Abstract
We assessed the role of plant growth-promoting rhizobacteria (PGPR) strains viz. Burkholdera cepacia SE4, Promicromonospora sp. SE188 and Acinetobacter calcoaceticus SE370 in counteracting salinity and drought stress to cucumber plants. The control plants had stunted growth, while PGPR-treated plants had significantly higher biomass and chlorophyll contents under salinity and drought stress. The ameliorative effects of PGPR-application were also evidenced by the increased water potential and decreased electrolytic leakage. The PGPR-applied plants had reduced sodium ion concentration, while the potassium and phosphorus were abundantly present as compared to control under stress. Oxidative stress was mitigated by PGPR through reduced activities of catalase, peroxidase, polyphenol oxidase, and total polyphenol as compared to control. The control plants showed up-regulation of stress-responsive abscisic acid as compared to PGPR application, while salicylic acid and gibberellin 4 were significantly higher in PGPR. In conclusion, the PGPR application might be used in marginalized agricultural lands to increase crop productivity.
Introduction
Agricultural crops are exposed to many stresses that are induced by both biotic and abiotic factors. Abiotic stress includes high and low temperature, salinity, drought, flooding, ultraviolet light, air pollution (ozone), and heavy metals. These stresses reduced the yield of crops. The yield losses associated with such stressful conditions can reach up to 50–82%, depending on the type of crop and stress period (Christensen et al. Citation2007). In many semi-arid and arid regions of the world, crop yield is limited due to increased rate of soil salinity. Salinity and drought are the two most common abiotic stresses, which have severely affected plant growth and biomass production since long. It is estimated that the global area of about 7.6 million km2 face a high risk of deterioration due to such environmental impacts (Christensen et al. Citation2007; Falkenmark Citation2013). Similarly, it is projected that up to the year 2050, the land area affected by drought will increase two-fold and water resources will decline by 30% (Falkenmark Citation2013).
Plants undergo a number of metabolic and physiological changes in response to salinity and water deficiency (drought) (Saharan & Nehra Citation2011). Plants are bestowed with the capability to respond via signal transduction pathways adjusting their metabolism (Bartels & Sunkar Citation2005). A defensive strategy of plants against such stressful conditions encompasses a cascade of signals ranging from primary (like changes in ionic/osmotic levels, stomatal closer, etc.) to secondary (e.g. phytohormones and secondary metabolites etc) responses. Salinity imposes ionic (mainly due to Na+, Cl−, and SO42−), osmotic, and secondary stresses such as nutritional imbalances and oxidative stress for glycophytes (Hussain et al. Citation2008). Drought affects the turgor pressure and biomass production (Bartels & Sunkar Citation2005), although drought is more pervasive and devastating than salinity; however, plants' responses to both is closely related (Hussain et al. Citation2008).
To rescue plant growth in such stressful conditions, plant growth-promoting rhizobacteria (PGPR) have been known to play an essential role in the growth and metabolism of plants. The occurrence and activity of soil microorganisms are affected by a variety of environmental factors as well as plant-related factors (species and age). In last two decades or so, various PGPR strains have been known to play an essential role in improving crop growth. Certain varieties of PGPR strains belonging to Bacillus, Enterobacter, Burkholderia, Acinetobacter, Alcaligenes, Arthrobacter, Azospirillium, Azotobacter, Beijerinckia, Erwinia, Flavobacterium, Rhizobium and Serratia are now being used worldwide with the aim to enhance the crop productivity (Burd et al. Citation2000; Chaiharn et al. Citation2008; Yang et al. Citation2009; Bharti et al. Citation2013). These PGPRs stimulate plant growth and enhance plant biomass (Adesemoye et al. Citation2009; Lugtenberg & Kamilova Citation2009). The beneficial effects of PGPRs have been demonstrated for many agricultural crop species such as wheat (Khalid et al. Citation2004), tobacco, Brassica juncea (Asghar et al. Citation2002) tomatoes (Kidoglu et al. Citation2008), bell peppers and cucumbers (Kidoglu et al. Citation2008) and barley (Cakmakci et al. Citation2007). Few examples of PGPR secreting phytohormones exist, while the roles of gibberellins producing PGPR have been poorly understood.
Cucumber (Cucumis sativus L.) belongs to family Cucurbitaceae, and is a popular cultivated vegetable. In South Korea, it is an important part of the daily meal and used as salad and in traditional recipe such as Kimchee and soups. To obtain higher production, the farmers use various organic fertilizers with irrigation. This can lead to marked deterioration in soil and ground water quality as well as reduce the soil fertility, thus catering to an unsustainable agricultural system (Bharti et al. Citation2013). However, use of bio-fertilizers containing beneficial microorganisms instead of synthetic chemicals is known to improve plant growth and development and may help to sustain environmental health and soil productivity (Cakmakci et al. Citation2007). The use of PGPR as bio-fertilizers or control agents for agriculture improvement has been a focus of numerous researchers for a number of years (Kang et al. Citation2010). The aim of the present study was to assess the role of novel PGPR strains namely Burkholdera cepacia SE4, Promicromonospora sp. SE188, and Acinetobacter calcoaceticus SE370 to counteract salinity and drought induced abiotic stress on cucumber plants. We have also determined the endogenous hormonal regulation (abscisic acid – ABA, gibberellin – GA4, and salicylic acid – SA) and oxidative damages to the plants treated with or without PGPR during abiotic stresses.
