Abstract
The objective of this study was to investigate the effect of different concentrations of methyl salicylate (MeSA) on direct defense and indirect defense in poplar cuttings (Populus × euramericana ‘Nanlin 895’). Four defense-related enzyme activities, such as superoxide dismutase (SOD, EC1.15.1.1), oxydoreductases peroxidase (POD, EC1.11.1.7), catalase (CAT, EC1.11.1.6), and polyphenol oxidase (PPO, EC 1.14.18.1), were measured. The results showed that the SOD activities were induced by 1.0 and 10.0 mM MeSA and the POD activities were induced by MeSA. The CAT activity was induced at low concentrations of MeSA but was inhibited by high concentrations, and the PPO activity was affected by 0.1, 1.0, and 10.0 mM MeSA. Furthermore, the volatiles were detected using gas chromatography–mass spectrometry and gas chromatography. Twenty-one volatile compounds were tentatively identified in the emissions from plant leaves. Of these volatile compounds, (Z)-3-hexen-1-ol and cis-3-hexenyl acetate emissions were the highest. (Z)-3-Nonen-1-ol, (E)-2-hexen-1-ol, 1-octanol, (E,Z)-3,6-nonadien-1-ol, β-ionone, and hexadecanamide were six compounds that were only produced after treatment by 10.0 mM MeSA. These results showed that direct and indirect defense mechanisms in poplars were induced by MeSA.
Abbreviations | ||
MeSA | = | methyl salicylate |
SOD | = | superoxide dismutase |
POD | = | oxydoreductases peroxidase |
CAT | = | catalase |
PPO | = | polyphenol oxidase |
PVPP | = | polyvinylpolypyrrolidone |
GC–MS | = | gas chromatography–mass spectrometry |
SA | = | salicylic acid |
HIPV | = | herbivore-induced plant volatile |
Introduction
Plants have evolved protective mechanisms to keep deleterious reactions to a minimum, such as antioxidative enzymatic defenses, including oxydoreductases peroxidase (POD), polyphenol oxidase (PPO), and catalase (CAT), or nonenzymatic defenses (Qin et al. Citation2005; Mahdavian et al. Citation2007). Methyl salicylate (MeSA) is a volatile organic compound that is widespread in the plant world. It is synthesized from salicylic acid (SA), a phytohormone that contributes to plant systemic resistance defenses. MeSA is synthesized by members of a family of O-methyltransferases (Tieman et al. Citation2010). MeSA and SA are key compounds in the shikimic acid pathway and are involved in the induction of direct and indirect plant defenses (Dicke & Hilker Citation2003). MeSA is an important component of the signal transduction cascades, which activate the plant defense response, and it induces an oxidative burst in sunflower seedling roots (Garrido et al. Citation2009).
MeSA is naturally produced by plants in response to herbivore damage (Van Den Boom et al. Citation2004). It is a herbivore-induced plant volatile (HIPV; Gadino et al. Citation2012) and has been shown to attract natural enemies and affect herbivore behavior (Mallinger et al. Citation2011). HIPVs attract the arthropod natural enemies of herbivores and therefore may potentially be able to enhance biological control. However, HIPVs can also affect the behavior of arthropod herbivores and possibly higher-order natural enemies, which may complicate the use of HIPVs in biological control (Orre et al. Citation2010). The positive response of beneficial arthropods toward MeSA and other herbivore-induced volatiles has been demonstrated in a number of laboratory assays (De Boer & Dicke Citation2004; Ishiwari et al. Citation2007; Shimoda Citation2010). For example, Coccinella septempunctata may use MeSA as the olfactory cue for prey location (Zhu & Park Citation2005).
In this study, we investigated both direct and indirect plant defenses in poplar cuttings (Populus × euramericana ‘Nanlin 895’). We focused on the change in enzymes related to direct defense and in the volatile compounds related to indirect defense. The aim of this study was to determine if there were any differences in poplar chemical defenses when treated with different concentrations of MeSA, compared to the control plants, in order to see whether these treatments had an effect on the antioxidant defense system and indirect volatiles defense systems, and thereby ascertain the changes in the direct and indirect defense mechanisms of poplar when treated with exogenous MeSA.
Materials and methods
Plant materials and growth conditions
Poplar cuttings (P. × euramericana ‘Nanlin 895’) were provided from the forest cultivation seedling base at Nanjing Forestry University. The seedlings were grown in a growth chamber at 25 ± 1°C and with a L16:D8 photoperiod. They were watered daily and supplied with Hoagland nutrient solution (Afrousheh et al. Citation2010) every 2 weeks in order to avoid water and nutrient stress. They were used in experiments after they had grown to a height of 60–80 cm.
