Abstract
The research aimed to verify the important physiological effect of nitrogen (N) on plants exposed to cadmium (Cd). The poplar plants were grown in a Hoagland nutrient solution and treated with extra N, Cd, and N + Cd. After treatment, plant growth and chlorophyll content were recorded. The oxidative stress, the activity of antioxidant enzymes, and the expression of related genes were also examined. The results indicated the plants treated with sole Cd presented obvious toxicity symptoms, i.e. growth inhibition, reactive oxygen species accumulation, and chlorophyll content decrement. However, when N was added to the plants under Cd stress, plant growth was enhanced, chlorophyll synthesis was promoted, and the oxidative stress was alleviated. Further, the expression of antioxidant enzymes genes was upregulated by N. The results indicated that N partially reversed the toxic effect of Cd on poplar plants, which can provide new methodology to enhance the phytoremediation technology for heavy metal pollution soil.
Introduction
Human development, such as industrialization and urbanization, has created serious environmental contamination problems. The increased amount of heavy metals in the environment poses a serious threat to the natural ecosystem by causing it to become unbalanced and deteriorated. Cadmium (Cd) is one of the most toxic pollutants found in the air, water, and soil, and is nonessential for plants. It can be accumulated through the food chain (Wagner Citation1993) and is a danger to animal and human health (Chaney et al. Citation1999; McLaughlin et al. Citation1999). Cd has a negative influence on photosynthetic, respiratory, and nitrogen (N) metabolism in plants, resulting in a poor growth and low biomass (Sanità di Toppi & Gabbrielli Citation1999; Pereira et al. Citation2002). Recently, Cd pollution has become more severe and has accelerated the environment deterioration, including the degradation of farmlands in the core polluted regions of the world (Brunetti et al. Citation2012).
Cd stress mainly stimulates the accumulation of reactive oxygen species (ROS) including superoxide radicals (O2 −), hydroxyl radicals (OH), and hydrogen peroxide (H2O2) in plants (Dietz et al. Citation1999; Sandalio et al. Citation2001; Romero-Puertas et al. Citation2004). An atom or group of atoms that has at least one unpaired electron is known as free radical, such as O2−, OH, H−, CH3− Most of free radicals in plant are oxygen free radicals (OFR). OFR accounts for more than 95% of free radical in plant and is mainly result of oxidation process. These free radicals can plunder other molecule's electrons, and damage DNA, RNA, and protein structure to impair the plant body. Normally, the speed of generation and elimination of ROS are balanced because of the activity of protective enzymes, including superoxide dismutase (SOD), catalase (CAT), glutathione peroxidase (GPX), and ascorbate peroxidase (APX) (Asada Citation1984). However, when plants are exposed to stress over an extended period of time, they accumulate more ROS than they eliminate. As soon as the balance is broken, the over-accumulation of ROS in plants leads to significant damage to the membrane system of plants from a lipid peroxidation reaction, which can increase permeability and conductivity (Gallego et al. Citation1996; Chaoui et al. Citation1997; Kaya et al. Citation2013).
Although plant growth, chlorophyll content, stomata opening, transpiration, and photosynthesis have been reported to be inhibited by Cd in nutrient solutions (Jiang & Li Citation1992; Baryla et al. Citation2001; Drążiewicz & Baszyński Citation2005; Sun et al. Citation2005), there have also been reports that Cd treatments had no effect on photosynthesis or growth (Greger & Lindberg Citation1986; Haag-Kerwer et al. Citation1999; Li et al. Citation2005; Zhou & Qiu Citation2005), and that N was possibly a bioactive signaling molecule in plant responses to heavy metal stress (Hassan et al. Citation2005; Floryszak-Wieczorek et al. Citation2006; Grün et al. Citation2006; Arasimowicz & Floryszak-Wieczorek Citation2007). However, despite all these studies, the precise mechanisms of how extra N alleviates the toxic symptoms caused by Cd stress remain unknown.
Our earlier study showed that after adding N to the treatment, the damage caused by Cd stress can be alleviated. In addition, these plants grew better than the plants without stress. However, the reason for this result remains unclear, because the generation of free radicals under Cd stress and the N-induced defense mechanism in poplar plants has not been studied thoroughly. Therefore, it is essential to investigate whether the gene expression of N-induced antioxidant enzymes, such as SOD, CAT, GPX, and APX, will increase the elimination of free oxygen radicals during Cd stress. Further, we want to figure out the detoxification mechanism of N to heavy metal stress in plant, which can provide new ideas and methodology to enhance the phytoremediation technology for heavy metal pollution.
Materials and methods
Plant material and treatment
The cutting poplar plants ‘107’ were selected as the experimental materials and NH4NO3 and CdCl2 as major reagents in the study. Forty-eight healthy cuttings with an average of 5 nodes and about 15 cm of height were sampled from 48 different trees of the poplar hybrid 107 (Populus deltoides × P. nigra) in Ya'an, southwestern China. These trees were collected from the same population and shared similar water and soil nutrient conditions. After sampling, these 48 cuttings were replanted into 16 plastic tubes (3 cuttings per tube, each tube was 100 × 50 × 20 cm, and contained a maximum of 60 L of solution), and each tube was filled with Hoagland nutrient solution. The cuttings were grown in a naturally lit greenhouse at the Sichuan Agricultural University and the Hoagland nutrient solution was replaced every 96 hours. When the cuttings grew to the 8–10 leaf stage (2–3 weeks old, 20 cm in height), they were subjected to the following four treatments: (T1) no Cd and no extra N (control; the standard Hoagland nutrient solution); (T2) extra N (45 µM) and no Cd, 2.10 g of NH4NO3 added to 1 L Hoagland nutrient solution (equivalent to 3 times the N concentration of the standard Hoagland nutrient solution); (T3) Cd (50 µM) and no extra N, 0.0059 g CdCl2 added to 1 L Hoagland nutrient solution; and (T4) Cd (50 µM) and extra N (45 µM). The amount of Cd (Cd2 +) added to each pot each time was equal among all the Cd treatments (T3 and T4); similarly, the amount of N per pot was equal between T2 and T4. Each treatment contained 4 tubes and 12 cuttings (). Leaf samples were harvested at 1, 6, 12, 24, 48, 120, 240, and 720 h after treatment and then immediately frozen in liquid N for enzyme activity and gene expression assays. During the 30 days of treatment, the treatment solutions were changed every 48 h to maintain the same N concentration.