Materials and methods
PGPR interactions and stress application
Experimental design contains six sets of cucumber (C. sativus L) plants with (1) PGPR interactions; (2) non-PGPR interactions; (3) PGPR interactions salinity (sodium chloride – NaCl); (4) non-PGPR interactions salinity; (5) PGPR interactions drought (polyethylene glycol – 15% PEG) and (6) non-PGPR interactions drought. B. cepacia SE4 (FJ445745), Promicromonospora sp. SE188 (KCTC 11096BP), A. calcoaceticus SE370 (KCTC11095BP) were assessed for their potential role to resist salt and drought stress. Cucumber seeds before sowing in autoclaved pots were surface sterilized with 1% Tween 80 solution, 2% perchloric acid and autoclaved double distilled water (DDW) for 5 minutes in shaking incubator (120 rpm) and germinated. Plants were grown in the green house (30 ± 2°C) for 3 weeks (27 plants per treatment) and irrigated with distilled water or salt or PEG solution.
One-week-old cucumber seedlings were treated with 5 mL of bacterial culture suspension. The cucumber plants were sown in soil substrate comprised peat moss (13–18%), perlite (7–11%), coco-peat (63–68%) and zeolite (6–8%) with macro-nutrients present as: NH4+ ∼0.09 mg g−1; NO3− ∼0.205 mg.g−1; P2O5 ∼0.35 mg.g−1and K2O ∼0.1 mg.g−1 (Kang et al. Citation2010). After 21 days of bacteria and plant growth in the pots, salt and drought stress was applied for 7 continuous days. NaCl solution (1400 mL for 7 days) was used for induction of high salt stress (120 mM). Similarly, drought stress was initiated by exposing plants to polyethylene glycol (15% PEG solution; 1400 mL solution for 7 days).
The growth parameters, that is, shoot length; shoot fresh and dry weights were measured for harvested cucumber plants, while chlorophyll content of fully expanded leaves was analyzed with the help of chlorophyll meter (SPAD-502 Minolta, Japan). Dry weights were measured after drying the plants at 70°C for 72 h in an oven.
Determining leaf water potential
Leaf relative water content (RWC) was measured according to (Gonzalez & Gonzalez-Vilar Citation2003). Individual leaves were first removed from stem and immediately weighed (fresh mass, FM). In order to determine the turgid mass (TM), leaves were floated in distilled water inside a closed petri dish. During the imbibition period, leaves were weighed periodically after water on the leaf surface was gently wiped with tissue paper. At the end of the imbibition periods, leaves were placed in a pre-heated oven at 70°C for 48 h, in order to obtain dry mass (DM). All mass measurements were made at a precision of 0.0001 g. Values of FM, TM and DM were used to calculate leaf RWC using the following equation:
Measuring electrolytic leakage from the leaves
Electrolytic leakage (EL) was determined on a prescribed method by Gonzalez and Gonzalez-Vilar (Citation2003). To determine electrolyte leakage, fresh leaf samples (200 mg) were cut into 5 mm lengths and placed in test tubes containing 10 mL distilled deionized water. The tubes covered with plastic caps were placed in a water bath at a constant temperature of 32°C. After 2 h the initial electrical conductivity of the medium (EC1) was measured using an electrical conductivity meter. The samples were autoclaved afterward at 121°C for 20 min to completely kill the tissues and release all electrolytes. The samples were then cooled to 25°C and the final electrical conductivity (EC2) measured. The EL was estimated using the following formula: EL = EC1/EC2 × 100.
Endogenous Gibberellin (GA4) analysis
Endogenous gibberellin (GA) were extracted from 0.5 g of freeze-dried aerial part of cucumber plants treated with PGPR, salt, and drought stresses. The method used for extraction and quantification of endogenous gibberellins was based on the already established procedure of Lee et al. (Citation1998). A 0.5 gm lyophilized sample was used for GA analysis each time. The gas chromatography (GC) (Hewlett-Packard 6890, 5973N Mass Selective Detector) with HA-1 capillary column (30 m × 0.25 mm i.d. 0.25 µm film thickness) oven temperature was programmed for 1 min at 60°C, then a rise of 15°C min−1 to 200°C, followed by 5°C min−1 to 285°C. Helium carrier gas was maintained at a head pressure of 30 kPa. The GC was directly interfaced to a Mass Selective Detector with an interface and source temperature of 280°C, an ionizing voltage of 70 eV and a dwell time of 100 ms. Full scan mode (the first trial) and three major ions of the supplemented [17, 17-2H2]-GA4 internal standards (the second trial) and the endogenous gibberellins were monitored simultaneously (standard GAs were purchased from Prof. Lewis N. Mander, Australian National University, Canberra, Australia). The endogenous GA contents of GA4 was calculated from the peak area ratios of 506/508, 284/286, 298/300, and 418/420, respectively. The data was calculated in nano-grams per gram dry weight and the analysis was repeated three times, using a different sample each time.