Plant treatment
Similar plants were chosen for the MeSA experiment in order to minimize the effects of differences between plants. The leaves were sprayed with 0.001, 0.01, 0.1, 1.0, and 10 mM MeSA (purity >99.5%; Sigma-Aldrich, St Louis, MO, USA) solution (with 50% alcohol as a solvent) at about 25 μl solution cm−2. Both sides of the leaves were sprayed. They were then used to assay for enzyme activities. For the volatile compounds experiment, the leaves were sprayed with 10 mM MeSA solution then all the volatile compounds were determined. The control plants were sprayed with 50% alcohol solution only. The leaves were harvested with their petiole after 24, 48, and 72 h. All the experiments were repeated three times and three replications were run at each time point in each treatment. New plants were sampled at each time point.
Assay for enzyme activities
Superoxide dismutase (SOD) activity was determined according to Oncel et al. (Citation2004) with slight modification. Exactly 200 mg of fresh leaf with 20 mg polyvinylpolypyrrolidone (PVPP) was ground and extracted in 3 ml 0.05 M phosphate buffer (pH 7.8). The homogenate was centrifuged at 1737 g for 10 min at 4°C. The reaction mixture (3 ml) contained 1.5 ml potassium phosphate buffer (pH 7.8), 50 µl enzyme extract, and 250 µl distilled water, 300 µl 130 mM L-methionine, 300 µl 750 µM nitroblue tetrazolium chloride (NBT), 300 µl 20 µM riboflavin, and 300 µl 100 µM ethylenediamine tetra acetic acid disodium salt. The reaction mixture was kept under 4000-l × fluorescent light for 30 min at 25°C. NBT reduction ratios were measured with a spectrophotometer adjusted to 560 nm (Cary 50). One SOD unit was described as the amount of enzyme where the NBT reduction ratio was 50%.
POD activity was assayed according to Yingsanga et al. (Citation2008) with slight modification. Exactly 100 mg of fresh leaf was homogenized in 2 ml 0.1 M ice-cold sodium phosphate buffer (pH 7.0). Then the homogenate was centrifuged at 1646 g for 10 min at 4°C and the supernatant was stored at −20°C until it was needed for the POD spectrophotometric assays. The substrate used in the POD activities analysis consisted of 50 µM guaiacol (Sigma) with 0.04% H2O2 (wt/wt) added as a cofactor. An aliquot of 500 µl of leaf enzyme extract was added to 1.5 ml of substrate in phosphoric acid buffer (0.1 M; pH 6.0) and the change in absorbance of the mixture at 470 nm was measured for 1 min (Cary 50). One unit of enzyme activity was defined as a change of 0.01 in absorbance per min.
CAT activity was assayed according to Tejera García et al. (Citation2007) with slight modification. Exactly 100 mg of fresh leaf was homogenized in 2 ml 0.2 M ice-cold sodium phosphate buffer (pH 7.8) containing 10 mg PVPP (Sigma). Then the homogenate was left for 10 min at 4°C and the supernatant was centrifuged at 1737 g for 15 min at 4°C. The supernatant was stored at −20°C until it was needed for the CAT spectrophotometric assays. An aliquot of 50 µl of leaf enzyme extract was added to 2.95 ml of 3.39 mM H2O2 in phosphoric acid buffer (0.05 M; pH 7.8) and the change in absorbance of the mixture at 240 nm was measured for 1 min (Cary 50). One unit of CAT activity was defined as the amount of protein decomposing 1 µmol of H2O2 per min, when compared to a control reaction without substrate.
To assay for PPO activity, 100 mg of fresh leaf was homogenized in 1.5 ml 0.1 M ice-cold sodium phosphate buffer (pH 7.5) that contained 10 mg PVPP (Sigma). Then the homogenate was centrifuged at 27,664 g for 20 min at 4°C and the supernatant was stored at −20°C until it was needed for the PPO spectrophotometric assays. PPO activity was assayed according to Vanitha and Umesha (Citation2011) with slight modification. An aliquot of 50 µl of leaf enzyme extract was added to 2.95 ml of 13.56 mM catechol (Sigma) in phosphoric acid buffer (0.2 M; pH 4.0) and the change in absorbance of the mixture at 420 nm was measured for 1 min (Cary 50). One unit of enzyme activity was defined as a change of 0.01 in absorbance per min.
