Abstract
The study aimed to identify and select important plant growth-promoting rhizobacteria (PGPR) and examine the response of tomato growth upon inoculation. Inoculation with rhizobacterial isolates increased all the measured physical, chemical, and enzymatic growth parameters compared to control. However, the TAN1 isolate had the highest effect, and significantly (P < 0.05) increased the root length (8.25-fold), root fresh (8.36-fold) and dry (12.6-fold) weight, shoot length (6.92-fold), shoot fresh (7.18-fold) and dry (6.90-fold) weight, number of leaves (11.0-fold), chlorophyll a (6.25-fold), chlorophyll b (10.7-fold), carotenoid contents (8.80-fold), seedlings fresh (9.0-fold) and dry (8.71-fold) weight, plant macronutrient uptake, i.e. N (7.7- and 8.9-fold), P (10.5- and 11.4-fold), K (7.8- and 8.8-fold), Ca (12.7- and 8.2-fold), and Mg (12.6- and 9-fold) in shoot and root, plant micronutrient uptake, i.e. Zn (6.6-, 10.2-), Cu (9.3-, and 10.3-fold), Fe (7.7- and 10.7-fold), and Mn (4.7- and 5.7-fold) in shoot and root and plant antioxidant enzymes, i.e. glutathione S-transferase (10.7-fold), peroxidase (8.1-fold), and catalase (10.5-fold). Our results concluded that inoculation of agricultural crops with rhizobacteria is a very useful approach to increase the plant growth. The rhizobacteria having both 1-aminocyclopropane-1-carboxylate (ACC) deaminase and nitrogen-fixing activity are more effective than rhizobacteria possessing either ACC-deaminase or nitrogen-fixing activity alone for growth promotion of crops.
Introduction
Plant growth-promoting rhizobacteria (PGPR) are free living microorganisms having beneficial effects on plants by colonizing their roots (Ahemad Citation2012). PGPR promote the plant growth by several direct and indirect ways (Ahemad Citation2012). Directly, PGPR provide the bacterium-synthesized compounds to the plants, facilitate the nutrient availability and uptake and production of certain phytohormones, e.g. auxin, cytokinins, ethylene, and abscisic acid and giberelins (Ahemad Citation2012). Indirectly, PGPR minimize or prevent the harmful effects of pathogenic organisms (Ahemad & Malik Citation2011), moreover, mobilize the nutrients in soils and improve the soil structure (Ahemad & Malik Citation2011). Inoculation of agricultural crops with PGPR results in multiple positive effects on plant, e.g. increase in the plant growth and vigor, increment in the chlorophyll, protein, proline, and antioxidant enzymes (e.g. peroxidase (POX), catalase, and glutathione reductase) activity that increase the plant immunity (Sahran & Nehra Citation2011). It has been observed that increased activity of antioxidant enzymes is correlated with resistance, against both biotic and abiotic stresses, in many plant species (Jan et al. Citation2011).
At present, the use of microorganisms with the aim of improving crop production on sustainable basis has increased tremendously in various parts of the world (Glick Citation2012). This is due to the emerging demand for dependence diminishing of synthetic chemical products, to the growing necessity of sustainable agriculture, and a holistic vision of environmental protection (Hayat et al. Citation2010; Glick Citation2012). In this regard, the use of PGPR has found a potential role in developing sustainable systems in crop production (Hayat et al. Citation2010). It has been reported that, the bacteria inhabiting around or in the plant roots, e.g. rhizobacteria are more versatile in transforming, mobilizing, and solubilizing the nutrients compared to those from bulk soils (Hayat et al. Citation2010). Rhizobacteria are the dominant deriving forces in recycling the soil nutrients and, consequently, for soil fertility and can play cardinal role in the plant growth and production (Glick Citation2012).
Chemical fertilizers not only nourish plants and microbes but also have harmful effects on the soil and plants life, especially when they are very concentrated and water soluble (Savci Citation2012). In recent years, fertilizer consumption increased (143.88 million nutrient tons) exponentially throughout the world (Savci Citation2012). Excessive fertilization is a potential global threat to the soil and environment through soil salinity, acidification as well as neutralization of the soil, heavy metal accumulation, water eutrophication, and accumulation of nitrate (Savci Citation2012). Therefore, number of scientists worldwide is considering the possibility of supplementing chemical fertilizers with microbial sources (Naveed et al. Citation2008). Therefore, the use of PGPR as a biofertilizer in agricultural ecosystems is a judicious and viable technique.
Ethylene is known as a growth hormone and a stimulator in germination by breaking the dormancy (Khalid et al. Citation2006). However, if the level of ethylene is higher than optimum level, then it acts as a stress hormone (Saleem et al. Citation2007). Zahir et al. (Citation2008) set an experiment to examine the effectiveness of rhizobacteria containing ACC-deaminase on the growth of pea (Pisum sativum) and concluded that rhizobacteria with ACC-deaminase facilitate plant growth by decreasing excessive ethylene levels (Zahir et al. Citation2008). The PGPR with ACC-deaminase take up the ethylene precursor ACC and convert it into 2-oxobutanoate and NH3 (Arshad et al. Citation2007). As a result, the major effects of seed inoculation with ACC-deaminase-producing rhizobacteria are the plant root elongation, promotion of shoot growth, and enhancement in rhizobial nodulation and nitrogen (N), phosphorous (P), and K uptake (Glick Citation2012).
Phytohormone auxin (indole-3-acetic acid/indole acetic acid [IAA]) played a vital role in many plant developmental processes, e.g. plant cell division and differentiation; seed germination; lateral and adventitious root formation; biosynthesis of various metabolites, and plant defense mechanisms (Glick Citation2012). The IAA produced by rhizobacteria likely, interfere the abovementioned physiological processes of plants by changing the plant auxin pool, and thus, rhizobacterial IAA is identified as an effector molecule in plant-microbe interactions, both in pathogenesis and phytostimulation (Spaepen & Vanderleyden Citation2011). An important molecule that alters the level of IAA synthesis is the amino acid tryptophan (L-tryptophan [L-TRP]), identified as the main precursor for IAA and thus plays a role in modulating the level of IAA biosynthesis (Zaidi et al. Citation2009). This suggests that rhizobacterial isolates, which can produce IAA in the presence of L-TRP, can play a vital role in the plant growth.
Among macronutrients N and P are known as most essential and major macronutrients for crops, which plays important roles in plant growth and metabolism (Lal Citation2002; Raymond et al. Citation2004). These macronutrients can be applied through synthetic, i.e. chemical or biological, means, but chemical nitrogenous fertilizers are expensive and health hazardous (Regan Citation1988). Therefore, biological means, e.g. use of bio-fertilizers by N-fixing microorganisms, which are widely distributed in nature are of tremendous potential and importance (Raymond et al. Citation2004). To get vigor, plant growth, strong plant stand, and optimum yields, biological means need to be explored for acquiring ecofriendly and cheap sources of plant nutrients, e.g. N and P. Moreover, the wide-scale application of PGPR may decrease the global dependence on agricultural chemicals, and it is a technology which is easily accessible and adoptable for farmers in both developed and developing countries (Gamalero et al. Citation2009). Thus we can increase the plant growth and yield on sustainable basis by inoculation with PGPR. Keeping in view all these facts, the current study was conducted with the objectives: (1) isolation and selection of important rhizobacterial strains, (2) to examine the physical, chemical, and enzymatic responses of tomato plant to three different rhizobacterial strains, Strain 1: containing ACC-deaminase activity (TACC1 and TACC2), Strain 2: having nitrogen-fixing ability (Azotobacter and TRN1), and Strain 3: having both ACC-deaminase activity and nitrogen-fixing ability (TAN1, TAN2).