Endogenous ABA quantification
Endogenous ABA was extracted from 0.5 g of freeze-dried aerial part of cucumber plants after stress and PGPR treatment. The endogenous ABA contents were extracted following the method of Qi et al. (Citation1998). The extracts were dried and methylated by adding diazomethane for gas chromatography mass spectrometer with selected ion monitoring (6890N network GC system, and 5973 network mass selective detector; Agilent Technologies, Palo Alto, CA, USA) analysis. For quantification, the Lab-Base (ThermoQuset, Manchester, UK) data system software was used to monitor responses to ions of m/e 162 and 190 for Me-ABA and 166 and 194 for Me-[2H6]-ABA.
SA extraction and quantification
SA was extracted and quantified as described previously (Seskar et al. Citation1998). Briefly, freeze-dried aerial tissue (0.5 g) of cucumber plant samples was ground to powder form and was sequentially extracted with 90 and 100% methanol by centrifuging at 10,000×g. The combined methanol extracts was vacuum dried. Dry pellets were re-suspended in 2.5 mL of 5% trichloroacetic acid the supernatant was partitioned with ethyl acetate:cyclopentane:isopropanol (100:99:1, v/v). The top organic layer containing free SA was transferred to a 4 mL vial and dried with nitrogen gas. The dry SA was again suspended in 1 mL of 70% methanol. High Performance Liquid Chromatography (HPLC) analysis were carried out on Shimadzu having fluorescence detector (Shimdzu RF-10AXL, excitation and emission 305–365 nm, respectively) fitted with C18 reverse-phase HPLC column (high performance hypersil octadecylsilyl groups, particle size 5 µm, pore size 120 Å Waters). The flow rate was 1.0 mL/min (Table S1Footnote1).
Measuring leaf total polyphenol contents
Total polyphenol contents were determined by the Folin–Ciocalteau colorimetric method as described by Kumazawa et al. (Citation2004). Aerial parts (100 mg) were ground with 80% ethanol, and extracts (0.5 mL) were mixed with 0.5 mL of the Folin–Ciocalteau reagent and 0.5 mL 10% Na2CO3, and the absorbance was measured at 760 nm after 1 h incubation at room temperature. Total polyphenol contents were expressed as microgram milligram (gallic acid equivalents).
Protein extraction and catalase (CAT) activity
To extract plant proteins, aerial tissues (100 mg) were homogenized in 50 mM Tris–hydrochloride buffer (pH 7.0) containing 3 mM MgCl2, 1 mM ethylenediaminetetraacetic acid, and 1.0% polyvinylpyrrolidone and then centrifuged at 2500×g for 15 min at 4°C; the supernatant was used for biochemical analysis. All parameters were expressed as activity per milligram protein. Catalase activity was assayed by the method of Aebi (Citation1984). The crude enzyme extract was treated with 0.5 mL 0.2 M H2O2 in 10 mM phosphate buffer (pH 7.0). Catalase activity was estimated by the decrease in absorbance of H2O2 at 240 nm, and 1 U of CAT was defined as micrograms of H2O2 released per milligram protein per minute.
Determining peroxidase (POD) and polyphenoloxidase activity
Peroxidase and polyphenoloxidase activity were measured as described by Kar and Mishra (Citation1976) with some modification. The aerial tissues (100 mg) were homogenized with phosphate buffer pH 6.8 (0.1 M) and centrifuged at 2°C for 15 min at 17,000×g in a refrigerated centrifuge. The clear supernatant was analyzed for enzyme activity. The assay mixture for the POD activity comprised 0.1 M phosphate buffer (pH 6.8), 50 µl pyrogallol, 50 µl H2O2 and 0.1 mL enzyme extract. The mixture was incubated for 5 min at 25°C after which the reaction was stopped by adding 0.5 mL 5% (v/v) H2SO4. The amount of purpurogallin formed was determined by the absorbance at 420 nm. The same assay mixture as that of POD without H2O2 was used to assay the activity of polyphenoloxidase. The absorbance of the purpurogallin formed was taken at 420 nm. One unit of POD and polyphenol oxidase was defined as an increase of 0.1 U of absorbance.
Quantification of Na ion and essential nutrients
The aerial parts (100 mg) of all treated plant samples in freeze-dried form were subjected to inductively coupled plasma spectrophotometer (ICP; Optima 7900DV, Perkin–Elmer, USA) analysis to assess the essential ion generation during stress. The contents of Na, K and phosphorus (P), potassium (K) and sodium (Na) were determined by ICP.
Statistical analysis
To know the effect of PGPR, culture application on plants in all the sets of experiments was performed in a completely randomized block design. Each experiment (ABA, SA, growth attributes, EL, and RWC) was repeated three times except GAs. The differences among the mean values were determined using Duncan's multiple range tests (DMRTs) at P < 0.05. The results were graphically presented using Graph Pad Prism software (version 5.0, San Diego, California USA) while Statistic Analysis System (SAS 9.1) for DMRT analysis.
Results
Plant growth dynamics under PGPRs and abiotic stresses
The effect of PGPR application on the cucumber plant growth and its allied attributes were assessed under salinity and drought stress. The results showed that the application of PGPR (B. cepacia, A. calcoaceticus and Promicromonospora sp.) significantly increased the shoot and root growth as compared to sole culture medium-applied control plants. The shoot length was significantly higher during sodium chloride (NaCl) induced salinity stress as compared to control cucumber plants. The three PGPRs inoculation exhibited a similar pattern of shoot growth under both normal and salinity stressed conditions (). The shoot length was 28.5, 26.1 and 26.9% higher in PGPR B. cepacia, Promicromonospora sp. and A. calcoaceticus application to the cucumber plants, respectively, as compared to control under normal conditions. Upon salinity stress, the shoot growth was 21.3, 20.3 and 18.7% higher in B. cepacia, Promicromonospora sp. and A. calcoaceticus treatments, respectively, as compared to control (). Among PGPRs, the growth-promoting effect of B. cepacia SE4 application was more significant as compared to other strains ().
