Abstract
Present experiments were conducted to assess the response of Panicum turgidum to salinity and possible role of arbuscular mycorrhizal fungi (AMF) in enhancing the salt tolerance. The activities of antioxidant enzymes such as superoxide dismutase (SOD), catalase (CAT), peroxidase (POD), glutathione reductase (GR) and compatible solutes were increased by salt stress and were further enhanced by AMF inoculation. Hydrogen peroxide and malonaldehyde content increased in salt-stressed plants while a reduction was observed due to AMF inoculation. Salt-stressed plants showed higher activities of pyruvate orthophosphate dikinase (PPDK), phosphoenolpyruvate carboxylase (PEPC) and malate dehydrogenase as compared to control and AMF-inoculated plants. Salt stress caused significant decrease in phosphorous, potassium and calcium uptake but an increase in sodium uptake was observed. AMF alleviate salinity-induced negative impact on the plant growth and nutrient uptake by reducing the oxidative damage through strengthening of the antioxidant system.
Introduction
Plants are continuously exposed to variety of environmental stresses resulting in perturbed growth and metabolism. Salinity is one of the important and adverse environmental constraint restricting growth and development of plant (Alqarawi et al. Citation2014; Fatma et al. Citation2014). At global level, salinity is an important problem particularly in arid and semiarid regions. Around 7% of the world's land is salt-affected (Ruiz-Lozano et al. Citation2001, Citation2012). Increasing industrialization and excessive use of saline water for crop irrigation further aggravates this problem. Salinity triggers osmotic effects resulting in reduced growth through alterations in physio-biochemical processes like photosynthesis, enzyme activity and ion homeostasis (Ahmad et al. Citation2012; Porcel et al. Citation2012; Fatma et al. Citation2014). Salt stress triggers excess production of toxic reactive oxygen species (ROS) and increased ROS levels induce oxidative stress in plants. Toxic ROS include superoxide, hydroxyl and peroxide radicals, and they pose detrimental effects to normal metabolism and growth (Mittler Citation2002; Ahmad et al. Citation2010). ROS produced accumulate in leaves and cause oxidation of various cellular molecules including lipids, proteins and chlorophylls. Peroxidation of membrane lipids, protein degradation and damage to nucleic acids are the immediate effects of the excess ROS (Mittler Citation2002; Ahmad et al. Citation2010). Moreover, photosynthetic apparatus and pigments are also often targeted by the toxic ROS (Ahmad et al. Citation2010; Fatma et al. Citation2014).
Nevertheless, plants are well equipped with different defense mechanisms to counteract the stress-induced oxidative damage. Upregulation of antioxidant enzymes is one of the prime defense mechanisms that help plants to combat stress-induced oxidative damage. Antioxidant defense system comprises superoxide dismutase (SOD), catalase (CAT), peroxidase (POD) and glutathione reductase (GR) (Ahmad et al. Citation2010; Liu et al. Citation2014). These intricate antioxidant enzymes mediate scavenging of toxic ROS so as to protect cells from the oxidative damage. In addition, increased synthesis and accumulation of compatible osmolytes is another tolerance mechanism employed by plants for ameliorating the damaging impacts of salinity (Ahmad & Sharma Citation2008; Ahanger et al. Citation2014b). Compatible solutes that contribute to osmoregulation through maintaining the cell water content include free proline, glycine betaine (GB), sugars and amino acids (Ahmad & Sharma Citation2008; Ahanger et al. Citation2014b). Accumulating compatible solutes or ions while bringing efficient sequestration and compartmentation of deleterious ions into the less sensitive parts like vacuole or apoplast is an important trait that determines salt tolerance in plants (Ahmad & Sharma Citation2008; Azooz et al. Citation2011). Most of the plants form symbiotic associations with the arbuscular mycorrhizal fungi (AMF). AMF improves host plant growth by enhancing the rhizospheric soil characteristics (Asghari et al. Citation2005; Ahanger et al. Citation2014a; Hodge & Storer Citation2015) and enhances mobilization and uptake of several essential elements by modifying root architecture (Wu et al. Citation2010; Ahanger et al. Citation2014a; Hameed et al. Citation2014; Hodge & Storer Citation2015). AMF has been reported to alleviate the salt stress in plants through various mechanisms. In citrus plants, AMF colonization caused enhancement in leaf area, photosynthesis and phosphorous uptake by plants (Sherstha et al. Citation1995).
Panicum turgidum Forssk belongs to Poaceae family is a C4 salt-tolerant perennial grass (Khan & Qaiser Citation2006; Koyro et al. Citation2013). It is commonly found in saline deserts of southern Pakistan but is also reported in other arid areas (Khan & Qaiser Citation2006). Halophytes are believed to be equipped well with several physiological and biochemical tolerance mechanisms and considered as suitable candidate plant for maintaining the sustainability of salt-affected soils (Khan et al. Citation2009). Present study was carried out to investigate the impact of salt stress on growth and key physio-biochemical attributes of P. turgidum and potential of AMF in ameliorating salt stress-induced deleterious changes.