Materials and methods
Physiochemical analysis
The soil was collected at 0–5 cm depth with the help of an augur and was transferred into large polyethylene bags to the laboratory. The samples were air-dried and were passed through 2-mm sieve for further use in the incubation study. Soil texture, pH, electrical conductivity (EC), available P, N, extractable K, and organic matter (OM) and saturation percentage were determined by the method of Hassan (Citation2013), Hassan, Akmal et al. (Citation2013), Hassan, Chen, Huang et al. (Citation2013), Hassan, Chen, Cai et al. (Citation2013), Hassan, David, Abbas (Citation2014), Hassan, Chen et al. (Citation2014), Hassan, Bano, Khatak et al. (Citation2014), Hassan, Bano, Bashir et al. (Citation2014) and Hassan and David (Citation2014). Some pertinent characteristics of the soil used in this study are given in .
Table 1. Physicochemical characteristics of experimental soil.
Isolation of PGPR
For isolation of bacterial strains, rhizosphere soil was collected by gentle shaking from tomato crop. Dilution plate technique (Wollum II Citation1982) was used for the isolation of rhizobacteria on Dworken and Foster (DF) salt minimal media (Dworken & Foster Citation1958) containing ACC as a sole N source for PGPR possessing ACC-deaminase activity, and modified mannitol agar media was used to isolate PGPR having N-fixing activity (enrichment technique).
Isolation of rhizobacteria having both abilities
The isolated rhizobacteria were further grown on both (DF salt minimal and modified mannitol agar) media to isolate the rhizobacteria having both N-fixing and ACC-deaminase activity. The isolates having ACC-deaminase activity was grown on modified mannitol agar media used for N-fixing isolates, and the rhizobacteria having N-fixing activity was grown on media that was used for isolates having ACC-deaminase activity. The isolates TAN1 and TAN2 grew on both media and thus having both abilities.
Selection of strains
Three different rhizobacterial strains were selected. Strain 1 rhizobacteria containing ACC-deaminase activity (TACC1 and TACC2) and Strain 2 rhizobacteria possessing N-fixing activity (Azotobacter and TRN1), and Strain 3 rhizobacteria having both ACC-deaminase and N-fixing activities (TAN1 and TAN2).
Preparation of inocula
The already autoclaved 250 ml flasks were used for the preparation of inocula. The autoclaved DF salt minimal media was used for the rhizobacterial isolates containing ACC-deaminase activity. Conversely, modified mannitol agar media was used for the rhizobacterial isolates having N-fixing activity. The flasks were incubated at 28 ± 1°C for 48 h in the orbital shaking incubator at 100 revolutions per minute. The optical density was measured to maintain the uniform population of bacteria in the broth at the time of inoculation.
Characterization of isolates
The method of Davies and Whitbread (Citation1989) was used to calculate the root colonization activity. Fleetingly, inoculated seeds were sown in glass jars containing sterilized sand, and the jars were kept in a growth chamber (18°C, 70% relative humidity, 16 h daylight). After 7 days of germination, the roots were cut off, dipped in phosphate buffer, and were shaken vigorously to remove the bacteria. After 2 days of incubation at 28°C, the number of colonies (CFU/cm) was calculated.
The National Botanical Research Institute’s Phosphate growth medium (NBRIP) was used for the calculation of phosphorus solubilizing activity. The bacterial strains were cultured, and a loop full of each culture was placed on the plates (five per plate) and plates were incubated at 28°C for 7 days. A clear zone around the colonies after 7 days was scored as a positive for phosphate solubilization, whereas phosphate solubilizing index (PSI) of the rhizobacterial isolates was measured by using the following formula as proposed by Premono et al. (Citation1996).
Table 2. Characterization of rhizobacterial strains.
Determination of plant physical parameters
Shoot and root lengths were measured by using a meter rod after careful separation of roots and shoots from the soil. The fresh weight of the shoots and roots was determined with the help of an analytical balance, whereas for the dry weight determination, plants were oven dried at 70°C to a constant dry weight.
Determination of chlorophyll and carotenoid
Acetone extract (80% v/v) was used by the spectrophotometric determination of chlorophyll and carotenoid contents at 663, 645, and 480 nm for chlorophyll a, b, and carotenoids contents, respectively (Arnon Citation1949).
Macro and micronutrients in plant
For the determination of plant nitrogen (N), 0.5 g of dry plant material was taken in 100 ml digestion tube. Then pumic boiling granule and 3 g catalyst mixture were added, immediately followed by 10 ml of concentrated H2SO4 addition. After stirring on vortex, mixer tube was placed at block digester set at 100°C for 20 min, then the volume was brought to 100 ml. Then 10 ml of 10 N NaOH solution was added and distillated at distillation apparatus for 10 min. The 35 ml distillate was collected and titrated against 0.01 N H2SO4 (Van-Schouwenberg & Walinge Citation1973).
For measuring the plant phosphorous (P), the plant material was digested and then 10 ml of digested filtrate was taken in a 100-ml volumetric flask, and 10 ml of ammonium vanadomolybdate reagent was added and solution was diluted. The calibration curve was prepared from standards, and the absorbance for P concentration was plotted, and P concentration was determined from the calibration curve (Buresh et al. Citation1982).
The plant potassium (K) was determined by using the dry ashing method. For this purpose, 1 g of ground plant material was taken in 50 ml porcelain crucible and was placed in muffle furnace and temperature was increased to 550°C, and crucibles were taken out and then cool ash was dissolved in 5 ml of 2N HCl. After mixing for 20 min, the volume was brought up to 50 ml, and was filtered by using Whatman No. 42 filter paper. The K concentration was determined through colorimetric method (Chapman & Pratt Citation1961).
The Ca+2, Mg+2, Mn+2, Zn+2, Cu+2, and Fe+3 were determined with dry ashing. For this purpose, 1 g of ground plant material was taken in 50 ml porcelain crucibles and placed in cool muffle furnace and increased the temperature gradually to 550°C, for 5 hours after attaining 550°C temperature. After 5 h muffle furnace was shut off and left for cooling, and crucibles were taken out carefully. The cooled ash was dissolved in 5 ml of 2N HCl and mixed with plastic rod. After 20 min, the volume was brought up to 50 ml with distal water and mixed thoroughly and allowed to stand for 30 min. The Ca+2, Mg+2, Mn+2, Zn+2, Cu+2, and Fe+3 were determined by atomic absorption spectroscopy after filtration through Whatman No. 42 filter paper (Chapman & Pratt Citation1961).
Plant enzymes activity
Samples for enzymes activity analyses were prepared by homogenizing 0.5 g of frozen leaf material in 3 ml of cold solution containing 50 × 10−3 M Na phosphate buffer (pH 7.8), 1 × 10−3 Ethylenediaminetetraacetic acid (M EDTA) and 2% (w/v) Polyvinylpolypyrrolidone (PVPP). The homogenate was centrifuged at 0°C for 40 min at 13,000 g.