Table 1. Effect of salt stress on the growth attributes of the cucumber plants with and without PGPRs inoculation.
The cucumber plants exposed to polyethylene glycol (15% PEG) induced drought stress. The PGPRs inoculation helped the cucumber plants in tolerating drought stress but also rescued plant growth and development. Upon osmotic stress, the shoot growth was 15.4, 12.3 and 14.4% higher in B. cepacia, Promicromonospora sp. and A. calcoaceticus application, respectively, as compared to control. Similarly, when the cucumber plants exposed to drought stress, the B. cepacia, A. calcoaceticus and Promicromonospora sp. inoculated plants had higher shoot fresh and dry weight and chlorophyll contents () under normal growth conditions. However, under drought stress condition, all the three PGPRs have significantly ameliorated the adverse effects (). The effect of B. cepacia was more pronounced than that of other two PGPRs.
Leaf water potential during PGPR application and stress
Exposure to salinity greatly influences plant water potential. The non-inoculated control plants had significantly lower leaf water status as compared to PGPR (B. cepacia, A. calcoaceticus and Promicromonospora sp.) application during normal growth conditions. Relative water content of control plants were 13.1, 8.6 and 5.7% lower than the B. cepacia, Promicromonospora sp. and A. calcoaceticus inoculated plants, respectively. However, this effect was more pronounced in non-inoculated control plants under salinity. Although, when salinity and drought stress were induced, the non-inoculated control plants were significantly deficient in their water potential as compared to the B. cepacia, A. calcoaceticus and Promicromonospora sp. inoculated cucumber plants (). The PGPR B. cepacia, A. calcoaceticus and Promicromonospora sp. application resulted in increased leaf water potential of 10.0, 9.8 and 11.2%, respectively, under salinity stress. A similar trend was observed in drought stress.
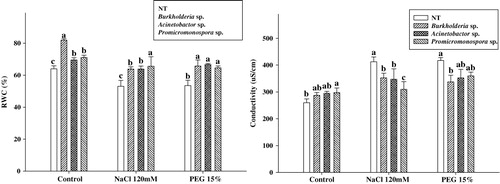
Leaf electrolytic leakage during PGPR and stress
Inoculating the cucumber plants with B. cepacia, A. calcoaceticus and Promicromonospora sp. resulted in a higher level of electrolytic release in comparison to control under normal growth conditions. However, when the cucumber plants were exposed to salinity and drought stress condition, the electrolytic leakage was more prominent in non-inoculated control plants than B. cepacia, A. calcoaceticus and Promicromonospora sp. treated cucumber plants (). The control plants had approximately 21.0 and 18.2% higher electrolytes release from leaves tissue as compared to PGPR-applied ones under salinity and drought conditions, respectively. In conclusion, the control plant suffered from more membrane injury as compared to PGPRs-applied cucumber plants.
Phytohormonal regulation under PGPR and abiotic stress
Endogenous phytohormonal regulation was assessed during PGPRs and stress application to cucumber plants. The results showed that stress-responsive ABA content in the B. cepacia, A. calcoaceticus and Promicromonospora sp. PGPRs-applied plants were significantly lower as compared to control. The control plants had 27% higher ABA contents as compared to PGPR applications. The same was observed in the salinity and drought stress. However, the ABA contents increased exponentially under stress in control plants, while this increase was not sharp in PGPR-applied plants. The ABA contents in control plants were 145 and 94% higher in salinity and drought stress, respectively, as compared to PGPR applications. This suggests the down-regulation of ABA responses during B. cepacia, A. calcoaceticus and Promicromonospora sp. treatments ().
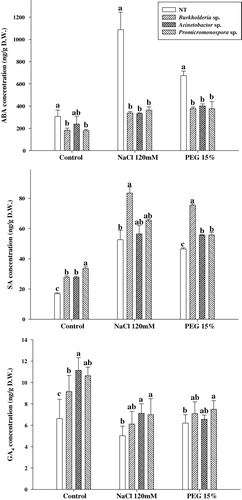
Contrary to ABA, the endogenous SA content was significantly higher in B. cepacia, A. calcoaceticus and Promicromonospora sp. inoculated plants than non-inoculated plants under normal growth conditions. The increase observed in PGPR treatments was ∼1.5-fold as compared to control. The same trend was repeated, while the increase in SA content was more pronounced in stress conditions. This increase was 0.7- to 1.4-fold higher than the control during salinity and drought stress under PGPR applications. The results reveal up-regulation of SA synthesis pathway under PGPR and stress conditions ().
In case of gibberellin (GA4), in normal growth conditions, the PGPR-applied plants had significantly higher endogenous GAs as compared to the medium and distilled water (DW)-treated cucumber plants. During the excursion of abiotic salinity stress, the endogenous GAs was significantly higher in PGPR-treated plants as compared to control plants (). The same trend was revisited in the drought stress ().