Table 1. Experimental design of the dynamic response of poplar plants under Cd stress to N supplement.
Growth and chlorophyll content assays
Plant height and DBH (diameter at breast height) of each cutting were measured before and after treatment. Leaf samples were taken from the third leaf counting from the bottom of plant immediately following harvest and used to determine chlorophyll content. Chlorophyll was extracted in 80% acetone. Absorbance was measured at 663 nm and 645 nm by a spectrophotometer (UV-2450, Shimadzu Corporation, and Japan). Extinction coefficients and equations reported by Lichtenthaler (Citation1987) were used to calculate the quantity of chlorophyll. Measurements were done in triplicate.
H2O2 and MDA (malondialdehyde) concentration analysis
After 30 d of treatment, H2O2 in leaves of treated and control plants was measured by FOX1 method (Bellincampi et al. Citation2000). Fresh leaves (4 g) were homogenized with 8 mL of 100 mM sodium phosphate buffer (pH 7.2), and 10% (W/V) polyvinylpolypyrrolidone (PVPP) (Lu et al. Citation2009). The homogenates were centrifuged at 10,000g for 10 min at 4°C. To determine the H2O2 concentration, 500 µL of the supernatant was added to 1 mL of assay reagent (500 µM ammonium ferrous sulfate, 50 mM H2SO4, 200 µM xylenol orange, and 200 mM sorbitol) and 500 µl of 100 mM sodium phosphate buffer (pH 7.2). Absorbance at 560 nm was recorded by a spectrophotometer (UV-2450, Shimadzu Corporation, Japan) after 30 min of incubation in dark. Standard curves of H2O2 were obtained for each independent experiment. H2O2 concentration (nmol·g−1·FW) was calculated from the difference of the absorbance of the blank and test samples.
MDA content was measured according to Jiang et al. (Citation2009). Fresh leaves (1 g) for each sample was ground in 8 mL ice-cold phosphate buffer (50 mM, pH 7.8). The homogenate was centrifuged at 15,000g for 10 min at 4°C. Then, the filtrate was diluted 5 times for the measurement of MDA content. The 1.5 mL dilution was added into 2.5 mL 0.5% thiobarbituric acid solution (dissolved in 20% trichloroacetic acid) and then boiled for 15 min. The absorbance of the supernatants was determined at 532 and 600 nm, respectively in an ultraspec 2450 spectrophotometer (UV-2450, Shimadzu Corporation, Japan). MDA concentration (µmol·g−1·FW) was calculated from the difference of the absorbance of the blank and test samples.
Total soluble proteins analysis
In order to measure the total soluble proteins from leaves utilized Guy et al. (Citation1992) procedures. Leaves were homogenized in 50 mM Tris-Hcl, pH 7.5; 0.04% (v/v) 2-mercaptoethanol and 2 mM EDTA. Then centrifuged at 11,500g for 21 min at 4°C. Supernatant stored at −20°C for analysis. Protein concentration was determined according to the method of Bradford (Citation1976) using Bovine serum albumin as standard.
Antioxidant enzymes activity analysis
Superoxide dismutase (SOD) (EC 1.15.1.1)
For SOD extractions, fresh leaves (0.5 g each sample) were ground with a pre-chilled pestle and mortar in liquid N and extracted with 50 mM potassium phosphate buffer (pH 7.8), which contained 0.5 mM EDTA. Each homogenate was transferred to centrifuge tubes and was centrifuged at 15,000g, 4°C for 15 min. The supernatant was used for an enzyme activity assay. Total SOD activity was assayed by monitoring the inhibition of photochemical reduction of nitroblue tetrazolium (NBT) according to the methods of Sairam et al. (Citation2002). One unit of SOD activity was defined as the amount of enzyme required to cause 50% inhibition of the reduction of NBT as monitored at 560 nm.
Catalase (CAT) activity (EC 1.11.1.6)
CAT extraction was similar to the extraction of SOD. The enzymatic activity of CAT was assayed by following the initial rate of H2O2 degradation (Aebi Citation1984). A 3 ml reaction mixture contained 100 mM PBS (pH 7.0), 10 mM H2O2, and 100 µl of enzyme extract. Enzyme activity was assayed by monitoring the decrease in absorbance at 240 nm as a consequence of H2O2 consumption. The enzyme activity was calculated using the extinction coefficient (0.036 mM−1 cm−1) for H2O2. One unit of CAT specific activity was defined as the amount to decompose 1 µmol of H2O2 per min per mg of total protein.
Ascorbate peroxidase (APX) (EC 1.11.1.11)
APX was determined spectrophotometrically according to the oxidation of ASA (ascorbate). Fresh leaves (0.5 g each sample) were homogenized in 50 mM ice cold potassium phosphate buffer (pH 7.5), which contained 0.5 mM EDTA, 2 mM ascorbate (AsA), and 5% polyvinyl pyrrolidone (PVP), with a pre-chilled pestle and mortar. Other steps were similar to the extraction of other enzymes (Esfandiari et al. Citation2007). All operations were performed at 0–4°C. APX activity was measured according to Yoshimura et al. (Citation2000) by monitoring the rate of ascorbate oxidation at 290 nm. The unit of antioxidant enzyme activities present as the change of absorbance per minute and specific activities as enzyme units per mg of protein.
Guaiacol peroxidase (GPX) (EC 1.11.1.7)
GPX activity was measured using Guaiacol as a substrate. GPX was extracted according to the method of Nasibi et al. (Citation2011). Reaction mixture (3 ml) contained 25 µl of enzyme extract, 2.77 ml of 50 mM phosphate buffer (pH 7.0), 0.1 ml of 1% H2O2 (V/V), and 0.1 ml of 4% guaiacol (V/V). The increase in absorbance at 470 nm due to the guaiacol oxidation was recorded for 3 min. One unit of enzyme activity was defined as the amount that causes a change of 0.01 in absorbance per minute (Zhang et al. Citation2005).