Determination of glutathione S-transferase activity (GST)
The GST was determined by the method of Habig et al. (Citation1974). The samples were homogenized in phosphate buffer at pH 6.5 and 100 mM and then centrifuged at 9,000 g for 30 min. The change in optical density was measured at 340 nm wave length. Concentrations of GST were expressed in units/mg protein.
Determination of POX
The method of Nakano and Azada (Citation1987) was used for the spectrophotometric determination of ascorbate POX activity. The final solution (3 ml) was made by the addition of 100 μl of enzyme extract, 50 μl of 0.3% H2O2, and 2850 μl phosphate buffer NaK-Ascorbate (50 mM NaK, 0.5 mM ascorbate, and pH 7.2). The activity of ascorbate POX was measured at 290 nm wave length and activity was expressed as units/mg protein.
Determination of catalase activity (Cata)
The spectrophotometric determination of Cata was made by following the method of Cakmak and Horst (Citation1991). The final solution was made by adding 100 μl of the crude enzyme extract, 50 μl of hydrogen peroxide to 0.3% H2O2, and 2850 μl of phosphate buffer (50 mM, pH7.2). The absorbance was recorded at a wavelength of 240 nm. The Cata was expressed as units/mg protein.
Experimental design
Two PGPR isolates (TACC1 and TACC2) containing ACC-deaminase activity, 2 PGPR isolates (Azotobacter and TRN1) possessing N-fixing activity, and 2 PGPR isolates (TAN1 and TAN2) having both ACC-deaminase and N fixing activity were selected for incubation experiment. The inoculum for trial was prepared by growing the selected PGPR isolates. For inoculation of treatments, germinated seeds were dipped in broth containing selected ACC-deaminase and/or N-fixing isolates. For control (CK) treatments, the tomato seeds were dipped in sterilized flasks containing 0.03 M MgSO4 and then dipped into the medium. Four inoculated seeds were sown in each pot having 500 g soil/pot. Sterilized ½ strength Hoagland solution was used to provide nutrients to growing seedling. Although N free Hoagland solution was used to provide nutrients to growing seedlings inoculated with rhizobacteria containing N-fixing activity (i.e. Azotobacter and TRN1), there were four replications for each treatment. Pots were arranged randomly according to completely randomized design (CRD) in a growth room under axenic conditions. After 30 days of germination, tomato plants were harvested, and the parameters regarding root length, root fresh and dry weight, shoot length, shoot fresh and dry weight, number of leaves, chlorophyll a, b, and carotenoid contents, fresh and dry weight of seedlings (root + shoot), macro- and micronutrients in plant shoot and root and antioxidant enzymes, i.e. GST, POX and Cata, were recorded.
Statistical analysis
The data were statistically analyzed by Statistix 8.1 statistical package (Statistix, USA). Parametric statistics of analysis of variance (ANOVA) was carried out to estimate the effect of rhizobacterial isolate inoculation on root length, root fresh and dry weight, shoot length, shoot fresh and dry weight, number of leaves, chlorophyll and carotenoid contents, fresh and dry weight of seedlings (root + shoot), macro and micronutrients in plant shoot and root, and plant enzymes activity. Mean separations were achieved using a least significant difference (LSD) test at p < 0.05.
Results
Root length
The inoculation of tomato seedlings with rhizobacterial isolates containing N-fixing and/or ACC-deaminase activity increased the root length as compared to uninoculated control that ranged from 2.21-fold to 8.26-fold under normal growth conditions (). The isolate TAN1 showed maximum increase in the root length that was 8.26-fold as compared to uninoculated control. Conversely, TAN2 was the next effective isolate in promoting root length (6.79-fold) as compared to uninoculated control. The other isolates, namely, Azotobacter, TRN1, TACC1, and TACC2 caused 5.76-, 4.77-, 3.28-, and 2.21-fold increase in root length as compared to uninoculated control.
Root fresh weight
Results showed that inoculation of tomato seedlings with rhizobacterial isolates containing N-fixing and/or ACC-deaminase activity increased the root fresh weight over uninoculated control that ranged from 2.09-fold to 8.36-fold (). The isolate TAN1 presented highest increase in root fresh weight that was 8.36-fold higher as compared to uninoculated control. The TAN2 was the next effective isolate that resulted in 6.81-fold higher root fresh weight as compared to uninoculated control. The increase in the root fresh weight due to other isolates, namely, Azotobacter, TRN1, TACC1, and TACC2 was 5.54-, 4.63-, 3.18-, and 2.09–fold, respectively, as compared to uninoculated control.
Root dry weight
The results of root dry weight showed that inoculation of tomato seedlings with rhizobacterial isolates containing N-fixing and/or ACC-deaminase activity increased the root dry weight over uninoculated control that ranged from 3.1-fold to 12.6-fold (). The maximum increase in the root dry weight was observed in the treatment inoculated with TAN1, i.e. 12.6-fold. The inoculation of other rhizobacterial strains, namely, TAN2, Azotobacter, TRN1, TACC1, and TACC2 also significantly (P < 0.05) increased the root dry weight ranged from 8.6-, 6.1-, 4.6-, 3.7-, and 3.1-fold, respectively, as compared to uninoculated control.
Shoot length
The shoot length of tomato seedlings increased in response to inoculation with rhizobacterial isolates containing either N-fixing and/or ACC-deaminase activity that ranged from 2.20 to 6.92 fold as compared to uninoculated control (). The most effective isolate was TAN1 that caused 6.92-fold increase in shoot length of tomato seedlings over uninoculated control. The TAN2 was the next effective isolate that increased the shoot length up to 5.93-fold as compared to uninoculated control. Tomato seedlings also responded better when inoculated with Azotobacter, TRN1, TACC1, and TACC2 and showed increase in the shoot length i.e. 4.95-, 4.26-, 3.04-, and 2.21-fold as compared to uninoculated control.
Shoot fresh weight
Inoculation with rhizobacteria containing either ACC-deaminase and/or N-fixing activity increased the shoot fresh weight that ranged from 2.0- to 9.33-fold as compared to uninoculated control. The most effective isolate was TAN1 that caused 9.33-fold increase over uninoculated control (). The next effective isolates were TAN2, Azotobacter, and TRN1 that caused 7.66-, 4.33-, and 3.66-fold increases, respectively, whereas the isolate TACC1 and TACC2 caused least increase of 2.66- and 2.0-fold, respectively.
Shoot dry weight
Results revealed that inoculation of tomato seedlings with rhizobacterial isolates containing N-fixing and/or ACC-deaminase activity significantly (P < 0.05) increased the shoot dry weight as compared to uninoculated control that ranged from 2-fold to 6.91-fold under normal growth conditions (). The isolate TAN1 showed highest increase in shoot dry weight that was 6.91-fold higher than uninoculated control. The TAN2 was the next effective isolate that resulted in 5.71-fold higher shoot dry weight as compared to uninoculated control. The increase in the shoot dry weight due to other isolates, namely, Azotobacter, TRN1, TACC1, and TACC2 was 4.63-, 3.9-, 2.91-, and 2-fold, respectively, as compared to uninoculated control.