Effect of PGPR application on oxidative stress enzyme
The antioxidant enzymes, CAT, POD, polyphenol peroxidase (PPO), and antioxidant (total polyphenols) were assessed in cucumber plants after salinity and drought stress, while inoculating with PGPR B. cepacia, A. calcoaceticus and Promicromonospora sp. The results showed that CAT activity was slightly decreased in B. cepacia, A. calcoaceticus and Promicromonospora sp. treated plants under normal conditions. However, in control plants, the CAT activity was significantly higher in salinity and drought stress as compared to the plant treated with the combination of PGPRs and stress (). The activity of CAT was two-fold higher in control under salinity, while it was 0.5-fold higher under drought stress. However, the activity of CAT increased distinctly under stress as compared to non-stressed conditions.
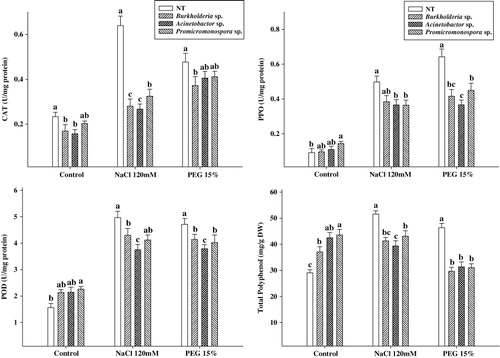
A similar effect was observed in case of POD and PPO activities. The PPO activity was significantly reduced in under stress. The control plants had approximately 0.4- to 0.9-fold higher activities under both salinity and drought stress as compared to PGPR-applied plants. Conversely, the POD activity was significantly higher in the PGPR treatments under normal growth conditions as compared to control; however, this response was antagonistic under salinity and drought stress.
Total polyphenol increased in PGPR inoculation under normal condition; however, after the excursion of salinity and drought stress, the content decreased significantly as compared to the PGPR-Free cucumber plants (). The reduced level of oxidative stress enzymes suggest a low level of stress convened to the PGPR-applied plants.
PGPR affect the sodium ion toxicity and K, P concentrations
The ICP analysis showed that the potassium (K) and phosphorus (P) ion concentrations were significantly higher in PGPR application as compared to non-PGPR-applied plants. When the plants were exposed to salinity stress, the Na accumulation was significantly lower in PGPR-treated plants as compared to control plants, suggesting that the high level of resistance to negative effects of sodium (Na) ions. Similarly, plant treated with drought stress, the P and K ions were significantly higher in PGPR-applied plants as compared to control plants (). The toxicity of Na ion was greatly minimized by the active role of PGPRs during both salinity and drought stress.
Table 2. Ion concentration in the shoots of stress-treated cucumber plants inoculated and non-inoculated with PGPR. Values are ×103 mg kg−1.
Discussion
PGPR plays an essential role in improving crop growth during difficult growth circumstances. We have found that our isolated PGPRs strains (B. cepacia, A. calcoaceticus and Promicromonospora sp.) have ameliorative effects on cucumber plants growth. These strains improved the cucumber plant growth under stress conditions to maintain its growth during sodium chloride and polyethylene glycol induced salinity and drought stress. Similar growth-promoting and stress tolerance effects were also observed by Rakshapal et al. (Citation2013). His results showed that Pseudomonas monteilii, Cronobacter dublinensis, and Bacillus sp. inoculation to Ocimum basilicum L. increased the nutrient uptake and reduced the antagonistic effects of abiotic stress. Another PGPR Azospirillum lipoferum has been found to increase maize growth, while accumulating free amino acids, soluble sugars, and proline during drought stress (Qudsaia et al. Citation2013). PGPRs species like Azospirillum sp. and Pseudomonas sp. increased the growth and biomass of canola plants by regulating the oxidative stress enzymes and essential nutrient under salinity stress (Noorieh et al. Citation2013). Recently, Anna et al. (Citation2013) isolated bacterial endophytes from the roots of tomato plants. These bacterial strains (Azospirillum brasilense, Gluconacetobacter diazotrophicus, Herbaspirillum seropedicae, and Burkholderia ambifaria) not only increased the growth of tomato plants but also helped in atmospheric nitrogen fixation to increase host's resistance against lateral pathogens. Recently, PGPRs like Mesorhizobium sp. and Pseudomonas aeruginosa have been shown to produce indole acetic acid. Both the PGPRs were found to increase the growth of chickpea plants while supporting host plants in P, and K uptake (Jay et al. Citation2013). PGPR strains from Azotobacter increased the maize plant growth and potassium and phosphorus intake under different levels of salinity stress (Rojas-Tapias et al. Citation2012).
Salinity causes an imbalance in the ion flux inside plants. The present results showed that during salinity, the control plants had higher Na+ and decreased K+, while PGPR inoculation resulted in significantly decreased Na+ and increased K+ concentration. This is also according to the results of (Rojas-Tapias et al. Citation2012). Increased ionic flux can damage the plant cellular membranes and effect water potential of the plant's cell (Hussain et al. Citation2008). Plant growth is dependent on water status of leaf, as salt and drought stress can create a water deficit inside plant tissues. Measuring RWC indicates stress response of plant (Gonzalez & Gonzalez-Vilar Citation2003). The RWC of PGPR-treated plants was observed to be higher than that of control during salinity stress. Thus, showing the beneficial association can undermine such stresses. Our findings are confirmatory to other studies (Rakshapal et al. Citation2013) which suggest that the PGPR-inoculated plants not only reduce stress but also help to fetch higher water quantity from sources inaccessible to control plants. Environmental stresses can establish higher electrolytes discharge (like K ions) through displacement of membrane-associated Ca from plasma lemma. Resultantly, membrane permeability is damaged and aggregate higher efflux of electrolytes inside plant cell/tissues (Garg & Manchanda Citation2009). The findings of the present study have shown that plants with PGPR association have lower electrolytic concentrations than control plants under salinity and drought stress. This indicates a lower permeability of the plasma membrane mainly attributed to the integrity and stability of cellular tissues by the PGPR interactions compared with non-PGPR interactions (Garg & Manchanda Citation2009).