Expression assay of antioxidant enzyme genes
RNA isolation
Total RNA was extracted from leaves as described by Verwoerd et al. (Citation1989). RNA integrity was verified by electrophoresis using ethidium bromide staining. First, cDNA strands were reverse transcribed according to the standard protocol of TaKaRa prime Script™ RT-PCR Kit. 500 ng RNA, 1.0 µl of 10 mmol/l dNTPs, 1.0 µl of 2.5 µmol/µl OligodT primer, 1.0 µl of 20 µmol/µl random primer, and 2 µl of RNase-free H2O were mixed and heated at 65°C for 5 min. After that, the mixture was immediately placed on ice for 2 min and then centrifuged for a short time. Then 4.0 µl of 5× first-strand buffers, 0.5 µl of 40 U/µl RNase inhibitor, 0.5 µl of the Primer Script™ RTase, and 5 µl RNase-free H2O were added and mixed together. 20 µl of reaction volume was subsequently incubated for 10 min at 30°C, 25 min at 45°C, 5 min at 95°C, and heated to 70°C for 15 min to stop the synthesis reaction and then held at 4°C.
Cloning and sequencing
Partial fragments of selected genes have been cloned and characterized in our laboratory (data not shown). Partial fragment sequence data were submitted to GenBank, and the accession numbers are listed in .
Table 2. The primers used in real-time RT-PCR.
Real-time RT-PCR
The first cDNA strand was reverse transcribed from 500 ng of DNase-treated RNA as described above. Based on the corresponding sequences of Cu/Zn-SOD, CAT, GPX, and APX, gene-specific primers were designed using the Primer Express software, version 2.0 (Applied Biosystems, Courtaboeuf, France). Gene expression profiles of response to Cd stress and N exposure were constructed using real-time RT-PCR with specific primers (). Real-time quantitative PCR was performed by Bio-Rad iQ5 (Bio-Rad Company), and the results were analyzed by an optical system version 2.0 (Bio-Rad) software. The actin gene of the poplar used as the inner reference gene was amplified alone with the target gene which allowing gene normalization and quantification. Based on the reaction protocol of the SYBR Premix Ex Taq™ II Kit (TaKaRa), the reaction system was listed as follows: 10 µl of SYBR Premix Ex Taq™ II (2×), 0.8 µl of each Primer (10 µmol/l), 1.5 µl template (cDNA solution), and 6.9 µl of dH2O. The two-step PCR reaction program was present as follow: pre-denaturalized at 95°C for 10 s, 95°C for 5 s and 55°C for 20 s, the PCR reaction with 40 cycles, and then kept at 72°C for 2 min. After the PCR reaction, a melting curve was generated for each sample at the end of each run to assess the purity of the amplified products. Real-time PCR was carried out in triplicate (technical repeats) to ensure the reproducibility of the results. The relative expression level was calculated as the transcription level under stress treatment using the 2−ΔΔCT method as described by Livak and Schmittgen (Citation2001).
Statistical analysis
Data are expressed as mean−standard error unless otherwise stated. All statistical analyses were performed by SAS software (version 8.0, SAS Institute, Cary, North Carolina, USA). All data met assumptions of normal distribution and equality of variance. Analysis of variance (ANOVA) was then used to determine the difference between the control and the treatment groups. Tukey's test of least significant difference (LSD) was applied for multiple comparisons when needed. Differences were considered statistically significant at P < 0.01.
Results
Effect of Cd treatment on plant growth
The height and DBH of poplar exposed to the treatments for 30 days are shown in . The results indicated that a significant growth inhibition was found in leaves of poplar plants treated with only Cd over the study period. The average height and DBH decreased 42.3% and 29.8%, respectively, compared to the control, and the leaves became chlorotic. In contrast, height and DBH increased 43.3% and 35.4%, respectively, under the only N treatment compared to the control. In addition, the plants treated with N + Cd grew well without any toxic symptoms, and a significant increase of growth was observed at day 30 (70.3% and 63.1% respective to the control) (). The difference in the growth of plants among the treatments was significant, and the interaction of Cd and N more significantly promoted the growth of poplar than N alone ( and ).
Table 3. Interactive effects of Cd and N treatment on growth of poplar plant height (cm) and DBH (cm). After 30 days of treatment, plant height and DBH were measured.
Effect of Cd on chlorophyll content
In order to evaluate the oxidative stress caused by Cd, chlorophyll content after 30 days of treatment was examined. Chlorophyll content varied widely among the treatments (). After 30 days of Cd treatment, there was a decrease of 30%, 48%, and 35% for Chl a, Chl b, and total Chl, respectively, relative to the control values. The chlorophyll a:b ratio (4.62:1) was substantially higher than that of the control (3.43:1), which indicates severe inhibition of Cd on Chl b synthesis. Surprisingly, chlorophyll levels were enhanced in poplar plants treated with N + Cd. A significant increase of 69.6%, 41.2%, and 63.2% of Chl a, Chl b, and total Chl compared to the control was found at day 30 (). The results suggest that the addition of N can significantly increase chlorophyll content in leaves under Cd stress.
Table 4. Interactive effects of Cd and N treatment on the chlorophyll content in poplar leaves.
Table 5. Analysis of variance significance for the interaction effects of Cd and N on height, DBH, and chlorophyll content in poplar plants.
Effect of Cd on H2O2 and MDA content
H2O2 and MDA are the products of membrane lipid peroxidation, and their content is related to the degree of membrane lipid peroxidation. Thus, they serve as the major indexes of heavy metal stress. The trends of H2O2 and MDA contents in all treatments were alike shown in . The H2O2 and MDA contents in the leaves of poplar plants increased by adding exogenous CdCl2, and their contents in plants treated with Cd were significantly higher in comparison with only N treatment and the control. Interestingly, their contents showed a significant decrease in plants treated with N + Cd when compared to sole Cd treatment over the study period (), while their content maintained at a low level in the plants treated with NH4NO3 during the study period. The results indicated that Cd stress actually stimulates the accumulation of reactive oxygen species (ROS) and N supplement under stress of Cd2 + can alleviate the production of ROS.
