2004. The potential of Lolium perenne for revegetation of contaminated soil from a metallurgical site. Sci Total Environ. 319:13–25. doi:10.1016/S0048-9697(03)00435-2
- Beard JB. 2002. Turfgrass management for golf courses. 2nd ed. Hoboken: Wiley; p. 793.
- Beligni MV, Lamattina L. 1999. Nitric oxide counteracts cytotoxic processes mediated by reactive oxygen species in plant tissues. Planta. 208:337–344. doi:10.1007/s004250050567
- Besson-Bard A, Pugin A, Wendehenne D. 2008. New insights into nitric oxide signaling in plants. Annu Rev Plant Biol. 59:21–39. doi:10.1146/annurev.arplant.59.032607.092830
- Brahim L, Mohamed M. 2011. Effects of copper stress on antioxidative enzymes, chlorophyll and protein content in Atriplex halimus. Afr J Biotechnol. 50:10143–10148.
- Chen F, Wang F, Sun HY, Cai Y, Mao WH, Zhang GP, Vincze E, Wu FB. 2010. Genotype-dependent effect of exogenous nitric oxide on Cd-induced changes in antioxidative metabolism, ultrastructure, and photosynthetic performance in barley seedlings (Hordeum vulgare). J Plant Growth Regul. 29:394–408. doi:10.1007/s00344-010-9151-2
- Coppens P, Novozhilova I, Kovalevsky A. 2002. Photoinduced linkage isomers of transition-metal nitrosyl compounds and related complexes. Chem Rev. 102:861–883. doi:10.1021/cr000031c
- Cui XM, Zhang YK, Wu XB, Liu CS. 2010. The investigation of the alleviated effect of copper toxicity by exogenous nitric oxide in tomato plants. Plant Soil Environ. 56:274–281.
- Elstner EF, Heupel A. 1976. Inhibition of nitrite formation from hydroxylammoniumchloride: a simple assay for superoxide dismutase. Anal Biochem. 70:616–620. doi:10.1016/0003-2697(76)90488-7
- Fecht-Christoffers MM, Braun HP, Lemaitre-Guillier C, van Dorsselaer A, Horst WJ. 2003. Effect of manganese toxicity on the proteome of the leaf apoplast in cowpea. Plant Physiol. 133: 1935–1946. doi:10.1104/pp.103.029215
- Fernandes JC, Henriques FS. 1991. Biochemical, physiological, and structural effects of excess copper in plants. Bot Rev. 57:246–273. doi:10.1007/BF02858564
- Harrison MD, Jones CE, Dameron CT. 1999. Copper chaperones: function structure and copper-binding properties. JBIC. 4:145–153. doi:10.1007/s007750050297
- Hattab S, Chouba L, Benkheder M, Mahouachi T, Boussetta H. 2009. Cadmium- and copper-induced DNA damage in Pisum sativum roots and leaves as determined by the Comet assay. Plant Biosyst. 143:6–11. doi:10.1080/11263500903187035
- Heath RL, Packer L. 1968. Photoperoxidation in isolated chloroplasts: I. Kinetics and stoichiometry of fatty acid peroxidation. Arch Biochem Biophys. 125:189–198. doi:10.1016/0003-9861(68)90654-1
- Hu KD, Hu LY, Li YH, Zhang FQ, Zhang H. 2007. Protective roles of nitric oxide on germination and antioxidant metabolism in wheat seeds under copper stress. Plant Growth Regul. 53:173–183. doi:10.1007/s10725-007-9216-9
- Jayakumar K, Abdul Jaleel C, Vijayarengan P. 2009. Effect of different concentrations of cobalt on pigment contents of soybean. Bot Res Int. 2:153–156.
- John R, Ahmad P, Gadgil K, Sharma S. 2009. Heavy metal toxicity: Effect on plant growth, biochemical parameters and metal accumulation by Brassica juncea L. Int J Plant Prod. 3:65–76.