Number of leaves
All rhizobacterial isolates caused increase in the number of leaves that ranged from 3.1- to 11.0-fold as compared to uninoculated control (). Among all the isolates, TAN1 and TAN2 showed most promising results and caused 11.0- and 9.0-fold increase in the number of leaves as compared to uninoculated control. Azotobacter was the next effective isolate that caused 7.31-fold increase in the number of leaves as compared to uninoculated control. Conversely, TRN1 and TACC1 showed 6.19- and 4.38-fold increase in the number of leaves over uninoculated control, respectively. While isolate TACC2 showed minimum increase in the number of leaves as compared to uninoculated control, i.e. 3.01-fold.
Chlorophyll a contents
The inoculation with either ACC-deaminase and/or N-fixing activity containing rhizobacteria significantly (P < 0.05) increased the chlorophyll a contents that ranged from 1.82- to 6.25-fold (). Isolate TAN1 was found to be the most effective that caused 6.25-fold increase in chlorophyll a contents over uninoculated control. The next effective isolates were TAN2, Azotobacter, TRN1, TACC1, and TACC2 that resulted in about 4.81-, 3.78-, 3.11-, 2.21-, and 1.83-fold increase in chlorophyll a contents, respectively, compared to uninoculated control.
Chlorophyll b contents
Results showed that inoculation with rhizobacteria containing either ACC-deaminase and/or N-fixing activity increased the chlorophyll b contents that ranged from 2.23- to 10.7-fold as compared to uninoculated control (). Among all the isolates, TAN1 and TAN2 caused highest increase in the chlorophyll b contents, i.e. 10.7- and 8.2-fold compared to uninoculated control. Azotobacter was the next effective isolate that showed 6.28-fold increase in the chlorophyll b contents compared to uninoculated control. Similarly, TRN1 and TACC1 showed 5.0- and 3.11-fold increase in the chlorophyll b contents over uninoculated control, respectively, whereas isolate TACC2 showed minimum increase in the chlorophyll b contents as compared to uninoculated control that was 2.2-fold.
Carotenoid contents
The inoculation with either ACC-deaminase and/or N-fixing activity containing rhizobacteria significantly (P < 0.05) increased the carotenoid contents that ranged from 1.85- to 8.81-fold (). Isolate TAN1 was found to be the most effective that caused 8.81-fold increase in carotenoid contents over uninoculated control. The TAN2, Azotobacter, and TRN1 were the next most effective isolates that resulted in 7.25-, 5.85-, and 3.91-fold increase in carotenoid contents, respectively, compared to uninoculated control. The TACC1 and TACC2 isolates showed minimum increase in the carotenoid contents as compared to uninoculated control that was 2.86- and 1.85-fold.
Fresh weight of seedlings (root + shoot)
The effect of inoculation with either ACC-deaminase and/or N-fixing activity containing rhizobacteria on the fresh weight of seedling is shown in . Data showed that fresh weight of seedlings increased significantly (P < 0.05) as a result of inoculation, and ranged from 2.02- to 9.07-fold. All rhizobacterial isolates caused significant (P < 0.05) increase in the fresh weight of seedlings, and among isolates, the TAN1 was the most efficient isolate, and caused an increase of 9.07-fold. The TAN2 was the second-best isolate, and caused an increase of 7.43-fold. The next effective isolates were Azotobacter, TRN1, TACC1, and TACC2 that resulted in about 4.65-, 3.92-, 2.80-, and 2.02-fold increase, respectively.
CK, control; AB, Azotobacter
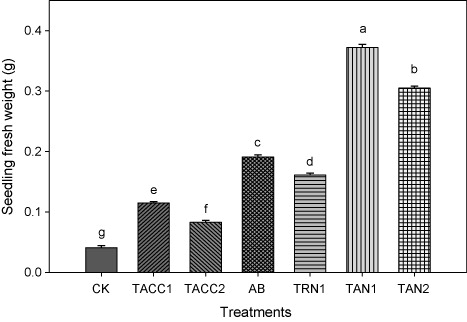
Dry weight of seedling (roots + shoots)
The inoculation with either ACC-deaminase and/or N-fixing activity containing rhizobacteria significantly (P < 0.05) increased the dry weight of seedlings that ranged from 1.93- to 8.71-fold (). Isolate TAN1 was the most effective that caused 8.71-fold increase in dry weight of seedlings over uninoculated control. The next effective isolates were TAN2, Azotobacter, TRN1, TACC1, and TACC2 that resulted in 7.14-, 4.5-, 3.78-, 2.71-, and 1.92-fold increase in dry weight of seedlings, respectively, compared to uninoculated control.
CK, control; AB, Azotobacter
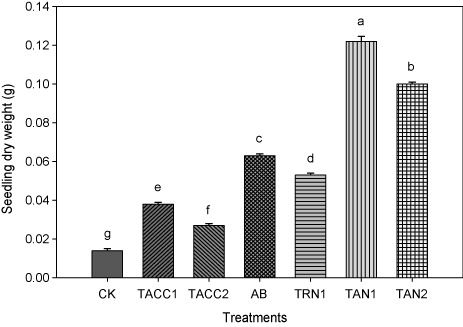
Macronutrient contents in shoot
Data showed that inoculation with rhizobacteria containing either ACC-deaminase or N-fixing activity increased the plant ionic uptake, e.g. macronutrient contents (N, P, K, Ca, and Mg) in shoot (). Among all the isolates, the maximum N (7.7-fold), P (10.5-fold), K (7.8-fold), Ca (12.7-fold), and Mg (12.6-fold) contents in plant shoot were found in TAN1. The other isolates, namely, TAN2, Azotobacter, TRN1, TACC1, and TACC2 also significantly (P < 0.05) increased the N (6.3-, 5.9-, 2.5-, 4.1-, and 1.8-fold), P (7-, 2.8-, 5.3-, 4-, and 1.5-fold), K (6.4-, 4.9-, 2-, 3.6-, and 1.5-fold), Ca (6.2-, 7.7-, 4.7-, 2.8-, and 1.4-fold), and Mg (4.6-, 6.7-, 9.2-, 2.8-, and 1.8-fold) in the plant shoot compared to control.
CK, control; AB, Azotobacter
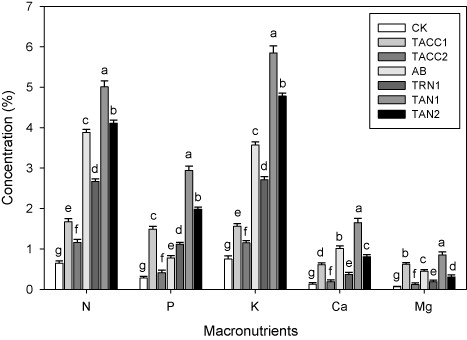
Macronutrient contents in root
Results showed that inoculation with rhizobacteria containing either ACC-deaminase and/or N-fixing activity increased the plant ionic uptake, e.g. macronutrient contents (N, P, K, Ca, and Mg) in root ). The highest N (8.9-), P (11.4-), K (8.8-) Ca (8.2-), and Mg (9-fold) contents in the plant root were observed in treatments inoculated with TAN1. The other isolates, namely, TAN2, Azotobacter, TRN1, TACC1, and TACC2 also significantly (P < 0.05) increased the N (7.6-, 5.4-, 2.9-, 4.1-, and 2-fold), P (8.7-, 7.3-, 2.8-, 4.6-, and 1.7-fold), K (7-, 4.9-, 2.6-, 3.2-, and 2-fold), Ca (4.1-, 6.3-, 3.5-, 2.1-, and 1.4-fold), and Mg (3.1-, 4.6-, 6.8-, 1.9-, and 1.5-fold) in the plant root compared to control.