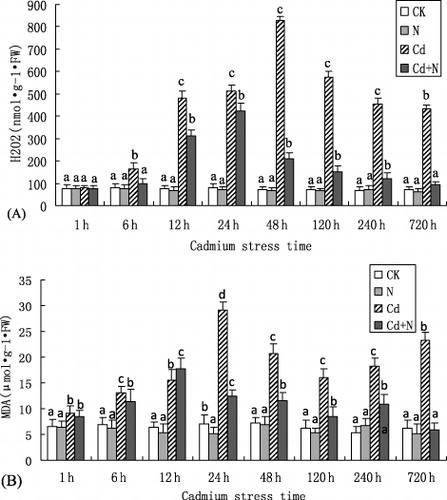
Effect of Cd on SOD, CAT, GPX, and APX activities
The antioxidant enzymatic system is one of the protective mechanisms that can eliminate reactive oxygen species (ROS) in plant cells and alleviate stress-induced oxidative damage (Beak & Skinner Citation2003). As it can be seen in , Cd exposure induced remarkable changes in SOD, CAT, GPX, and APX activities in poplar leaves over the study period. SOD activity showed a slight increase under the N treatment compared to the control, but remarkably increased under the Cd and N + Cd treatments. Additionally, SOD activities peaked at 24 h under Cd and N + Cd stress. The average activities for SOD were in this order: N + Cd > Cd > N > CK ().
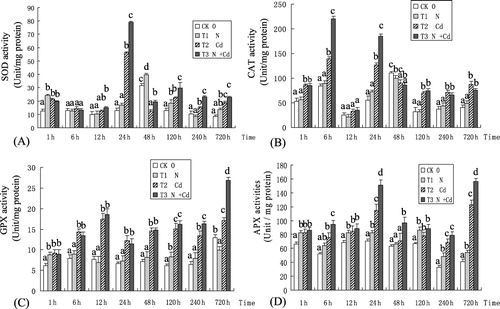
For the CAT activities, there was a significant increase in poplar leaves in plants treated with Cd or N + Cd over the study period (). Conversely, N exposure alone presented no effect on CAT activity in poplar leaves compared to the control. The CAT activities showed a peak at 6 h and 24 h under N + Cd treatment, and a similar pattern in the Cd treatment ().
shows that GPX activity was almost unaltered in the poplar leaves in the N treatment versus the control. However, it was higher under the N + Cd and Cd treatments than the control, respectively. APX showed the same patterns as GPX in response to different treatments and presented a fluctuant curve. The APX activity levels in poplar leaves were N + Cd > Cd > N > CK ().
Expression profiling of Cu/Zn-SOD, CAT1, CAT2, GPX, and APX genes under Cd stress
illustrates the variation of five transcript gene levels in poplar leaves from 1 h to 30 d, which corresponds to Cd stress. Cu-Zn/SOD was upregulated compared to the control for all treatments, but the levels were different among the treatments (). SOD transcript level showed a slight change under the N treatment compared to the control, while it increased under Cd and N + Cd treatments. Surprisingly, the SOD gene was most induced at 24 h under Cd stress and N + Cd treatments. Further, a significant increase of the SOD gene was 4.2 and 5.4 times higher than the control, respectively, after 24 h of stress. Similarly, the average expression value of SOD under all the treatments increased 107.7% and 138.5% more than the control, respectively, suggesting that SOD was upregulated by Cd stress, especially by N + Cd.
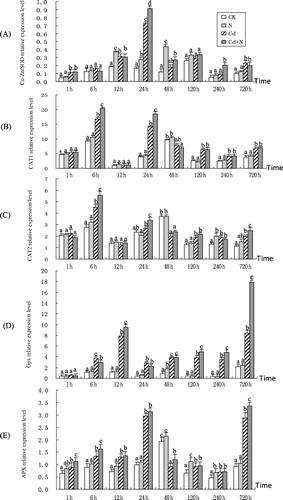
CAT alleles showed two isoforms (CAT1 and CAT2), which were detected in all the leaves at all the measured times. CAT3 was not found. CAT1 and CAT2 were cloned and analyzed for their response to Cd stress. CAT1 and CAT2 shared similar expression profiles and were both enhanced by Cd and N + Cd treatments compared to the control (). Their expression levels increased sharply, followed by a gradual decrease, and then another increase. They exhibited a peak at 6 and 24 h respectively, and reached their lowest expression at 12 h. No significant difference of transcript levels of CAT1 and CAT2 between control and N treatment was detected in the poplar leaves analyzed. The average expression levels for CAT1 and CAT2 were both in this order: N + Cd > Cd > N > CK ( and ).
GPX was unchanged under the N treatment at all of the treatment times, but it was upregulated by Cd and N + Cd stress (). GPX expression for Cd and N + Cd increased 7.9 and 6.6 times compared to that of the control at 12 h, and 8.1 and 3.9 times higher than the control at 30 d under stress, respectively. The trend of GPX gene expression indicated that the plant turned on its defense mechanism immediately after sensing Cd stress and rapidly upregulated the GPX gene to synthesize the amount of GPX enzyme that can catalyze L-Glutathione reduced (GSH) to L-Glutathione oxidized (GSSG) and eliminate active oxygen (H2O2) in plant cells.
The level of APX gene expression showed a stable fluctuation under the N treatment during the treatment periods and had no significant difference compared to the control, which indicated the N treatment had no significant influence on APX gene expression in poplar (). On the other hand, under the Cd and N + Cd treatments, the APX gene expression fluctuated greatly. During the first 24 h, the expression of the APX gene in poplar upregulated dramatically and peaked at 24 h with the increase of 202.0% and 218.4% than that of the control, respectively. Further, after 24 h, the APX gene expression of both treatments downregulated dramatically and bottomed out at 240 h, then gradually increased and reached another peak at 30 d. The trend of APX gene expression indicated that plants rapidly upregulate the APX gene to synthesize the APX enzyme, which can eliminate active oxygen (H2O2) in plant cells and alleviate stress-induced oxidative damage during the first 24 h.