Protective effect of ABA is pivotal in plant growth as it promotes stomatal closure to minimize water loss and mediates stress damage through activation of many stress-responsive genes, which collectively increases the plant's stress tolerance (Zhang et al. Citation2006). It has been widely described that ABA contents in plants increase under salt and drought stress (Wang et al. Citation2001). However, the findings of our study showed significantly lower ABA production in the presence of PGPR interaction compared to non-PGPR interaction. The ABA results of drought stress were no different from salt stress. As the role of ABA is regulation of signaling pathways involved in plant growth and development, both of them might have been affected by the presence of PGPR interaction during abiotic stress; although, other studies suggest that PGPR inoculation can increase the ABA accumulation in leaves and roots as compared to non-inoculation control plants (Herrera-Medina et al. Citation2007). However, the effect may fluctuate among different classes of microorganisms and plant species as some earlier reports have elaborated (Evelin et al. Citation2009; Yang & Crowley Citation2000). There have been several studies, which narrate the same findings of low ABA levels under stress and PGPR inoculation. Similarly, some reports elucidated low level of ABA contents after exogenous GA3 (Hamayun et al. Citation2010; Iqbal & Ashraf Citation2013) in NaCl induced salinity stress. Higher ABA in salt stress is correlated to inhibition of leaf expansion and shoots development in different species; however, PGPR-treated plants counteracted adverse effects of stress by significantly increasing leaf area and producing a low level of ABA compared control plants. It has been shown that abiotic stress activates a broad range of genes with multiple functions and provokes both ABA dependent and independent responses (Shinozaki & Yamaguchi-Shinozaki Citation1997). It has been evidenced that under PGPR association ABA showed altered levels.
SA on the other hand induces systemic acquired resistance against pathogenic attack in non-infected plant's parts. However, under mutualistic relationship SA production initiates induced systemic resistance and improves plant performance in both biotic and abiotic stresses (Pozo & Azcón-Aguilar Citation2007). Recently, Alonso-Ramírez et al. (Citation2009) have reported that GA-responsive gene and exogenous addition of GAs are able to counteract the inhibitory effects of different adverse environmental conditions in seed germination and seedling growth of Arabidopsis through modulation of SA biosynthesis. A similar trend has also been observed in other researchers (Hamayun et al. Citation2010; Iqbal & Ashraf Citation2013). Thus, the enhanced SA levels have not only induced systemic resistance to the plants but also help the plant to mediate the adverse effects of salinity stress.
GAs are plant growth hormones specialized for plant growth and development, while ABA is a well-known plant stress hormone. Our study showed that application of A. calcoaceticus significantly promoted endogenous bioactive GA1 and GA4 and their immediate precursors. Presence of GA1 and GA4 showed that both early C-13 hydroxylation and non C-13 hydroxylation pathways are operational in cucumber, while a significantly higher amount of GA4 suggests that the major GA biosynthesis pathway in cucumber is the non C-13 hydroxylation pathway. On the other hand, ABA contents of cucumber plants treated with A. calcoaceticus significantly lowered as compared to NB and DW treatments. It is well understood that ABA contents increase in response to abiotic stress and can inhibit plant growth by lowering leaf area and shoot length (Kang et al. Citation2010). A decrease in ABA contents in A. calcoaceticus inoculated plants suggests favorable environment provision by PGPR.
These ameliorative effects of PGPRs can be due to their ability to secret biologically active secondary metabolites including phytohormones. Previously, B. cepacia, A. calcoaceticus and Promicromonospora sp. were found to produce gibberellins and auxins during their growth. Such bioactive PGPRs can extend additional support to plant growth during abiotic stresses as shown by other studies of Asghar et al. (Citation2002), Lugtenberg and Kamilova (Citation2009), Noorieh et al. (Citation2013) and Rakshapal et al. (Citation2013). While the exogenous phytohormones have already been identified to ameliorate plant growth and development during abiotic stresses (Hamayun et al. Citation2010; Iqbal & Ashraf Citation2013; Kang et al. Citation2014). This is in correlation with our findings as well. The present growth stimulatory effects were due to their potential to produce gibberellins (Kang et al. Citation2009, Citation2010, Citation2014). In conclusion, such phytohormones producing PGPRs can be applied to crops to increase their productivity, as well as their association will reduce the negative impacts of salinity and short-term drought periods.
Supplementary_Material.doc
Download MS Word (26.5 KB)Acknowledgment
This study was supported by Korea Ministry of Environment through ‘The Eco-Innovation Project’.