CK, control; AB, Azotobacter
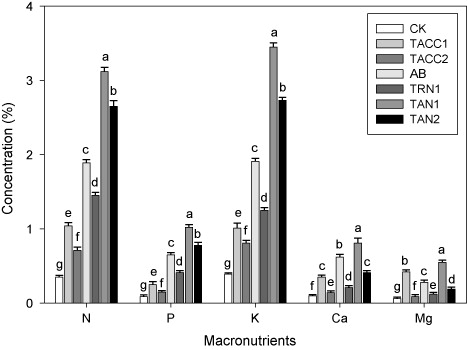
Micronutrient contents in shoot
Data showed that inoculation with rhizobacteria containing either ACC-deaminase or N-fixing activity increased the plant ionic uptake, e.g. micronutrient contents (Zn, Cu, Fe, and Mn) in shoot (). Among all the isolates, the maximum Zn (6.6-), Cu (9.3-), Fe (7.7-), and Mn (4.7-fold) contents in plant shoot were found in treatments inoculated with TAN1. The other isolates, namely, TAN2, Azotobacter, TRN1, TACC1, and TACC2 also significantly (P < 0.05) increased the Zn (5.8-, 4.7-, 2.8-, 3.8-, and 2-fold), Cu (7.6-, 5.5-, 2.9-, 4.1-, and 1.9-fold), Fe (4-, 5-, 6.4-, 3.1-, and 2.2-fold), and Mn (3.5-, 4-, 2.9-, 2.4-, and 1.9-fold) contents in the plant shoot compared to control.
CK, control; AB, Azotobacter
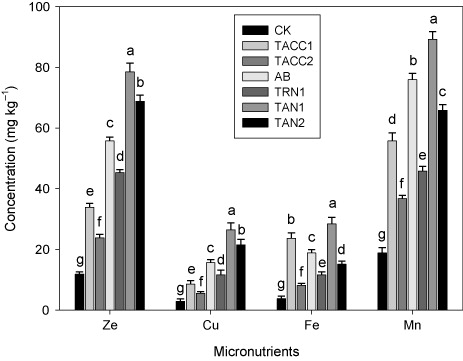
Micronutrient contents in root
Data showed that inoculation with rhizobacteria containing either ACC-deaminase or N-fixing activity increased the plant ionic uptake, e.g. micronutrient contents (Zn, Cu, Fe, and Mn) in root (). Among all the rhizobacterial isolates, the maximum Zn (10.2-), Cu (10.3-), Fe (10.7-), and Mn (5.7-fold) contents in plant root were found in TAN1. The other isolates, namely, TAN2, Azotobacter, TRN1, TACC1, and TACC2 also significantly (P < 0.05) increased the Zn (8.7-, 6.8-, 3.6-, 5.2-, and 2.1-fold), Cu (7.5-, 5.7-, 2.5-, 3.9-, and 1.5-fold), Fe (5.2-, 6.9-, 8.6-, 3.4-, and 1.9-fold), and Mn (4-, 4.8-, 3.2-, 2.8-, and 2.1) contents in the plant root compared to control.
CK, control; AB, Azotobacter
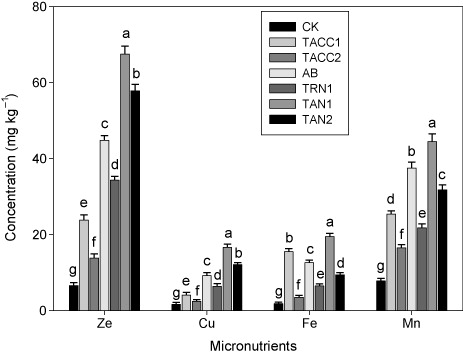
Glutathione S-transferase activity
The inoculation with rhizobacteria containing either ACC-deaminase or N-fixing activity significantly (P < 0.05) increased the GST activity (). All rhizobacterial isolates showed increase in the GST activity. Nevertheless, among all rhizobacterial isolates, TAN1 was the most effective and showed highest increase in the GST activity, i.e. 11.7-fold. The TAN2, Azotobacter, and TRN1 were the next best effective rhizobacterial isolates and caused an increase of 9-, 7.1-, and 5.3-fold in the GST activity, whereas, TACC1 and TACC2 showed minimum increase in the GST activity comparing with other rhizobacterial isolates, i.e. 3.5- and 1.9-fold.
Peroxidase activity
Data of plant enzymes activity showed that inoculation with rhizobacteria containing either ACC-deaminase or N-fixing activity significantly increased the POX activity (). Comparing the efficacy of rhizobacterial isolates, the TAN1 was the best isolate and showed an increase of 8.1-fold in the POX activity. The other rhizobacterial isolates, namely, TAN2 (6.4-fold), Azotobacter (4.7-fold), TRN1 (3.6-fold), TACC1 (2.8-fold), and TACC2 (1.9-fold) also showed a significant increase in the POX activity compared to control.
Catalase activity
The inoculation with either ACC-deaminase or N-fixing activity containing rhizobacteria significantly (P < 0.05) increased the CAT activity that ranged from 2.2 to 10.5-fold (). Isolate TAN1 was the most effective that caused 10.5-fold increase in CAT activity over uninoculated control. The next effective isolates were TAN2, Azotobacter, TRN1, TACC1, and TACC2 that resulted in, 8.3-, 6.8-, 5.4-, 3.9-, and 2.2-fold increase in CAT activity, respectively, compared to uninoculated control.
Characteristics of isolates
Some pertinent characteristics of isolates used in the experiment are given in . It was observed from characterization of isolates that, among all isolates, TAN1 had maximum root colonization activity, i.e. 11.7×107 and produced more auxin (30.1 mg L−1) in the presence of L-TRP. Conversely, TACC1 had maximum ACC-deaminase activity, i.e. 18.58 μmol α-ketobutyrate g-biomass 1/2 h–1.
Discussion
Inoculation with rhizobacterial isolate containing ACC-deaminase and N-fixing activity significantly (P < 0.05) increased the root length as compared to uninoculated control. Comparing the effect of rhizobacterial isolates, the TAN1 showed maximum root length than other isolates and uninoculated control (). Ali et al. (Citation2011) found that inoculation of PGPR significantly increased the root and shoot length and dry biomass of wheat over uninoculated plants. Significant increases in growth and in yield of agronomically important crops in response to inoculation with PGPR have been found in an experiment at Recife, Brazil (Figueiredo et al. Citation2008). It was revealed that isolate having both N-fixing and ACC-deaminase activity (such as TAN1) showed more promising increase in root length, due to its maximum root colonization activity, and minimizing the endogenous levels of ethylene synthesis (Shaharoona et al. Citation2003).