Collection of volatile compounds
Headspace solid-phase micro extraction (SPME) was used to analyze the volatiles coming from the treated leaves (Zhang & Pawliszyn Citation1993). The fiber coating was PDMS-100 (100 µm polydimethylsiloxane; Supelco, Bellefonte, PA, USA). The leaf sample (2 g, shredded) was placed in a 15 ml glass vial that had been closed with a septum cap. The septum was punctured and the SPME needle inserted into the headspace. Samples were extracted at 45°C and the fiber was exposed for 30 min. After this, the fiber was inserted into the injector of a gas chromatography (GC) or a gas chromatography–mass spectrometry (GC–MS) system for thermal desorption of the volatiles (Abel et al. Citation2009). All the experiments were repeated three times, and three replications were run at each time point for each treatment. New plants were used at each time point.
Chemical analyses
Gas chromatography–mass spectrometry (GC–MS)
GC–MS analyses were undertaken using an Agilent HP7890A GC coupled to an Agilent 5975C system (Agilent Technologies, USA) with the injector set at 250°C in the splitless mode. Analyses were performed using a DB-WAX column (30 m × 0.32 mm, 0.25 μm, Agilent J&W; 42 cm s−1 He was used as the carrier gas, temperature increased from 70°C [2 min] to 230°C at 6°C min−1 with a 6 min hold). The temperatures of the transfer line, the ion source, and the quadrupole were 250°C, 230°C, and 150°C, respectively. Electron ionization mass spectra were recorded (with a 4 min solvent delay) at 70 eV over the m/z range of 25–350 with a threshold abundance of 10.
The compounds were identified using the mass spectral libraries: NIST08.L (Agilent Technologies, Palo Alto, CA, USA) and Wiley275, and confirmed with authentic standards where available.
Gas chromatography (GC)
GC analyses were carried out using a HP6890 GC system (Agilent Technologies, USA). The injection port (250°C) was in the splitless mode and the flame ionization detector was heated to 250°C. A capillary column was used with N2 (37 cm s−1) as the carrier gas. A polyethylene glycol DB-WAX column (30 m× 0.32 mm, 0.25 μm, Agilent J&W) was maintained at 70°C for 2 min, then increased at 6°C min−1 to 230°C, which was maintained for 5 min (Tooker et al. Citation2008). The total ion peaks of each compound were integrated and the amount of each compound was calculated based on external calibration curves constructed from 1 µl injections of known n-heptanol, which was generated using authentic standards (Schmelz et al. Citation2003). All statistical analyses were performed using GraphPad InStat version 3.00.
Results
Induction of defense enzymes
The SOD, POD, CAT, and PPO activities induced by MeSA treatment over 72 h are shown in .
Note: All values are mean ± standard deviation of the mean (SD). Different letters indicate significant differences between the treatment and the control (P < 0.05; t test).
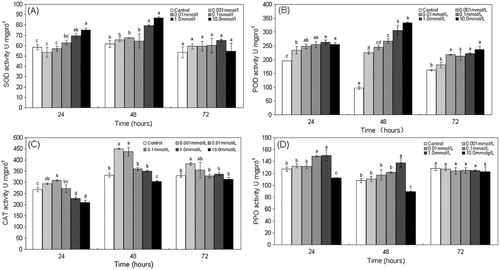
Treatment with the different concentrations of MeSA led to different effects on SOD activity in poplar leaves. At 24 and 48 h, the results for the 0.001, 0.01, and 0.1 mM concentrations were not significantly different (P > 0.05) from the controls, but the 1.0 and 10.0 mM treatments were significantly higher (P < 0.05) than the controls. After 72 h, none of the treatments were significantly different from the controls ().
In MeSA-treated plants, the POD specific activities increased (P < 0.05) for all treatments at 24 and 48 h compared to the control leaves. At 72 h, the 0.001 mM treated leaves were not significantly different (P > 0.05) from the controls, but the other treatments were all significantly higher than the controls. At 48 h, all treatments reached their peak rates (P < 0.001) for POD ().
At 24 h, the CAT activity in leaves treated with 0.001 and 0.01 mM of MeSA was higher (P < 0.05) than the controls. The 0.1 mM treatment did not differ (P > 0.05) from the control, but the CAT activity was lower (P < 0.05) in the 1.0 and 10.0 mM MeSA-treated leaves, compared to the control leaves. At 48 h, the CAT activity in the leaves treated with 0.001 and 0.01 mM MeSA was still higher (P < 0.05) than in the controls. The 0.1 and 1.0 mM treatments were not significantly different (P > 0.05) from the controls, but the 10.0 mM treatment was significantly lower than the controls. At 72 h, the CAT activity in the leaves treated with 0.001 mM was higher (P < 0.05) than the controls, but the other treatments were not significantly different ().
The 0.1 and 1.0 mM MeSA treatments increased the PPO activity in poplar leaves at 24 h, but 10.0 mM decreased activity. At 48 h, the PPO activity in poplar leaves treated with 1.0 mM of MeSA was higher (P < 0.05) than the controls but was lower in the leaves treated with 10.0 mM MeSA. At 72 h, the PPO activity in all treatments was not significantly different to the controls ().