Discussion
Cd-dependent inhibition of growth has been reported in several plant species (Baryla et al. Citation2001; Hammami et al. Citation2004; Amani Citation2008; Anjum et al. Citation2008; John et al. Citation2008). Lozano-Rodríguez et al. (Citation1997) demonstrated that there is a 70% and 30% growth inhibition in pea and maize plants, respectively, treated with Cd. There are others reports that Cd treatments have no effect on photosynthesis or growth (Li et al. Citation2005; Zhou & Qiu Citation2005). In the present study, Cd caused a significant reduction in the growth of poplar plants (). Average height and DBH decreased 42.3% and 29.8% compared to the control after 30 d of Cd treatment, respectively. The plants treated with N + Cd grew well without toxic symptoms. The height, DBH, and Chl content showed a dramatic increase. Our past study also revealed that N addition could promote the growth and increase the content of Chl a and Chl b, photosynthesis rate, and PS II quantum yields of Eucalyptus grandis plants under stress of Cd2 +, Pb2 +, and Cu2 +, respectively (Wan et al. Citation2012). Heavy metal actually inhibited the growth of plants. However, exogenous N can mitigate the damages induced by heavy metal. Sodium nitroprusside (SNP, a donor of NO) (100µM) had obvious alleviated effect on Cu toxicity in ryegrass seedlings (Dong et al. Citation2013). Similarly, Li et al. (Citation2007) reported that adding the appropriate amount of N to plants could enhance the development of root systems and increase the accumulation of Cd in Sedum alfredii Hance. The biomass of Kandelia candel dramatically increased with the increased N supplement under the same concentration of Cd stress (Zhang Citation2008). The above results indicate that N, as a bioactive signaling molecule, may play an important physiological role in detoxifying plants from heavy metals, and the detoxification of Cd in poplar by sufficient N may be a common mechanism in all plants to other heavy metals such as Pb, Cu, As, and Hg. If this reasoning is confirmed in the future, phytoremediation of contaminated soil may be enhanced with N fertilization.
However, the precise mechanisms of how extra N can alleviate the toxic symptoms in plants caused by Cd stress remain unknown. Plants damaged by Cd always present chlorosis, growth inhibition, or death. On the other hand, when faced with Cd stress, plants exert a number of strategies to defend against Cd toxicity. According to related research, three of detoxification strategies are as follows: (1) The presence of some chelate complexes, such as phytochelatin-Cd2 + or GSH-Cd2 + (Quan et al. Citation2006; Gustavo et al. Citation2007); (2) Elimination of ROS by antioxidants (Sun & Yang Citation2006; Romero-Puertas et al. Citation2007); and (3) Regulation of related gene expression (Benavides et al. Citation2005; Xu et al. Citation2006; Verbruggen et al. Citation2009). In this study, the results indicated that N + Cd obviously promoted the growth of poplar plants and improved chlorophyll content. Cd-induced oxidative damages were eliminated by exogenous N, reflected by decreased accumulation of hydrogen peroxide (H2O2), and malondialdehyde (MDA). Moreover, exogenous N increased Cd-decreased activities of SOD, CAT, APX, and GPX. So the positive interaction effects of Cd and N () can partially explain the protective effect of excess N in plants facing heavy metal stress.
In addition, by comparing expression levels of Cu/Zn-SOD, CAT1, CAT2, GPX, and APX genes under N, Cd, and N + Cd treatments (), we still hypothesize that N may play an important physiological role in detoxifying plants from heavy metals. N upregulated the gene expression to create more antioxidant enzymes. The more antioxidant enzymes exist, the more ROS is eliminated. ROS always exerts a variety of damaging effects such as increased permeability and conductivity of the membrane system (David & Rafael Citation2006).
The oxidative stress caused by adverse factors including heavy metal stress can be alleviated by exogenous N. Xu et al. (Citation2013) revealed that Cd-induced oxidative damages were eliminated by exogenous NO, reflected by decreased accumulation of hydrogen peroxide (H2O2), and malondialdehyde (MDA). Moreover, exogenous NO increased Cd-decreased activities of superoxide dismutase (SOD), peroxidase (POD), and catalase (CAT) in peanuts. In addition, the oxidative stress caused by NaCl in Azolla caroliniana is mitigated by nitrate (Mostafa & Tammam Citation2012). Arginine increased the activity of SOD, APX, and glutathione reductase (GR) and decreased the content of H2O2 and MDA in tomato plants. The oxidative damage in tomato plants underwater stress was alleviated by exogenous arginine (Nasibi et al. Citation2011). On the contrary, if N is deficient in plant body, reactive oxygen species (ROS) level will be increased (Kong et al. Citation2013). The above results demonstrated that N played an important role in eliminating ROS and in detoxifying heavy metals from plants.
On the other hand, N contributes to the detoxification of plants to Cd by sequestration with Glutathione (GSH) and phytochelation (PC). GSH can directly eliminate the over-accumulation of ROS by a reduction reaction. GSH also can be used for PC synthesis. Both GSH and PC could bind Cd2 + to form a stable chelate (GSH-Cd2 + or PC-Cd2 +) and then transfer chelates into vacuoles. Both these direct and indirect methods can efficiently decrease the concentration of Cd and alleviate the damage caused by ROS in plants (Tennstedt et al. Citation2009; Park et al. Citation2012; Wang et al. Citation2012). In our experiment, the gene expression under the N treatment showed no significant difference compared to the control, but these genes were upregulated in Cd and N + Cd treated plants. This difference might be because the plants allocated extra N to growth instead of defense when not under Cd stress, and this hypothesis could explain our result that plants had better growth and higher chlorophyll content under the N treatment. In the meantime, the difference of expression levels of these genes between Cd and N + Cd treatments weas significant in the study, which indicates that adding extra N to poplar under Cd stress could dramatically induce the upregulation expression of these genes. Moreover, the presence of extra N also can provide enough gradients, such as L-glutamate and L-cysteine, for enzymes to synthesize GSH and PC, which could help plants efficiently alleviate Cd stress. This theory also explains why the toxic symptoms only occurred when N supplementation was limited. When extra N was insufficient, more Cd2 + entered into plant cells, and the plants allocated more N to enhance GSH synthesis to protect themselves against Cd stress. This action led to a deficiency of glutamate, which is used for chlorophyll synthesis and growth in plant. Thus, chlorosis and growth inhibition occurred. When abundant exogenous N was imported into the plant cells and the plant had enough N to synthesize chlorophyll, promoting plant growth, but also had excess N to biosynthesize GSH for free Cd2 + chelation. It can be said that with a sufficient supplement of N, plants would never be threatened by Cd stress.