- Kaplan M. 1999. Accumulation of Cu in soils and leaves of tomato plants in greenhouses in Turkey. J Plant Nutr. 22:237–244. doi:10.1080/01904169909365622
- Kechavarzi C, Pettersson K, Leeds-Harrison P, Ritchie L, Ledin S. 2007. Root establishment of perennial ryegrass (L. perenne) in diesel contaminated subsurface soil layers. Environ Pollut. 145:68–74. doi:10.1016/j.envpol.2006.03.039
- Kevresan S, Petrovic N, Popovic M, Kandrac J. 2001. Nitrogen and protein metabolism in young pea plants as affected by different concentrations of nickel, cadmium, lead, and molybdenum. J Plant Nutr. 24:1633–1644. doi:10.1081/PLN-100106026
- Knudson LL, Tibbitts TW, Edwards GE. 1997. Measurement of ozone injury by determination of leaf chlorophyll concentration. Plant Physiol. 60:606–608. doi:10.1104/pp.60.4.606
- Lamattina L, Garcia-Mata C, Graziano M, Pagnussat G. 2003. Nitric oxide: the versatility of an extensive signal molecule. Annu Rev Plant Biol. 54:109–136. doi:10.1146/annurev.arplant.54.031902.134752
- Losak T, Hlusek J, Martinec J, Jandák J, Szostkova M, Filipcík R, Manásek J, Prokes K, Peterka J, Varga L, et al. 2011. Nitrogen fertilization does not affect micronutrient uptake in grain maize (Zea mays L.). Acta Agric Scand Sect B – SP. 61:543–550.
- Maksymiec W. 1997. Effect of copper on cellular processes in higher plants. Photosynthetica. 34:321–342. doi:10.1023/A:1006818815528
- Maksymiec W, Bednara J, Baszynski T. 1995. Responses of runner plants to excess copper as a function of plant growth stages: effects on morphology and structure of primary leaves and their chloroplast ultrastructure. Photosynthetica. 31:427–435.
- Martinez GR, Mascio PD, Bonini MG, Augusto O, Briviba K, Sies H. 2000. Peroxynitrite does not decompose to singlet oxygen (1gO2) and nitroxyl (NO-). Proc Natl Acad Sci. 97:10307–10312. doi:10.1073/pnas.190256897
- Mihailovic N, Drazic G. 2011. Incomplete alleviation of nickel toxicity in bean by nitric oxide supplementation. Plant Soil Environ. 57:396–401. doi:10.1080/00380768.2011.582588
- Nakano Y, Asada K. 1981. Hydrogen peroxide scanvenged by ascorbated specific peroxidase in spinach chloroplast. Plant Cell Physiol. 22:867–880.
- Nickel RS, Cunningham BA. 1969. Improved peroxidase assay method using Ieuco 2, 3, 6-trichlcroindophenol and application to comparative measurements of peroxidase catalysis. Anal Biochem. 27:292–299. doi:10.1016/0003-2697(69)90035-9
- Patra HL, Kar M, Mishre D. 1978. Catalase activity in leaves and cotyledons during plant development and senescence. Biochem Pharmacol. 172:385–390.
- Polle A, Schützendübel A. 2004. Heavy metal signalling in plants: linking cellular and organismic responses. In: Hirt H, Schinozaki K, editors. Plant Responses to Abiotic Stress. Berlin, Heidelberg: Springer–Verlag; p. 187–216.
- Qiao W, Fan LM. 2008. Nitric oxide signaling in plant responses to abiotic stresses. J Integr Plant Biol. 50:1238–1246. doi:10.1111/j.1744-7909.2008.00759.x
- Ramirez L, Zabaleta EJ, Lamattina L. 2010. Nitric oxide and frataxin: two players contributing to maintain cellular iron homeostasis. Ann Bot. 46:415–422.
- Romero-Puertas MC, Laxa M, Matte A, Zaninotto F, Finkemeier I, Jones AM, Perazzolli M, Vandelle E, Dietz KJ, Delledonne M. 2007. S-Nitrosylation of peroxiredoxin II E promotes peroxynitrite-mediated tyrosine nitration. Plant Cell. 19:4120–4130. doi:10.1105/tpc.107.055061
- Sheldon AR, Menzies NW. 2005. The effect of copper toxicity on the growth and root morphology of Rhodes grass (Chloris gayana Knuth.) in resin buffered solution culture. Plant Soil. 278:341–349. doi:10.1007/s11104-005-8815-3
- Siddiqui MH, Al-Whaibi MH, Basalah MO. 2011. Role of nitric oxide in tolerance of plants to abiotic stress. Protoplasma. 248:447–455. doi:10.1007/s00709-010-0206-9
- Singh HP, Kaur S, Batish DR, Sharma VP, Sharma N, Kohli PK. 2009. Nitric oxide alleviates arsenic toxicity by reducing oxidative damage in the roots of Oryza sativa (rice). Nitric Oxide. 20:289–297. doi:10.1016/j.niox.2009.02.004
- Stewart RC, Bewley JD. 1980. Lipid peroxidation associated with accelerated aging of soybean axes. Plant Physiol. 65:245–248. doi:10.1104/pp.65.2.245
- Tewari RK, Hahn EJ, Paek KY. 2008. Modulation of copper toxicity-induced oxidative damage by nitric oxide supply in the adventitious roots of Panax ginseng. Plant Cell Rep. 27:171–181. doi:10.1007/s00299-007-0423-7
- Tu J, Shen WB, Xu LL. 2003. Regulation of nitric oxide on the aging process of wheat leaves. Acta Botanica Sinica. 45:1055–1062.