Volatile compounds
In total, 21 volatile compounds were tentatively identified in the emissions from the treated and control plant leaves by comparing their retention indices and mass spectra with those of synthetic standards ( and ).
Notes: The bars represent hexyl formate (1), (Z)-butanoic acid-3-hexenyl ester (2), cis-3-hexenyl-2-methylbutyrate (3), 2-ethyl-1-hexanol (4), unknown (5), 2-hydroxy-benzaldehyde (6), 2,6-dimethyl-5-heptenal (7), methylsalicylate (8), methoxy-phenyl-oxime- (9), geraniol (10), phenol (11), eugenol (12), dibutylphthalate (13), (Z)-3-nonen-1-ol (14), (E)-2-hexen-1-ol (15), 1-octanol (16), (E,Z)-3,6-nonadien-1-ol (17), β-ionone (18), and hexadecanamide (19). Each compound was more than 80% matched. For each number, different small letters indicate statistically significant differences in volatile release concentrations between the treated and the control plants (GraphPad InStat version 3.00; N = 3; P < 0.05).
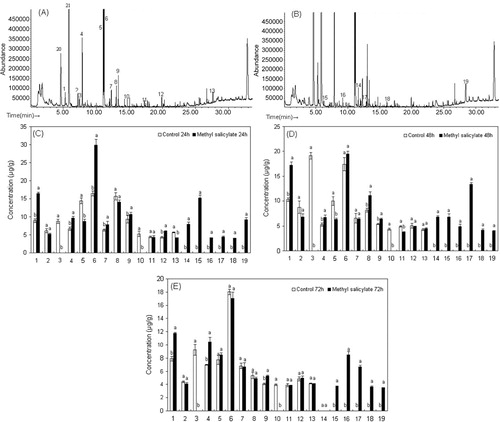
Most volatiles released from the poplar plants were common plant volatiles. However, further quantitative GC and GC–MS analyses revealed that there were differences in emissions between the treated and control leaves at the different time periods for the 21 compounds identified. The contents of the first 19 compounds were lower than the last two and they changed over time , , and ). The content of hexyl formate, 2-ethyl-1-hexanol, and methoxy-phenyl-oxime- increased significantly at 72 h after treatment with MeSA. There were no significant differences in the contents of (Z)-butanoic acid-3-hexenyl ester between the treated and control leaves over the three time periods. cis-3-Hexenyl-2-methylbutyrate and (E,Z)-3,6-nonadien-1-ol occurred only in the control plants and were not detected in the treated plants. The 2-hydroxy-benzaldehyde and 2,6-dimethyl-5-heptenal contents in the treated leaves were higher than in the control leaves at 24 h, but there were no significant differences at 48 and 72 h. The MeSA content was higher in the treated leaves than that in the control leaves at 48 h, but there were no significant differences at 24 and 72 h. The phenol contents of the treated leaves were not significantly different from the controls at 24 and 72 h but significantly lower than the controls at 48 h. The dibutylphthalate content was lower in the treated plants than that in the controls at 24 h, but there were no significant differences at 48 and 72 h. (Z)-3-Nonen-1-ol was only present in the treated plants and occurred at 24 and 48 h. (E)-2-Hexen-1-ol, 1-octanoloctanol, (E,Z)-3,6-nonadien-1-ol, β-ionone, and hexadecanamide were also only detected in the treated plants and occurred at 24, 48, and 72 h.
shows the content changes for the six compounds only found in the treated leaves at the different time periods. (Z)-3-Nonen-1-ol was detected at 24 and 48 h and its content at 24 h was significantly higher than at 48 h. The content of (E)-2-hexen-1-ol fell over time and each time period was significantly different. Its concentration reached a maximum (15.19 μg g−1) at 24 h. The content of 1-octanol increased over time and reached a maximum concentration (8.52 μg g−1) at 72 h. There was also a significant difference in concentration of (E,Z)-3,6-nonadien-1-ol between the 24 and 48 h time periods. At 24 and 48 h, the β-ionone concentrations were not significantly different, but they were significantly higher than at 72 h. The hexadecanamide concentration reached a maximum (9.16 μg g−1) at 24 h and then declined.
Table 1. The content changes of six volatile compounds only produced in the treated leaves (among 19 low-quantity volatile compounds) at the different time periods.
shows the concentrations of cis-3-hexenyl acetate and (Z)-3-hexen-1-ol in the poplar leaves at the 24, 48, and 72 h time periods. The results indicated that the amount of cis-3-hexenyl acetate released from the treated leaves was higher (P < 0.05) than that from the control leaves for the entire length of the experiment. The amount of (Z)-3-hexen-1-ol released from the treatment leaves was not significantly different from the control leaves (P > 0.05) at 24 h, but then increased and became higher than the controls at 48 and 72 h. The peak release quantities of these two compounds were 319.50 and 139.90 μg g−1, respectively, and occurred at 48 h.