The important physiological role of N in detoxifying plants from heavy metals has not been studied thoroughly. However, that nitric oxide (NO) acts as a bioactive signaling molecule in plant responses to heavy metal stress has been reported in many studies (Hassan et al. Citation2005; Floryszak-Wieczorek et al. Citation2006; Grün et al. Citation2006; Arasimowicz & Floryszak-Wieczorek Citation2007). It was documented that exogenous NO reduces the destructive action of heavy metals, ethylene, and herbicides on plants (Kopyra & Gwózdz Citation2003). Garcia-Mata and Lamattina (Citation2001) reported that NO induced stomatal closure and enhanced the adaptive plant responses against drought stress. In this study, extra N was added in the form of NH4NO3 to a Hoagland nutrient solution. Whether or not NH4 + or NO3 − would also detoxify plants from heavy metals remains unknown. Therefore, the question of which form of N works best in the detoxification process remains to be studied.
Conclusion
Cd contamination has a detrimental effect on plant growth and development. Over-accumulation of Cd in plants shows various symptoms of toxicity, including growth inhibition, chlorotic leaves, and a decrease in biomass. In this study, the researchers discovered that N could effectively alleviate Cd induced damage in poplar (P. deltoides × P. nigra). Adding supplemental N to poplars under Cd stress can enhance plant growth, promote the synthesis of chlorophyll, and induce the activity of antioxidant enzymes and gene expression. This result indicates that N partially alleviated the toxic effect of heavy metal on poplar plants, and N may play an important physiological role in the detoxification of heavy metal stress on plant.
Furthermore, it is worth mentioning that our experiments only studied the effect of extra N on antioxidant enzymes and gene expression in poplars and had no further study on other metabolic pathways, such as protein synthesis. As a result, our hypothesis about the detoxification effect and mechanism of N on plant under Cd stress is not complete and needs further study.
Acknowledgments
This work was supported by the National Natural Science Foundation of China (No. 31300514) and by the 12th Five Year Key Programs for forest breeding in Sichuan Province (No. 2011YZGG).
References
- Aebi H. 1984. Catalase in vitro. Methods Enzymol. 105:121–126.
- Amani AL. 2008. Cadmium induced changes in pigment content, ion uptake, proline content and phosphoenolpyruvate carboxylase activity in Triticum aestivum seedlings. Aust J Basic Appl Sci. 2:57–62.
- Anjum NA, Umar S, Ahmad A, Iqbal M, Khan NA. 2008. Ontogenic variation in response of Brassica campestris L. to cadmium toxicity. J Plant Interact. 3:189–198. doi:10.1080/17429140701823164
- Arasimowicz M, Floryszak-Wieczorek J. 2007. Nitric oxide as a bioactive signalling molecule in plant stress responses. Plant Sci. 172:876–887. doi:10.1016/j.plantsci.2007.02.005
- Asada K. 1984. Chloroplasts: formation of active oxygen and its scavenging methods. Enzymol. 10:422–429.
- Baryla A, Carrier P, Franck F, Coulomb C, Sahut C, Havaux M. 2001. Leaf chlorosis in oilseed rape plants (Brassica napus) grown on cadmium-polluted soil: cause and consequences for photosynthesis and growth. Planta. 212:696–709. doi:10.1007/s004250000439
- Beak KH, Skinner DZ. 2003. Alteration of antioxidant enzyme gene expression during cold acclimation of near-isogenic wheat lines. Plants Sci. 165:1221–1227. doi:10.1016/S0168-9452(03)00329-7
- Bellincampi D, Dipierro N, Salvi G, Cervone F, Lorenzo GD. 2000. Extracellular H2O2 induced by oligogalacturonides is not involved in the inhibition of the auxin-regulated rolB gene expression in tobacco leaf explants. Plant Physiol. 122:1379–1385. doi:10.1104/pp.122.4.1379
- Benavides M, Gallego S, Tomaro M. 2005. Cadmium toxicity in plants. Brazilian J Plant Physiol. 17:21–34. doi:10.1590/S1677-04202005000100003
- Bradford MM. 1976. A rapid and sensitive method for quantitation of microgram quantities of protein utilizing the principle of protein-dye binding. Anal Biochem. 72:248–254. doi:10.1016/0003-2697(76)90527-3
- Brunetti G, Farrag K, Solerrovira P, Ferrara M, Nigro F, Senesi N. 2012. Heavy metals accumulation and distribution in durum wheat and barley grown in contaminated soils under Mediterranean field conditions. J Plant Interact. 7:160–174. doi:10.1080/17429145.2011.603438
- Chaney RL, Ryan JA, Li YM, Brown SL. 1999. Soil cadmium as a threat to human health. In: McLaughlin MJ, Singh BR, editors. Cadmium in soils and plants. Dordrecht: Kluwer Academic Publishers; p. 219–256.
- Chaoui A, Mazhoudi S, Ghorbal MH, Ferjani EL. 1997. Cd and Zinc induction of lipid peroxidation and effects on antioxidant enzyme activities in bean (Phaseolus vulgaris L.). Plant Sci. 127:139–147. doi:10.1016/S0168-9452(97)00115-5
- David GM, Rafael M. 2006. Control of glutathione and phytochelatin synthesis under cadmium stress. Pathway modeling for plants. J Theor Biol. 238:919–936. doi:10.1016/j.jtbi.2005.07.003
- Dietz KJ, Baier M, Krämer U. 1999. Free radicals and reactive oxygen species as mediators of heavy metals toxicity in plants. In: Prasad MNV, Hagemeyer J, editors. Heavy metal stress in plants. Berlin, Heidelberg: Springer-Verlag; p. 73–97.