Notes
1. Supplemental Content may be viewed online at http://dx.doi.org/10.1080/17429145.2014.894587
References
- Adesemoye AO, Torbert HA, Kloepper JW. 2009. Plant growth promoting rhizobacteria allow reduced application rates of chemical fertilizers. Microb Ecol. 58:921–929. 10.1007/s00248-009-9531-y
- Aebi H. 1984. Catalase in vitro. Methods Enzymol. 105:121–126.
- Alonso-Ramírez A, Rodrıguez D, Reyes D, Jimenez JA, Nicolas G, Lopez-Climent M, Gomez-Cadenas A, Nicolas C. 2009. Evidence for a role of gibberellins in salicylic acid-modulated early plant responses to abiotic stress in Arabidopsis seeds. Plant Physiol. 150:1335–1344. 10.1104/pp.109.139352
- Anna LB, Alessandra S, Claudia E, Paola C, Maddalena DG. 2013. In vitro and in vivo inoculation of four endophytic bacteria on Lycopersicon esculentum. New Biotechnol. 30:666–674. 10.1016/j.nbt.2013.01.001
- Asghar HN, Zahir ZA, Arshad M, Khaliq K. 2002. Relationship between in vitro production of auxins by rhizobacteria and their growth-promoting activities in Brassica juncea L. Biol Fertil Soils. 35:231–237. 10.1007/s00374-002-0462-8
- Bartels D, Sunkar R. 2005. Drought and salt tolerance in plants. Crit Rev Plant Sci. 24:23–58. 10.1080/07352680590910410
- Bharti N, Yadav D, Barnawal D, Maji D, Kalra A. 2013. Exiguobacterium oxidotolerans, a halotolerant plant growth promoting rhizobacteria, improves yield and content of secondary metabolites in Bacopa monnieri (L.) Pennell under primary and secondary salt stress. World J Microbiol Biotechnol. 29:379–387. 10.1007/s11274-012-1192-1
- Burd G, Dixon DG, Glick BR. 2000. Plant growth promoting bacteria that decrease heavy metal toxicity in plants. Can J Microbiol. 46:237–245. 10.1139/w99-143
- Cakmakci R, Donmez MF, Erdogan U. 2007. The effect of plant growth promoting rhizobacteria on barley seedling growth, nutrient uptake, some soil properties, and bacterial counts. Turk J Agric For. 31:189–199.
- Chaiharn M, Chunhaleuchanon S, Kozo A, Lumyong S. 2008. Screening of rhizobacteria for their plant growth promoting activities. KMITL Sci Technol J. 8:18–23.
- Christensen JH, Hewitson B, Busuioc A, Chen A, Gao X, Held I, Jones R, Kolli RK, Kwon W-T, Laprise R, et al. 2007. Regional climate projections. In: Solomon S, Qin D, Manning M, Chen Z, Marquis M, Averyt KB, Tignor M, Miller HL, editors. Climate change 2007: The physical science basis. Contribution of working group I to the fourth assessment report of the intergovernmental panel on climate change. Cambridge, UK and New York, NY: Cambridge University Press; p. 848–940.
- Evelin H, Kapoor R, Giri B. 2009. Arbuscular mycorrhizal fungi in alleviation of salt stress: a review. Ann Bot. 104:1263–1280. 10.1111/j.1439-037X.2008.00349.x
- Falkenmark M. 2013. Growing water scarcity in agriculture: future challenge to global water security. Philos Trans R Soc A. 371:20120410. 10.1098/rsta.2012.0410
- Garg N, Manchanda G. 2009. Role of arbuscular mycorrhizae in the alleviation of ionic, osmotic and oxidative stresses induced by salinity in Cajanus cajan (L.) Millsp. (pigeonpea). J Agron Crop Sci. 195:110–123. 10.1111/j.1439-037X.2008.00349.x
- Gonzalez L, Gonzalez-Vilar M. 2003. Determination of relative water content. In: Reigosa MJ, editor. Handbook of plant ecophysiology techniques. Dordrecht: Kluwer Academic; p. 207–212.
- Hamayun M, Khan SA, Khan AL, Shin JH, Lee IJ. 2010. Exogenous gibberellic acid reprograms soybean to higher growth, and salt stress tolerance. J Agric Food Chem. 58:7226–7232. 10.1021/jf101221t
- Herrera-Medina MJ, Steinkellner S, Vierheilig H, Bote JAO, Garrido JMG. 2007. Abscisic acid determines arbuscule development and functionality in the tomato arbuscular mycorrhiza. New Phytologist. 175:554–564. 10.1111/j.1469-8137.2007.02107.x
- Hussain TM, Chandrasekhar T, Hazara M, Sultan Z, Saleh BK, Gopal GR. 2008. Recent advances in salt stress biology – a review. Biotechnol Mol Biol Rev. 3:8–13.
- Iqbal M, Ashraf M. 2013. Gibberellic acid mediated induction of salt tolerance in wheat plants: growth, ionic partitioning, photosynthesis, yield and hormonal homeostasis. Environ Exp Bot. 86:76–85.