Note: Columns with an asterisk or with different small letters have significantly different volatile release concentrations between the treated and control leaves (GraphPad InStat version 3.00; N = 3; P < 0.05).
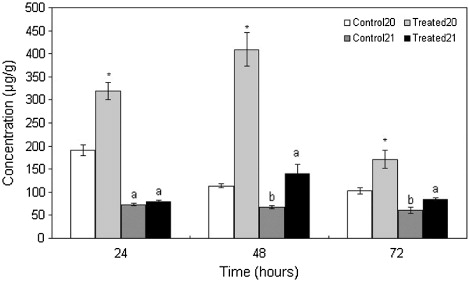
Discussion
Diverse environmental stresses affect plant processes in various different ways and can lead to the loss of cellular homeostasis and the formation of reactive oxygen species (ROS; Almeselmani et al. Citation2006), which induce the production of superoxide radicals (O2−), singlet oxygen (1O2), hydrogen peroxide (H2O2), and hydroxyl radicals (OH−; Foyer et al. Citation1994). They cause oxidative damage to membranes, lipids, proteins, and nucleic acids (Srivalli et al. Citation2003). Antioxidant enzymes play important roles in adaptation to stress conditions. SOD, POD, and CAT are protective enzymes in plants and they can be affected by light and cold stress (Gechev et al. Citation2003), salinity (Rios-Gonzalez et al. Citation2002; Misra & Gupta Citation2006), high temperature stress (Ali et al. Citation2005; Almeselmani et al. Citation2006), and drought stress (Sun et al. Citation2010). The coordinated functions of antioxidant enzymes, such as SOD, CAT, and POD, facilitate ROS processing and the regeneration of redox ascorbate and glutathione metabolites (Foyer & Nector Citation2000).
Within a cell, the SODs constitute the first line of defense against ROS (Alscher et al. Citation2002). SODs are enzymes that play a pivotal role in metabolizing O2−. They preempt oxidizing chain reactions that cause extensive damage and stop the formation of a number of deleterious ROS including: H2O2, hypochlorite (OCl−), peroxynitrate (ONO2−), and OH− (Miller Citation2012). SOD converts one form of ROS (O2−) to another equally toxic one (H2O2; Almeselmani et al. Citation2006). The increased SOD activity could be a consequence of excess O2− generation when leaves have been treated with high MeSA concentrations. MeSA induces antioxidant enzyme production, which might have resulted in the increased production of SOD seen at the 24 and 48 h time periods. The rise in SOD coincided with an activity increase in the H2O2 scavenging enzymes. SOD generates H2O2, which is then eliminated by POD (Rios-Gonzalez et al. Citation2002). PODs are polyfunctional enzymes that undergo two reaction cycles: the peroxidase–oxidase cycle (with NADH as substrate, also called NADH peroxidases) resulting in H2O2 production (Halliwell Citation1978) and the peroxidase cycle (with guaiacol as phenol substrate, also called guaiacol peroxidase) leading to H2O2 consumption (Fecht-Christoffers et al. Citation2003). PODs are believed to act as induced and constitutive defenses against leaf feeding insects (Barbehenn et al. Citation2010). The POD activity increased between 24 and 72 h in this study. CAT was the first antioxidant enzyme to be discovered and characterized. The typical CAT reaction is the dismutation of two molecules of H2O2 to water and O2 (Mhamdi et al. Citation2010). The CAT activity increased between 24 and 72 h when leaves were treated with low concentrations of MeSA, whereas high concentrations of MeSA caused CAT to decline over the same time frame. In these experiments, the H2O2 was probably of enzymatic origin, considering the oxygen concentration in the system and the positive effects of the various inhibitors of H2O2-producing enzymes (Blokhina et al. Citation2003). PPOs are metalloenzymes that catalyze the oxygen-dependent oxidation of phenols to quinones. Eolini et al. (Citation2004) suggested that the physiological regulation of PPO expressions was part of the plant defense system and was related to other metabolic pathways. Moreover, they also suggested that these enzymes were involved in plant responses to different stress conditions. Highly reactive intermediates and their secondary reactions are responsible for much of the oxidative browning that accompanies plant senescence, wounding, and responses to pathogens and herbivore attack. In addition, the role of PPO in the generation of ROS is evident from the large contribution PPO-based phenolic oxidation makes to H2O2 production in plant extracts (Thipyapong et al. Citation2004). Compared with the controls, PPO increased after leaves were treated with 0.1 and 1.0 mM MeSA at the 24 h time period but declined at the 24 and 48 h time periods after the plant leaves were treated with 10.0 mM MeSA.