Data regarding root fresh and dry weight revealed that all rhizobacterial isolates had significant effect, and among isolates TAN1 showed significant (P < 0.05) increase in root fresh and dry weight as compared to uninoculated control ( and ). Radhakrishnan et al. (Citation2014) investigated the plant growth promotion and stress mitigation effects of PGPR, i.e. RDA01, NICS01, and DFC01 on sesame plants. Authors observed significant increase in the shoot length, root length, and fresh and dry seedling weight. Fröhlich et al. (Citation2012) found that inoculation with PGPR, i.e. Pseudomonas sp. DSMZ 13134, significantly increased the shoot fresh and dry weight of barley.
Results of shoot length showed that the most effective isolate was TAN1 that caused highest increase in shoot length over uninoculated control (). Yet again, isolates that had both ACC-deaminase and N-fixing activities proved to be the best than isolates that had only one activity. Zhou et al. (Citation2014) found significant increase in the growth and development of five flower species, i.e. shoot and petals length, and concluded that certain PGPR have the ability to enhance the host plants’ growth. Radhakrishnan et al. (Citation2014) investigated the effect of PGPR on the shoot length of sesame plants and found significant increase in the shoot length of sesame seedlings over uninoculated control.
Data of shoot fresh and dry weight of tomato seedling showed that the most effective isolate was TAN1 that caused highest increase in shoot fresh and dry weight over uninoculated control ( and ). The effect of other rhizobacterial isolates were in the order of TAN2 > Azotobacter > TRN1 > TACC1 > TACC2. This suggested that PGPR isolate that had both ACC-deaminase and N-fixing activities can perform much better than do isolates that had single ability. Shaharoona et al. (Citation2006) in a pot experiment revealed that inoculation with PGPR containing ACC-deaminase activity significantly increased the root and shoot growth and fresh and dry weights. It has been reported that certain microorganisms contain an enzyme ACC-deaminase that hydrolyzed the ACC into NH3 and α-ketobutyrate and reduced the inhibitory effects of ethylene and increased the root-shoot length and fresh and dry weights (Mayak et al. Citation2004).
The results of number of leaves revealed that TAN1 and TAN2 showed significant positive effect, as compared to other rhizobacterial isolates and uninoculated control (). The effect of other rhizobacterial isolates on the number of plant leaves was in the order of Azotobacter > TRN1 > TACC1 > TACC2. Secretion of plant growth-promoting substances by the bacteria could be responsible for the beneficial effects of PGPR on plant growth parameters, e.g. number of leaves, root, and shoot length and weight (Vafadar et al. Citation2014). The ACC-deaminase enriched rhizobacterial isolates (like TAN1) are capable of promoting the plant growth by increasing the root and shoot growth and number of leaves by lowering the endogenous ethylene production (Ali et al. Citation2011).
In case of chlorophyll a, b, and carotenoid contents, data clearly revealed that among all the experimental isolates, again TAN1 showed significant (P < 0.05) increase in chlorophyll a, b, and carotenoid contents compared to other rhizobacterial isolates and uninoculated control (–). The effect of other isolates was in the order of TAN2 > Azotobacter > TRN1 > TACC1 > TACC2. Vafadar et al. (Citation2014) revealed that rhizobacterial inoculation increased the chlorophyll and carotenoid contents. Stefan et al. (Citation2013) investigated the effects of inoculation of two PGPR (S4 and S7) on photosynthesis and yield of runner bean and found that the tested strains, alone or in combination, increased photosynthesis and leaves chlorophyll and carotenoid contents.
Data of fresh and dry weights of seedlings (root + shoot), showed that all rhizobacterial isolates caused significant (P < 0.05) increase in the fresh and dry weights of seedlings, and among rhizobacterial isolates, the TAN1 was the most efficient isolate, followed by TAN2, Azotobacter, TRN1, TACC1, and TACC2 ( and ). Vafadar et al. (Citation2014) concluded that inoculation with Azospirillum species enhanced the production of auxin and is thus related to the rapid establishment of a bigger root system that stimulates the growth and vigor and eventually seedling weight (root + shoot) of the host plant. The PGPR can supplement the nutrient requirement of tomato on soilless culture media under protected environment and increased the overall growth and weight of the tomato seedlings (Jan et al. Citation2011).
The significant increase (P < 0.05) in the ionic uptake of plant, e.g. macronutrient contents (N, P, K, Ca, and Mg) in the shoot and root was observed after inoculation with rhizobacterial isolates ( and . Among the rhizobacterial isolates, TAN1 was the most effective isolate and caused highest increase in the macronutrient contents of the plant shoot and root. The efficacy of other rhizobacterial isolates was in the following order TAN2 > Azotobacter > TRN1 > TACC1 > TACC2. Vafadar et al. (Citation2014) concluded that in comparison to control, inoculation with PGPR significantly increased the mineral N, P, and K content in plants shoot and root. Campanelli et al. (Citation2013) examined that inoculation with PGPR significantly increased the macronutrients contents, i.e. N of the globe artichoke. Sahran and Nehra (Citation2011) concluded that inoculation with PGPR significantly increased the plant mineral (N, P, K, Ca, and Mg) consumption.
The inoculation of rhizobacterial isolates on the ionic uptake, i.e. micronutrient (Zn, Cu, Fe, and Mn) contents of plant shoot and root showed a significant increase. All rhizobacterial isolates increased the ionic consumption in the shoot and root of plant in the following order TAN1 > TAN2 > Azotobacter > TRN1> TACC1 > TACC2 and ). Sahran and Nehra (Citation2011) stated that PGPR increased the nutrient availability for plants by converting the fixed and unavailable nutrients, e.g. Zn, Cu, and Fe into mobile, soluble, and available. conducted a study at Erzurum, Turkey, and found that PGPR are important for plant nutrition by increasing the availability of nutrients (Zn, Cu, and Mn) in soil and uptake in plants.
The data related to plant enzymes activity showed (–) that inoculation of rhizobacterial isolates significantly enhanced the antioxidant plant enzymes activity, namely, GST, ascorbate POX, and Cata. All rhizobacterial isolates had positive effect on plant enzymes activity, however, comparing the efficacy of the rhizobacterial isolates, the TAN1 was found to be the best. The effect of other rhizobacterial isolates on the plant enzymes activity was in the order of TAN2 > Azotobacter > TRN1 > TACC1 > TACC2. Damodaran et al. (Citation2014) found that treated gladiolus plants showed an increased activity of antioxidant enzymes, i.e. catalase, POX, superoxide dismutase, and phenyl alanine lyase. The PGPR produce a wide variety of antibiotics and enzymes, which enhance the plant vigor to withstand the deleterious biotic and abiotic stresses (Sahran & Nehra Citation2011). Hayat et al. (Citation2010) stated that growth-promoting rhizobacteria increase the plant vigor and decrease the biotic and abiotic stresses by producing different hormones and enzymes.