- Jay PV, Janardan Y, Kavindra NT, Ashok K. 2013. Effect of indigenous Mesorhizobium spp. and plant growth promoting rhizobacteria on yields and nutrients uptake of chickpea (Cicer arietinum L.) under sustainable agriculture. Ecol Eng. 51:282–286. 10.1016/j.ecoleng.2012.12.022
- Kang SM, Hamayun M, Joo GJ, Khan AL, Kim YH, Kim SK, Jeong HJ, Lee IJ. 2010. Effect of Burkholderia sp. KCTC 11096BP on some physiochemical attributes of cucumber. Eur J Soil Biol. 46:264–268. 10.1016/j.ejsobi.2010.03.002
- Kang SM, Joo GJ, Hamayun M, Na CI, Shin DH, Kim H, Hong JK, Lee IJ. 2009. Gibberellin production and phosphate solubilization by newly isolated strain of Acinetobacter calcoaceticus and its effect on plant growth. Biotechnol Lett. 31:277–281. 10.1007/s10529-008-9867-2
- Kang SM, Waqas M, Khan AL, Lee IJ. 2014. Plant growth promoting rhizobacteria: potential candidates for gibberellins production and crop growth promotion. In: Miransari M, editor. Use of microbes for the alleviation of soil stresses. New York: Springer; p. 1–19.
- Kar M, Mishra D. 1976. Catalase, peroxidase, and polyphenoloxidase activities during rice leaf senescence. Plant Physiol. 57:315–319. 10.1104/pp.57.2.315
- Khalid A, Arshad M, Zahir ZA. 2004. Screening plant growth-promoting rhizobacteria for improving growth and yield of wheat. J Appl Microbiol. 96:473–480. 10.1046/j.1365-2672.2003.02161.x
- Kidoglu F, Gül A, Ozaktan H, Tüzel Y. 2008. Effect of rhizobacteria on plant growth of different vegetables. Acta Hortic. 801:1471–1477.
- Kumazawa S, Hamasaka T, Nakayama T. 2004. Antioxidant activity of propolis of various geographic origins. Food Chem. 84:329–339. 10.1016/S0308-8146(03)00216-4
- Lee IJ, Foster K, Morgan PW. 1998. Photoperiod control of gibberellin levels and flowering in sorghum. Plant Physiol. 116:1003–1011. 10.1104/pp.116.3.1003
- Lugtenberg B, Kamilova F. 2009. Plant-growth-promoting rhizobacteria. Annu Rev Microbiol. 63:541–556. 10.1146/annurev.micro.62.081307.162918
- Noorieh B, Arzanesh MH, Mahlegha G, Maryam S. 2013. The effect of plant growth promoting rhizobacteria on growth parameters, antioxidant enzymes and microelements of canola under salt stress. J Appl Environ Biol Sci. 3:17–27.
- Pozo MJ, Azcón-Aguilar C. 2007. Unravelling mycorrhiza induced resistance. Curr Opin Plant Biol. 10:393–398. 10.1016/j.pbi.2007.05.004
- Qi QC, Rose PA, Abrams GD, Taylor DC, Abrams SR, Cutler AJ. 1998. Abscisic acid metabolism, 3-ketoacyl-coenzyme a synthase gene expression and very-long-chain monounsaturated fatty acid biosynthesis in Brassica napus embryos. Plant Physiol. 117:319–339. 10.1104/pp.117.3.979
- Qudsaia B, Noshinil Y, Asghari B, Nadia Z, Abida A, Fayazul H. 2013. Effect of Azospirillum inoculation on maize (Zea mays L.) under drought stress. Pak J Bot. 45:13–20.
- Rakshapal S, Sumit KS, Rajendra PP, Alok K. 2013. Technology for improving essential oil yield of Ocimum basilicum L. (sweet basil) by application of bioinoculant colonized seeds under organic field conditions. Indian Crop Prod. 45:335–342. 10.1016/j.indcrop.2013.01.003
- Rojas-Tapias D, Moreno-Galván A, Pardo-Díaz S, Obando M, Rivera D, Bonilla R. 2012. Effect of inoculation with plant growth-promoting bacteria (PGPB) on amelioration of saline stress in maize (Zea mays). Appl Soil Ecol. 61:264–272. 10.1016/j.apsoil.2012.01.006
- Saharan BS, Nehra V. 2011. Plant growth promoting rhizobacteria: a critical review. Life Sci Med Res. 20:1–30.
- Seskar M, Shulaev V, Raskin I. 1998. Endogenous methysalicylate in pathogen-inoculated tobacco plants. Plant Physiol. 116:387–392. 10.1104/pp.116.1.387
- Shinozaki K, Yamaguchi-Shinozaki K. 1997. Gene expression and signal transduction in water-stress response. Plant Physiol. 115:327–334. 10.1104/pp.115.2.327
- Wang Y, Mopper S, Hasenstein KH. 2001. Effects of salinity on endogenous ABA, IAA, JA, and SA in Iris hexagona. J Chem Ecol. 27:327–342. 10.1023/A:1005632506230
- Yang CH, Crowley DE. 2000. Rhizosphere microbial community structure in relation to root location and plant iron nutritional status. Appl Environ Microbiol. 66:345–351. 10.1128/AEM.66.1.345-351.2000
- Yang J, Kloepper JW, Ryu C-M. 2009. Rhizosphere bacteria help plants tolerate abiotic stress. Trends Plant Sci. 14:1–4.
- Zhang J, Jia W, Yang J, Ismail AM. 2006. Role of ABA in integrating plant responses to drought and salt stresses. Field Crop Res. 97:111–119. 10.1016/j.fcr.2005.08.018