Plant odors are the most ubiquitous volatiles in nature. Any type of surface damage commonly causes plant leaves to release so-called green leaf volatiles (Hoballah & Turlings Citation2005). Han and Chen (Citation2002) showed that tea can produce different compounds after being mechanically pierced or attacked by the tea aphid. This study investigated the effect of MeSA on the production of volatile blends in poplar. The amount of MeSA seen in poplar treated with 10 mM MeSA was significantly higher than in the control leaves () and MeSA has been shown to trigger the plant's own production of HIPVs (Khan et al. Citation2008). MeSA also acts as a transmission signal and recent evidence supports this conclusion (Schulaev et al. Citation1997; Chen et al. Citation2003; Koo et al. Citation2007; Park et al. Citation2007). It is also known to be a HIPV that attracts natural enemies of the herbivores feeding on the plants (Girling et al. Citation2006). After the leaves had been treated with MeSA, (Z)-3-hexen-1-ol, and (Z)-3-hexenyl acetate levels increased. This was consistent with others' results (Ruther et al. Citation2002; Fraser et al. Citation2003). Furthermore, Ruther's experiment showed that (Z)-3-hexen-1-ol was highly attractive to male Melolontha hippocastani antennae (Ruther et al. Citation2002). Fraser et al. (Citation2003) confirmed that male Manduca sexta can detect (Z)-3-hexenyl acetate and that MeSA elicited large electroantennogram responses, even when it was present in trace amounts. To the contrary, Snoeren et al. (Citation2010) demonstrated that MeSA acts as a repellent to naïve Diadegma semiclausum and that the repellent effect of MeSA was dose-dependent. Therefore, the effect of these plant volatile organic compounds on natural enemies of poplar pests needs further studies.
Additional information
Funding
References
- Abel C, Clauss M, Schaub A, Gershenzon J, Tholl D. 2009. Floral and insect-induced volatile formation in Arabidopsis lyrata ssp. petraea, a perennial, outcrossing relative of A. thaliana. Planta. 230:1–11. 10.1007/s00425-009-0921-7
- Afrousheh M, Ardalan M, Hokmabadi H, Afrousheh M. 2010. Nutrient deficiency disorders in Pistacia vera seedling rootstock in relation to eco-physiological, biochemical characteristics and uptake pattern of nutrients. Sci Hortic-Amesterdam. 124:141–148. 10.1016/j.scienta.2009.12.001
- Ali MB, Hahn EJ, Paek KY. 2005. Effects of temperature on oxidative stress defense systems, lipid peroxidation and lipoxygenase activity in Phalaenopsis. Plant Physiol Biochem. 43:213–223. 10.1016/j.plaphy.2005.01.007
- Almeselmani M, Deshmukh PS, Sairam RK, Kushwaha SR, Singh TP. 2006. Protective role of antioxidant enzymes under high temperature stress. Plant Sci. 171:382–388. 10.1016/j.plantsci.2006.04.009
- Alscher RG, Erturk N, Heath LS. 2002. Role of superoxide dismutases (SODs) in controlling oxidative stress in plants. J Exp Bot. 53:1331–1341. 10.1093/jexbot/53.372.1331
- Barbehenn R, Dukatz C, Holt C, Reese A, Martiskainen O, Salminen JP, Yip L, Tran L, Constabel CP. 2010. Feeding on poplar leaves by caterpillars potentiates foliar peroxidase action in their guts and increases plant resistance. Oecologia. 164:993–1004. 10.1007/s00442-010-1733-y
- Blokhina O, Virolainen E, Fagerstedt KV. 2003. Antioxidants, oxidative damage and oxygen deprivation stress: a review. Ann Bot. 91:179–194.
- Chen F, D'Auria JC, Tholl D, Ross JR, Gershenzon J, Noe JP, Pichersky E. 2003. An Arabidopsis thaliana gene for methylsalicylate biosynthesis, identified by a biochemical genomics approach, has a role in defense. Plant J. 36:577–588.
- De Boer JG, Dicke M. 2004. The role of methyl salicylate in prey searching behavior of the predatory mite Phytoseiulus persimilis. J Chem Ecol. 30:255–271.