- Van Assche F, Clijsters H. 1990. Effects of metals on enzyme activity in plant. Plant Cell Environ. 13:195–206. doi:10.1111/j.1365-3040.1990.tb01304.x
- Vantová I, Bačkor M, Klejdus B, Bačkorová M, Kováčik J. 2013. Copper uptake and copper-induced physiological changes in the epiphytic lichen Evernia prunastri. Plant Growth Regul. 69:1–9. doi:10.1007/s10725-012-9741-z
- Velikova V, Yordanov I, Edreva A. 2000. Oxidative stress and some antioxidant systems in acid rain-treated bean plants. Plant Sci. 151: 59–66. doi:10.1016/S0168-9452(99)00197-1
- Wang QH, Liang X, Dong YJ, Xu LL, Zhang XW, Hou J, Fan ZY. 2013a. Effects of exogenous nitric oxide on cadmium toxicity, element contents and antioxidative system in perennial ryegrass. Plant Growth Regul. 69:11–20. doi:10.1007/s10725-012-9742-y
- Wang QH, Liang X, Dong YJ, Xu LL, Zhang XW, Kong J, Liu S. 2013b. Effects of exogenous salicylic acid and nitric oxide on physiological characteristics of perennial ryegrass under cadmium stress. J Plant Growth Regul. doi:10.1007/s00344-013-9339-3
- Wang YS, Yang ZM. 2005. Nitric oxide reduces aluminum toxicity by preventing oxidative stress in the roots of Cassia tora L. Plant Cell Physiol. 46:1915–1923. doi:10.1093/pcp/pci202
- Xiong J, An L, Lu H, Yhu C. 2009. Exogenous nitric oxide enhances cadmium tolerance of rice by increasing pectin and hemicellulose contents in root cell wall. Planta. 230:755–765. doi:10.1007/s00425-009-0984-5
- Xu J, Wang WY, Yin HX, Liu XJ, Sun H, Mi Q. 2010. Exogenous nitric oxide improves antioxidative capacity and reduces auxin degradation in roots of Medicago truncatula seedlings under cadmium stress. Plant Soil. 326:321–330. doi:10.1007/s11104-009-0011-4
- Xu LL, Fan ZY, Dong YJ, Kong J, Liu S, Hou J, Bai XY. 2013a. Effects of exogenous NO supplied with different approaches on cadmium toxicity in lettuce seedlings. Plant Biosyst. Available from: http://dx.doi.org/10.1080/11263504.2013.822030
- Xu LL, Dong YJ, Fan ZY, Kong J, Liu S, Bai XY. 2013b. Effects of the application of exogenous NO at different growth stage on the physiological characteristics of peanut grown in Cd-contaminated soil. J Plant Interact. Available from: http://www.tandfonline.com/doi/full/10.1080/17429145.2013.830780
- Yu CC, Hung KT, Kao CH. 2005. Nitric oxide reduces Cu toxicity and Cu-induced NH4+ accumulation in rice leaves. J Plant Physiol. 162:1319–1330. doi:10.1016/j.jplph.2005.02.003
- Zhang FQ, Wang YS, Lou ZP, Dong GD. 2007. Effect of heavy metal stress on antioxidative enzymes and lipid peroxidation in leaves and roots of two mangrove plant seedlings (Kandelia candel and Bruguiera gymnorrhiza). Chemotherapy. 67:44–50.
- Zhang Y., Han X., Chen X., Jin H., Cui X. 2009. Exogenous nitric oxide on antioxidative system and ATPase activities from tomato seedlings under copper stress. Scientia Hortic. 123:217–223. doi:10.1016/j.scienta.2009.08.015
- Zhu JK, Liu JP, Xiong LM. 1998. Genetic analysis of salt tolerance in Arabidopsis: evidence for a critical role of potassium nutrition. Plant Cell. 10:1181–1191.