Results of rhizobacterial isolates characteristics clearly revealed that among all isolates, TAN1 had maximum root colonization activity and produced more auxin in the presence of L-TRP (). Whereas, TACC1 had maximum ACC-deaminase activity among all rhizobacterial isolates (). The difference in plant growth promotion by different rhizobacterial isolates may be due to the differences in their efficiency of colonizing the germinating roots, production of plant hormones (e.g. auxin) and ability to hydrolyze the ACC in plant roots (Zahir et al. Citation2004). Asghar et al. (Citation2004) reported a 3.5-fold increase in auxin production by rhizobacteria with L-TRP than without L-TRP. It has been reported that other traits, e.g. production of IAA, auxin, phosphate solubilization, and chitin production by PGPR, are helpful for plants in better nutrient mobilization, availability, and thus uptake and overall growth of the host plant (Zafar-ul-Hye et al. Citation2007). Phosphate solubilization, dinitrogen fixation, ACC-deaminase and antifungal activity, IAA, and siderophore biosynthesis characteristics of PGPR are responsible for the better plant growth promotion (Sahran & Nehra Citation2011). The results of this study concluded that PGPR safeguard the plants from the deleterious effects of biotic and abiotic stresses by producing phytohormones and antioxidant enzymes and increase the plant growth by increasing the nutrients availability and uptake. The ACC enrichment technique is an effective and efficient approach to select most promising PGPR. Rhizobacteria containing both ACC-deaminase and N-fixing activity are more effective than rhizobacteria containing either ACC-deaminase or N-fixing activity alone for growth promotion of agricultural crops. It is important to increase the working and productive efficiency of a specific PGPR with the proper optimization and acclimatization according to the prevailing soil and environmental conditions. Further in-depth research is essential to understand the functioning mechanisms of PGPR and to find out more competent rhizobacterial strains which can work under diverse agro-ecological conditions.
References
- Ahemad M. 2012. Implications of bacterial resistance against heavy metals in bioremediation: a review. Inst Integ Omics Appl Biotech. 3:39–46.
- Ahemad M, Malik A. 2011. Bioaccumulation of heavy metals by zinc resistant bacteria isolated from agricultural soils irrigated with wastewater. Bacteriol J. 2:12–21. 10.3923/bj.2012.12.21
- Ali SZ, Sandhya V, Grover M, Linga VR, Bandi V. 2011. Effect of inoculation with a thermotolerant plant growth promoting Pseudomonas putida strain AKMP7 on growth of wheat (Triticum spp.) under heat stress. J Plant Interact. 6:239–246. 10.1080/17429145.2010.545147
- Arnon DI. 1949. Copper induced enzyme in isolated chloroplasts. Polyphenoloxidase in Beta vulgaris. Plant Physiol. 24:1–15. 10.1104/pp.24.1.1
- Arshad M, Saleem M, Hussain S. 2007. Perspectives of bacterial ACC deaminase in phytoremediation. Trends Biotechnol. 25:356–362. 10.1016/j.tibtech.2007.05.005
- Asghar HN, Zahir ZA, Arshad M. 2004. Screening rhizobacteria for improving growth, yield and oil contents of canola (Brassica napus L.). Aust J Agri Res. 55:187–194. 10.1071/AR03112
- Buresh RJ, Austin ER, Craswell ET. 1982. Analytical methods in N-15 research. Fert Res. 3:37–62. 10.1007/BF01063408
- Çakmakçi R, Dönmez F, Aydm A, Şahin F. 2006. Growth promotion of plants by plant growth-promoting rhizobacteria under greenhouse and two different field soil conditions. Soil Biol Biochem. 38:1482–1487.
- Cakmak I, Horst WJ. 1991. Effect of aluminium on lipid peroxidation, superoxidase dismutase, catalase and peroxidase activities in root tips of soybean (Glycine max). Physiol Plant. 83:463–468. 10.1111/j.1399-3054.1991.tb00121.x
- Campanelli A, Ruta C, Tagarelli A, Morone-Fortunato I, Mastro GD. 2013. Effectiveness of mycorrhizal fungi on globe artichoke (Cynara cardunculus L. var. scolymus) micropropagation. J Plant Interact. 9:100–106. 10.1080/17429145.2013.770928
- Chapman HD, Pratt PF. 1961. Methods of analysis for soils, plants and water. Berkeley: University of California.
- Damodaran T, Rai RB, Jha SK, Kannan R, Pandey BK, Vijayalaxmi Sah, Mishra VK, Sharma DK. 2014. Rhizosphere and endophytic bacteria for induction of salt tolerance in gladiolus grown in sodic soils. J Plant Interact. 9:577–584. 10.1080/17429145.2013.873958
- Davies KG, Whitbread R. 1989. A comparison of method for measuring the colonization of root system by fluorescent pseudomonads. Plant Soil. 116:239–246. 10.1007/BF02214553
- Dworken M, Foster J. 1958. Experiment with some microorganisms which utilize ethane and hydrogen. J Bacteriol. 75:92–601.
- Figueiredo MVB, Burity HA, Martinez CR, Chanway CP. 2008. Alleviation of water stress effects in common bean (Phaseolus vulgaris L.) by co-inoculation Paenibacillus and Rhizobium tropici. Applied Soil Ecol. 40:182–188. 10.1016/j.apsoil.2008.04.005
- Fröhlich A, Buddrus-Schiemann K, Durner J, Hartmann A, von Rad U. 2012. Response of barley to root colonization by Pseudomonas sp. DSMZ 13134 under laboratory, greenhouse, and field conditions. J Plant Interact. 7:1–9.
- Gamalero E, Berta G, Glick BR. 2009. The use of microorganisms to facilitate the growth of plants in saline soils. In: Khan, MS, Zaidi A, Musarrat J, editors. Microbial strategies for crop improvement. New York: Springer; p. 1–22.
- Glick BR. 2012. Plant growth-promoting bacteria: mechanisms and applications, Hindawi Publishing Corporation, Scientifica. 2012, Article ID 963401; p. 15. doi:10.6064/2012/963401
- Habig WH, Pabst MJ, Jakoby WB. 1974. Glutathione S-transferases, the first enzymatic step in mercapturic acid formation. J Biol Chem. 249:7130–7139.
- Hassan W. 2013. C and N mineralization and dissolved organic matter potentials of two contrasting plant residues: effects of residue type, moisture and temperature. Acta Agr Scand B-S P. 63:642–652.
- Hassan W, Akmal M, Muhammad I, Younas M, Zahaid KR, Ali F. 2013. Response of soil microbial biomass and enzymes activity to cadmium (Cd) toxicity under different soil textures and incubation times. Aust J Crop Sci. 7:674–680.
- Hassan W, Bano R, Bashir F, David J. 2014. Comparative effectiveness of ACC-deaminase and/or nitrogen fixing rhizobacteria in promotion of maize (Zea mays L.) growth under lead pollution. Environ Sci Pollut Res. doi:10.1002/clen.201300727
- Hassan W, Bano R, Khatak BU, Hussain I, Yousaf M, David J. 2014. Temperature sensitivity and soil organic carbon pools decomposition under different moisture regimes: effect on total microbial and enzymatic activity. CLEAN – Soil, Air, Water. 21:10983–10996. 10.1002/clen.201300727
- Hassan W, Chen W, Cai P, Huang Q. 2013. Oxidative enzymes, the ultimate regulator: implications for factors affecting their efficiency. J Environ Qual. 42:1779–1790. 10.2134/jeq2013.05.0204
- Hassan W, Chen W, Cai P, Huang Q. 2014. Estimation of enzymatic, microbial and chemical properties in Brown soil by microcalorimetry. J Therm Anal Cal. 116:969–998.