- Dong Y, Xu L, Wang Q, Fan Z, Kong J, Bai X. 2013. Effects of exogenous nitric oxide on photosynthesis, antioxidative ability, and mineral element contents of perennial ryegrass under copper stress. J Plant Interact. doi:10.1080/17429145.2013.845917
- Drążiewicz M, Baszyński T. 2005. Growth parameters and photosynthetic pigments in leaf segments of Zea mays exposed to cadmium, as related to protection mechanisms. J Plant Physiol. 162:1013–1021. doi:10.1016/j.jplph.2004.10.010
- Esfandiari EA, Shakiba MR, Mahboob SA, Alyari H, Toorchi M. 2007. Water stress, antioxidant enzyme activity and lipid peroxidation in wheat seedling. J Food Agri Environ. 5:48–53.
- Floryszak-Wieczorek J, Milczarek G, Arasimowicz M, Ciszewski A. 2006. Do nitric oxide donors mimic an endogenous NO-related response in plants? Planta. 224:1363–1372. doi:10.1007/s00425-006-0321-1
- Gallego SM, Benavy des MP, Tomaro M. 1996. Effect of heavy metal ion excess on sunflower leaves: evidence for involvement of oxidative stress. Plants Sci. 121:151–159. doi:10.1016/S0168-9452(96)04528-1
- Garcia-Mata C, Lamattina L. 2001. Nitric oxide induces stomatal closure and enhances the adaptive plant responses against drought stress. Plant Physiol. 126:1196–1204. doi:10.1104/pp.126.3.1196
- Greger M, Lindberg S. 1986. Effects of Cd2 + and EDTA on young sugar beets (Betavulgaris). I.Cd2 + uptake and sugar accumulation. Plant Physiol. 66:69–74. doi:10.1111/j.1399-3054.1986.tb01235.x
- Grün S, Lindermayr C, Sell S, Durner J. 2006. Nitric oxide and gene regulation in plants. J Exp Bot. 57:507–516. doi:10.1093/jxb/erj053
- Gustavo G, Ana JF, Diego MS, María LT. 2007. Glutathione reductase activity and isoforms in leaves and roots of wheat plants subjected to cadmium stress. Phytochemistry. 68:505–512. doi:10.1016/j.phytochem.2006.11.016
- Guy C, Haskell D, Neven L, Klein P, Smelser C. 1992. Hydration-state-responsive proteins link cold and drought stress in spinach. Planta. 188:265–270. doi:10.1007/BF00216823
- Haag-Kerwer A, Heiss S, Walter C, Rausch T, Schafer HJ. 1999. Cadmium exposure in Brassica juncea causes a decline in transpiration rate and leaf expansion without effect on photosynthesis. J Exp Bot. 341:1827–1835.
- Hammami SS, Chaffai R, Ferjani EE. 2004. Effect of cadmium on sunflower growth, leaf pigment and photosynthetic enzymes. Pakistan J Biol Sci. 7:1419–1426. doi:10.3923/pjbs.2004.1419.1426
- Hassan MJ, Wang F, Ali S, Zhang G. 2005. Toxic effect of Cd on rice as affected by nitrogen fertilizer form. Plant Soil. 277:359–365. doi:10.1007/s11104-005-8160-6
- Jiang J, Zhuang JY, Fan YY, Shen B. 2009. Mapping of QTLs for leaf malondialdehyde content associated with stress tolerance in rice. Rice Sci. 16:72–74. Chinese. doi:10.1016/S1672-6308(08)60059-1
- Jiang WZ, Li JL. 1992. Effect of cadmium on the construction of photosynthetic membrane system. J Yunnan University 14:318–323. Chinese.
- John R, Ahmad P, Gadgil K, Sharma S. 2008. Effect of cadmium and lead on growth, biochemical parameters and uptake in Lemna polyrrhiza L. Plant Soil Environ. 54:262–270.
- Kaya C, Sonmez O, Aydemir S, Ashraf M, Dikilitas M. 2013. Exogenous application of mannitol and thiourea regulates plant growth and oxidative stress responses in salt-stressed maize (Zea mays L.). J Plant Interact. 8:234–241. doi:10.1080/17429145.2012.725480
- Kong L, Wang F, Si J, Feng B, Zhang B, Li S, Wang Z. 2013. Increasing in ROS levels and callose deposition in peduncle vascular bundles of wheat (Triticum aestivum L.) grown under nitrogen deficiency. J Plant Interact. 8:109–116. doi:10.1080/17429145.2012.712723
- Kopyra M, Gwózdz EA. 2003. Nitric oxide stimulates seed germination and counteracts the inhibitory effect of heavy metals and salinity on root growth of Lupinus luteus. Plant Physiol Biochem. 41:1011–1017. doi:10.1016/j.plaphy.2003.09.003
- Li DM, Zhu ZJ, Liu YH. 2005. Influence of cadmium on photosynthesis of Brassica campestris ssp. chinensis L. J Zhejiang University (Agric & Life Sci). 31:459–464. Chinese.
- Li JG, Jin SL, Chen YQ, Lin GL, Han XR, Li TQ, Yang XE, Zhu E. 2007. Effects of nitrogen fertilizer on the root morphology and cadmium accumulation in low cadmium treatment Sedum alfredii Hance. Chin Agric Sci Bull 23:260–265. Chinese.
- Lichtenthaler HK. 1987. Chlorophylls and carotenoids: pigments of photosynthetic biomembranes. Methods Enzymol. 148:350–382.