- Dicke M, Hilker M. 2003. Induced plant defences: from molecular biology to evolutionary ecology. Basic Appl Ecol. 4:3–14. 10.1078/1439-1791-00129
- Eolini F, Hochkoeppler A, Credi A, Rodrìguez AG, Poggi V. 2004. Polyphenol oxidase expression in potato (Solanum tuberosum) tubers inhibited to sprouting by treatment with iodine atmosphere. Phytochemistry. 65:2181–2187. 10.1016/j.phytochem.2004.07.002
- Fecht-Christoffers MM, Braun H-P, Lemaitre-Guillier C, VanDorsselear A, Horst WJ. 2003. Effect of manganese toxicity on the proteome of the leaf apoplast in cowpea. Plant Physiol. 133:1935–1946.
- Foyer CH, Lelandais M, Kunert KJ. 1994. Photooxidative stress in plants. Physiol Plant. 92:696–717.
- Foyer CH, Nector G. 2000. Oxygen processing in photosynthesis: regulation and signaling. New Phytol. 146:359–388. 10.1046/j.1469-8137.2000.00667.x
- Fraser AM, Mechaber WL, Hildebrand JG. 2003. Electroantennographic and behavioral responses of the sphinx moth Manduca sexta to host plant headspace volatiles. J Chem Ecol. 29:1813–1833. 10.1023/A:1024898127549
- Gadino AN, Walton VM, Lee JC. 2012. Evaluation of methyl salicylate lures on populations of Typhlodromus pyri (Acari: Phytoseiidae) and other natural enemies in western Oregon vineyards. Biol Control. 63:48–55.
- Garrido I, Espinosa F, Álvarez-Tinaut MC. 2009. Oxidative defence reactions in sunflower roots induced by methyl-jasmonate and methyl-salicylate and their relation with calcium signalling. Protoplasma. 237:27–39.
- Gechev T, Willekens H, Van Montagu M, Inzé D, Van Camp W, Toneva V, Minkov I. 2003. Different responses of tobacco antioxidant enzymes to light and chilling stress. J Plant Physiol. 160:509–515.
- Girling RD, Hassall M, Turner JG, Poppy GM. 2006. Behavioural responses of the aphid parasitoid Diaeretiella rapae to volatiles from Arabidopsis thaliana induced by Myzus persicae. Entomol Exp Appl. 120:1–9.
- Halliwell B. 1978. Lignin synthesis: the generation of hydrogen peroxide and superoxide by horseradish peroxidase and its stimulation by manganese (II) and phenols. Planta. 140:81–88.
- Han BY, Chen ZM. 2002. Composition of the volatiles from intact and mechanically pierced tea aphid-tea shoot complexes and their attraction to natural enemies of the tea aphid. J Agric Food Chem. 50:2571–2575. 10.1021/jf010681x
- Hoballah ME, Turlings TC. 2005. The role of fresh versus old leaf damage in the attraction of parasitic wasps to herbivore-induced maize volatiles. J Chem Ecol. 31:2003–2018. 10.1007/s10886-005-6074-7
- Ishiwari H, Suzuki T, Maeda T. 2007. Essential compounds in herbivore-induced plant volatiles that attract the predatory mite Neoseiulus womersleyi. J Chem Ecol. 33:1670–1681. 10.1007/s10886-007-9344-8
- Khan ZR, James DG, Midega CAO, Pickett JA. 2008. Chemical ecology and conservation biological control. Biol Control. 45:210–224.
- Koo YJ, Kim MA, Kim EH, Song JT, Jung C, Moon JK, Kim JH, Seo HS, Song SI, Kim JK, et al. 2007. Overexpression of salicylic acid carboxyl methyltransferase reduces salicylic acid-mediated pathogen resistance in Arabidopsis thaliana. Plant Mol Biol. 64:1–15.
- Mahdavian K, Kalantari KM, Ghorbanli M. 2007. The effect of different concentrations of salicylic acid on protective enzyme activities of Pepper (Capsicum annuum L.) plants. Pak J Biol Sci. 10:3162–3165. 10.3923/pjbs.2007.3162.3165
- Mallinger RE, Hogg DB, Gratton C. 2011. Methyl salicylate attracts natural enemies and reduces populations of soybean aphids (Hemiptera: Aphididae) in soybean agroecosystems. J Econ Entomol. 104:115–124.
- Mhamdi A, Queval G, Chaouch S, Vanderauwera S, Van Breusegem F, Noctor G. 2010. Catalase function in plants: a focus on Arabidopsis mutants as stress-mimic models. J Exp Bot. 61:4197–4220.
- Miller AF. 2012. Superoxide dismutases: ancient enzymes and new insights. FEBS Lett. 586:585–595.
- Misra N, Gupta AK. 2006. Effect of salinity and different nitrogen sources on the activity of antioxidant enzymes and indole alkaloid content in Catharanthus roseus seedlings. J Plant Physiol. 163:11–18.