- Hassan W, Chen W, Huang Q, Mohamed I. 2013. Microcalorimetric evaluation of soil microbiological properties under plant residues and dogmatic water gradients in Red soil. Soil Sci Plant Nut. 59:858–870. 10.1080/00380768.2013.845735
- Hassan W, David J. 2014. Effect of lead pollution on soil microbiological index under spinach (Spinacia oleracea L.) cultivation. J Soils Sediments. 14:44–59. 10.1007/s11368-013-0802-3]SS
- Hassan W, David J, Abbas F. 2014. Effect of type and quality of two contrasting plant residues on CO2 emission potential of Ultisol soil: implications for indirect influence of temperature and moisture. Catena. 114:90–96. 10.1016/j.catena.2013.11.001
- Hayat R, Ali S, Amara U, Khalid R, Ahmed I. 2010. Soil beneficial bacteria and their role in plant growth promotion: a review. Ann Microbiol. 60:579–598. 10.1007/s13213-010-0117-1
- Honma M, Shimomura T. 1978. Metabolism of 1-aminocyclopropane-1-carboxylic acid. Agri Biol Chem. 42:1825–1831. 10.1271/bbb1961.42.1825
- Jan AT, Azam M, Ali A, Haq QMR. 2011. Novel approaches of beneficial Pseudomonas in mitigation of plant diseases–an appraisal. J Plant Interact. 6:195–205. 10.1080/17429145.2010.541944
- Khalid A, Akhtar MJ, Mahmood MH, Arshad M. 2006. Effect of substrate-dependent microbial ethylene production on plant growth. Microbiology. 75:231–236. 10.1134/S0026261706020196
- Lal L. 2002. Phosphate mineralizing and solubilizing microorganisms. Phosphatic biofertilizers. Udaipur: Agrotech Publishing Academy; p. 224.
- Mayak S, Tirosh T, Glick BR. 2004. Plant growth-promoting bacteria confer resistance in tomato plants to salt stress. Plant Physiol Biochem. 42:565–572. 10.1016/j.plaphy.2004.05.009
- Nakano Y, Azada K. 1987. Purification of ascorbate peroxidase in spinach chloroplasts: its inactivation in ascorbate depleted medium and reactivation by monodehydroascorbate radical. Plant Cell Physiol. 28:131–140.
- Naveed M, Zahir ZA, Khalid M, Asghar HN, Akhtar MJ, Arshad M. 2008. Rhizobacteria containing ACC-deaminase for improving growth and yield of wheat under fertilized conditions. Pak J Bot. 40:1231–1241.
- Premono HME, Moawad AM, Vlek PLG. 1996. Effect of phosphate-solubilizing Pseudomonas putida on the growth of maize and its survival in the rhizosphere. Indonesian J Crop Sci. 11:13–23.
- Radhakrishnan R, Kang SM, Baek IY, Lee IJ. 2014. Characterization of plant growth-promoting traits of Penicillium species against the effects of high soil salinity and root disease. J Plant Interact. 9:754–762. 10.1080/17429145.2014.930524
- Raymond J, Siefert JL, Staples CR, Blankenship RE. 2004. The natural history of nitrogen fixation. Mol Biol Evol. 21:541–554. 10.1093/molbev/msh047
- Regan DL. 1988. Other micro-algae. In: Borowitzka MA, Borowitzka LJ, editors. Microalgal biotechnology. Cambridge: Cambridge University Press; p. 135–150.
- Sahran BS, Nehra V. 2011. Plant growth promoting rhizobacteria: a critical review. Lif Sci Med Res. 2011:LSMR-21.
- Saleem M, Arshad M, Hussain S, Bhatti AS. 2007. Perspective of plant growth promoting rhizobacteria (PGPR) containing ACC deaminase in stress agriculture. J Indian Microbiol Biotechnol. 34:635–648. 10.1007/s10295-007-0240-6
- Sarwar M, Arshad M, Martins DA, Frankenberger Jr. 1992. Tryptophan-dependent biosynthesis of auxins in soil. Plant Soil. 147:207–215. 10.1007/BF00029072
- Savci S. 2012. An agricultural pollutant: chemical fertilizer. Int J Environ Sci Dev. 3:77–80.
- Shaharoona B, Arshad M, Zahir ZA. 2006. Effect of plant growth promoting rhizobacteria containing ACC-deaminase on maize (Zea mays L.) growth under axenic conditions and on nodulation in mung bean. Lett Appl Microbiol. 42:155–159. 10.1111/j.1472-765X.2005.01827.x
- Shaharoona B, Arshad M, Zahir ZA, Mahmood MH. 2003. 1-aminocyclopropane-1-carboxylate (ACC) enrichment: an effective approach to screen plant growth promoting rhizobacteria for maize. Pak J Agri Sci. 40:126–132.
- Spaepen S, Vanderleyden J. 2011. Auxin and plant-microbe interactions. Cold Spring Harb Perspect Biol. 3(4): pii: a001438. Available from: http://dx.doi.org/10.1101/cshperspect.a001438.
- Stefan M, Munteanu N, Stoleru V, Mihasan M. 2013. Effects of inoculation with plant growth promoting rhizobacteria on photosynthesis, antioxidant status and yield of runner bean. Rom Biotech Letters. 18(2):8132–8143.
- Vafadar F, Amooaghaie R, Otroshy M. 2014. Effects of plant growth-promoting rhizobacteria and Arbuscular mycorrhizal fungus on plant growth, stevioside, NPK and chlorophyll content of Stevia rebaudiana. J Plant Interact. 1:128–136. 10.1080/17429145.2013.779035
- Van-Schouwenberg JCH, Walinge I. 1973. Methods of analysis for plant material. Wageningen (The Netherlands): Agriculture University Wageningen.
- Wollum II AG. 1982. Cultural methods for soil microorganisms. In: Page AL, editor. Methods of soil analysis, part 2: chemical and microbiological properties, Agronomy No. 9. Madison, WI: ASA; p. 781–802.
- Zafar-ul-Hye M, Zahir ZA, Shahzad SM, Naveed M, Arshad M, Khalid M. 2007. Preliminary screening of rhizobacteria containing ACC-deaminase for promoting growth of lentil seedlings under axenic conditions. Pak J Bot. 39:1725–1738.
- Zahir ZA, Arshad M, Frankenberger WT. 2004. Plant growth promoting rhizobacteria: applications and perspectives in agriculture. Adv Agron. 81:97–168. 10.1016/S0065-2113(03)81003-9
- Zahir ZA, Munir A, Asghar HN, Shaharoona B, Arshad M. 2008. Effectiveness of rhizobacteria containing ACC-deaminase for growth promotion of pea (Pisum sativum) under drought conditions. J Microbiol Biotechnol. 18:958–963.
- Zaidi A, Khan MS, Ahemad M, Oves M. 2009. Plant growth promotion by phosphate solubilizing bacteria. Acta Microbiol Immunol Hung. 56:263–284. 10.1556/AMicr.56.2009.3.6
- Zhou Z, Zhang C, Zhou W, Li W, Chu L, Yan J, Li H. 2014. Diversity and plant growth-promoting ability of endophytic fungi from the five flower plant species collected from Yunnan, Southwest China. J Plant Interact. 9:585–591. 10.1080/17429145.2013.873959