- Livak KJ, Schmittgen TD. 2001. Analysis of relative gene expression data using real-time quantitative PCR and the 2−ΔΔCT method. Methods. 25:402–408. doi:10.1006/meth.2001.1262
- Lozano-Rodríguez E, Hernández LE, Bonay P, Carpena-Ruiz RO. 1997. Distribution of cadmium in shoot and root tissues of maize and pea plants: physiological disturbances. J Exp Bot. 48:123–128. doi:10.1093/jxb/48.1.123
- Lu SM, Jun S, Campbell-Palmer L. 2009. A modified chemiluminescence method for hydrogen peroxide determination in apple fruit tissues. Sci Hortic. 3:336–341. doi:10.1016/j.scienta.2008.11.003
- McLaughlin MJ, Parker DR, Clarke JM. 1999. Metals and micronutrients-food safety issues. Field Crop Res. 60:143–163. doi:10.1016/S0378-4290(98)00137-3
- Mostafa EM, Tammam AA. 2012. The oxidative stress caused by NaCl in Azolla caroliniana is mitigated by nitrate. J Plant Interact. 7:356–366. doi:10.1080/17429145.2011.628452
- Nasibi F, Yaghoobi MM, Kalantari KM. 2011. Effect of exogenous arginine on alleviation of oxidative damage in tomato plant underwater stress. J Plant Interact. 6:291–296. doi:10.1080/17429145.2010.539708
- Park J, Song WY, Ko D, Eom Y, Hansen TH, Schiller M, Lee EM, Lee Y. 2012. The phytochelatin transporters AtABCC1 and AtABCC2 mediate tolerance to cadmium and mercury. Plant J. 69:278–288. doi:10.1111/j.1365-313X.2011.04789.x
- Pereira GJG, Molina SMG, Lea PJ, Azevedo RA. 2002. Activity of antioxidant enzymes in response to cadmium in Crotalaria juncea. Plant Soil. 239:123–132. doi:10.1023/A:1014951524286
- Quan XQ, Zhang HT, Shan L, Bi YP. 2006. Advances in plant metallothionein and its heavy metal detoxification mechanisms. Hereditas. 28:375–382.
- Romero-Puertas MC, Corpas FJ, Rodríquez-Serrano M, Gómez M, Del Río LA, Sandalio LM. 2007. Differential expression and regulation of antioxidative enzymes by cadmium in pea plants. J Plant Physiol. 164:1346–1357. doi:10.1016/j.jplph.2006.06.018
- Romero-Puertas MC, Rodríguez-serrano M, Corpas FJ, Gómez M, Delrío LA, Sandalio LM. 2004. Cadmium-induced subcellular accumulation of O2 and H2O2 in pea leaves. Plant Cell Environ. 27:1122–1134. doi:10.1111/j.1365-3040.2004.01217.x
- Sairam RK, Rao KV, Srivastava GC. 2002. Differential response of wheat genotypes to long term salinity stress in relation to oxidative stress, antioxidant activity and osmolyte concentration. Plants Sci. 163:1037–1046. doi:10.1016/S0168-9452(02)00278-9
- Sandalio LM, Dalurzo HC, Gomez M, Romero-Puertas MC, del Rio LA. 2001. Cadmium-induced changes in the growth and oxidative metabolism of pea plants. J Exp Bot. 52:2115–2126.
- Sanità di Toppi L, Gabbrielli R. 1999. Response to cadmium in higher plants. Environ. Exp. Bot. 41:105–130. doi:10.1016/S0098-8472(98)00058-6
- Sun GW, Chen RY, Liu HC. 2005. Advances on investigation of effect of cadmium on photosynthesis and nitrogen metabolism of plant. Chin Agri Sci Bull. 9:234–236. Chinese.
- Sun XM, Yang ZM. 2006. Journal of plant sulfate assimilation and regulation of the activity of related enzymes under cadmium stress. Physiol Mol Biol. 32:9–16.
- Tennstedt P, Peisker D, Böttcher C, Trampczynska A, Clemens S. 2009. Phytochelatin synthesis is essential for the detoxification of excess zinc and contributes significantly to the accumulation of zinc. Plant Physiol. 149:938–948. doi:10.1104/pp.108.127472
- Verbruggen N, Hermans C, Schat H. 2009. Mechanisms to cope with arsenic or cadmium excess in plants. Curr Opin Plant Biol. 12:364–372. doi:10.1016/j.pbi.2009.05.001
- Verwoerd TC, Dekker BMM, Hoekema AA. 1989. Small-scale procedure for the rapid isolation of plant RNAs. Nucleic Acids Res. 17:2362–2362. doi:10.1093/nar/17.6.2362
- Wagner GJ. 1993. Accumulation of cadmium in crop plants and its consequences to human health. Adv Agron. 51:173–212.
- Wan XQ, Zhang F, Wang CL, Ding YH. 2012. Effects of nitrogen supplement on photosynthetic characteristic and growth rate of Eucalyptus Grandis under three kind of heavy metal stress. J Nuclear Agri Sci. 26:1087–1093. Chinese.
- Wang F, Wang Z, Zhu C. 2012. Heteroexpression of the wheat phytochelatin synthase gene (TaPCS1) in rice enhances cadmium sensitivity. Acta Biochim Biophys Sin. 44:886–893. doi:10.1093/abbs/gms073
- Xu L, Dong Y, Fan Z, Kong J, Liu S, Bai X. 2013. Effects of the application of exogenous NO at different growth stage on the physiological characteristics of peanut grown in Cd-contaminated soil. J Plant Interact. doi:10.1080/17429145.2013.830780
- Xu ZH, Shen GJ, Zhu CQ, Xu LJ, He Y, Yu GS. 2006. Molecular mechanisms of plant resistance to cadmium toxicity. Chin J Appl Ecol. 17:1112–1116.
- Yoshimura K, Yabute Y, Ishikawa T, Shigeoka S. 2000. Expression of spinach ascorbate peroxidase isoenzymes in response to oxidative stresses. Plant Physiol. 123:223–233. doi:10.1104/pp.123.1.223
- Zhang WQ. 2008. Variation of some physiological process and biomass of Kandelia candel under stress of N and Cd [The academic dissertation]. Fuzhou: Fujian Agriculture and Forestry University.
- Zhang Z, Pang X, Duan X, Ji ZL, Jiang Y. 2005. Role of peroxidase in anthocyanin degradation in litchi fruit pericarp. Food Chem. 90:47–52. doi:10.1016/j.foodchem.2004.03.023
- Zhou WB, Qiu BS. 2005. Effects of cadmium hyperaccumulation on physiological characteristics of Sedum alfredii Hance (Crassulaceae). Plant Sci. 169:737–745. doi:10.1016/j.plantsci.2005.05.030