- Oncel I, Yurdakulol E, Keles Y, Kurt L, Yildiz A. 2004. Role of antioxidant defense system and biochemical adaptation on stress tolerance of high mountain and steppe plants. Acta Oecol. 26:211–218.
- Orre GUS, Wratten SD, Jonsson M, Hale RJ. 2010. Effects of an herbivore-induced plant volatile on arthropods from three trophic levels in brassicas. Biol Control. 53:62–67. 10.1016/j.biocontrol.2009.10.010
- Park SW, Kaimoyo E, Kumar D, Mosher S, Klessig DF. 2007. Methyl salicylate is a critical mobile signal for plant systemic acquired resistance. Science. 318:113–116.
- Qin QJ, Shi XY, Liang P, Gao XW. 2005. Induction of phenylalanine ammonia-lyase and lipoxygenase in cotton seedlings by mechanical wounding and aphid infestation. Prog Nat Sci. 15:419–423.
- Rios-Gonzalez K, Erdei L, Lips SH. 2002. The activity of antioxidant enzymes in maize and sunflower seedlings as affected by salinity and different nitrogen sources. Plant Sci. 162:923–930.
- Ruther J, Reinecke A, Hilker M. 2002. Plant volatiles in the sexual communication of Melolontha hippocastani: response towards time-dependent bouquets and novel function of (Z)-3-hexen-1-ol as a sexual kairomone. Ecol Entomol. 27:76–83.
- Schmelz EA, Alborn HT, Tumlinson JH. 2003. Synergistic interactions between volicitin, jasmonic acid and ethylene mediate insect-induced volatile emission in Zea mays. Physiol Plant. 117:403–412. 10.1034/j.1399-3054.2003.00054.x
- Schulaev V, Silverman P, Raskin I. 1997. Airborne signaling by methyl salicylate in plant pathogen resistance. Nature. 385:718–721.
- Shimoda T. 2010. A key volatile infochemical that elicits a strong olfactory response of the predatory mite Neoseiulus californicus, an important natural enemy of the two-spotted spider mite Tetranychus urticae. Exp App Acarol. 50:9–22.
- Snoeren TAL, Mumm R, Poelman EH, Yang Y, Pichersky E, Dicke M. 2010. The herbivore-induced plant volatile methyl salicylate negatively affects attraction of the parasitoid Diadegma semiclausum. J Chem Ecol. 36:479–489.
- Srivalli B, Vishanathan C, Renu KC. 2003. Antioxidant defense in response to abiotic stresses in plants. J Plant Biol. 30:121–139.
- Sun CH, Du W, Cheng XL, Xu XN, Zhang YH, Song D, Shi JJ. 2010. The effects of drought stress on the activity of acid phosphatase and its protective enzymes in pigweed leaves. Afr J Biotechnol. 9:825–833.
- Tejera García NA, Iribarne C, Palma F, Lluch C. 2007. Inhibition of the catalase activity from Phaseolus vulgaris and Medicago sativa by sodium chloride. Plant Physiol Biochem. 45:535–541.
- Thipyapong P, Melkonian J, Wolfe DW, Steffens JC. 2004. Suppression of polyphenol oxidases increases stress tolerance in tomato. Plant Sci. 167:693–703.
- Tieman D, Zeigler M, Schmelz E, Taylor MG, Rushing S, Jones JB, Klee HJ. 2010. Functional analysis of a tomato salicylic acid methyl transferase and its role in synthesis of the flavor volatile methyl salicylate. Plant J. 62:113–123.
- Tooker JF, Rohr JR, Abrahamson WG, De Moraes CM. 2008. Gall insects can avoid and alter indirect plant defenses. New Phytol. 178:657–671.
- Van Den Boom CE, Van Beek TA, Posthumus MA, De Groot A, Dicke M. 2004. Qualitative and quantitative variation among volatile profiles induced by Tetranychus urticae feeding on plants from various families. J Chem Ecol. 30:69–89.
- Vanitha SC, Umesha S. 2011. Pseudomonas fluorescens mediated systemic resistance in tomato is driven through an elevated synthesis of defense enzymes. Biol Plantarum. 55:317–322.
- Yingsanga P, Srilaong V, Kanlayanarat S, Noichinda S, McGlasson WB. 2008. Relationship between browning and related enzymes (PAL, PPO and POD) in rambutan fruit (Nephelium lappaceum Linn.) cvs. Rongrien and See-Chompoo. Postharvest Biol Tech. 50:164–168.
- Zhang ZY, Pawliszyn J. 1993. Headspace solid-phase microextraction. Anal Chem. 65:1843–1852.
- Zhu J, Park KC. 2005. Methyl salicylate, a soybean aphid-induced plant volatile attractive to the predator Coccinella septempunctata. J Chem Ecol. 31:1733–1746.