Materials and methods
Soil, plant and mycorrhizal inoculums
The experiments were conducted under climate controlled growth chamber conditions at the Plant Production Department, College of Food & Agricultural Sciences, King Saud University, Riyadh, Saudi Arabia. The soil used in the present investigation was sandy loam with the following properties (%): moisture content, 18.17; organic carbon, 0.74; total soluble salts, 0.26; total nitrogen, 0.058; electrical conductivity (EC), 6.8 dS/m and pH 7.8. Dried soil and fine sand (2:1; w/w) was autoclaved for 3 h at 121°C and then divided equally among plastic pots (10 cm in diameter, with 5 kg capacity). The seeds of P. turgidum were collected from Thomama region (N 25° 336,291 E 46° 533,651), Riyadh, Saudi Arabia. Seeds were surface sterilized with sodium hypochlorite (0.5%, v/v) for 3 min followed by thorough washing with distilled water. Healthy germinating seeds were transferred to pots (1 plant/pot). Hoagland's solution (Hoagland & Arnon Citation1950) supplemented with NaCl (250 mM) as described by Koyro et al. (Citation2013) was used for irrigation every alternate day. The mycorrhizal inoculum [Funneliformis mosseae (syn. Glomus mosseae); Rhizophagus intraradices (syn. Glomus intraradices) and Claroideoglomus etunicatum (syn. Glomus etunicatum)] () was prepared according to Hashem et al. (Citation2014). Fungal inoculums consisted of AM fungal spores, hyphae and colonized root fragments. The mycorrhizal inoculum was added to the experimental soil as 10 g of trap soil culture (approx. 100 spores/g trap soil, M = 80%)/pot (1 Kg). Nonmycorrhizal soil served as control. The pots incubated for eight weeks in growth chamber at 20/30oC as night/day alternative temperatures (12/12 h).
Determination of arbuscular mycorrhizal colonization
The mycorrhizal spores were extracted from the experimental soil of each treatment by wet sieving and decanting method as described by Daniels and Skipper (Citation1982) and modified by Utobo et al. (Citation2011). The intensity of fungal infection (mycelium, vesicles and arbuscules) and development within the infected regions of the roots were calculated as described in details (Hashem et al. Citation2014) according to the following formula:
Photosynthetic pigments
The leaf samples (0.5 g) were extracted in aqueous acetone (80%) and extract was centrifuged at 10,000 × g for 10 min. The optical densities of the supernatant were recorded at 480, 645 and 663 nm (T80 UV/VIS Spectrometer, PG Instruments Ltd, USA) using acetone as blank (Arnon Citation1949).
Extraction and estimation of carbohydrate fractions
The extraction of soluble carbohydrate fractions was carried out according to the method of Said et al. (Citation1964). In this method, known weight of powdered leaf samples were homogenized in a mixture of 5.0 mL of 20% (w/v) phenol and 10.0 mL of 30% (w/v) trichloroacetic acid (TCA) and homogenate was left overnight in refrigerator at 4°C followed by centrifugation at 5000g for 15 min. The supernatant was used for estimation of water-soluble carbohydrates (monosaccharides and disaccharides), however the precipitate was oven dried (at 80°C for two successive constant weights) and used for estimation of polysaccharides. The content of soluble sugars (monosaccharides and disaccharides) and insoluble sugars (polysaccharides) was estimated according to the method of Nelson (Citation1944) as modified by Naguib (Citation1964).
Extraction and estimation of carboxylation enzymes
The fresh leaf samples were grinded in 50 mM Na acetate (pH 6.0) buffer as described by Omoto et al. (Citation2012). NADP-MDH (NADP-dependent malate dehydrogenase, EC 1.1.1.82) and NAD-MDH (NAD-dependent malate dehydrogenase, EC 1.1.1.37) activities were determined using the methods described by Scheibe and Stitt (Citation1988). NADP-ME (NADP-malic enzyme, EC 1.1.1.40) activity was assayed in accordance with Kanai and Edwards (Citation1973) with minor modifications as described in details by Omoto et al. (Citation2012). RuBPCase (ribulose-1,5-bisphosphate carboxylase, EC 4.1.1.39) activity was assayed according to Du et al. (Citation1996). The method described by Fukayama et al. (Citation2001) was used for assaying PPDK (Pyruvate orthophosphate dikinase, EC 2.7.9.1). PEPC (Phosphoenolpyruvate carboxylase, EC 4.1.1.31) activity was assayed following Du et al. (Citation1996).
Extraction and estimation of antioxidant enzymes
The fresh leaf tissues (5.0 g) were macerated in 10 mL potassium phosphate buffer [pH 7.8; 50 mM] using an ice cooled sterilized pestle and mortar. The extract was centrifuged at 15,000 × g for 15 min at 4°C and supernatant was used to determine the enzyme activity. Protein content in the extract was determined following Bradford (Citation1976).
SOD (EC 1.15.1.1) was assayed by measuring inhibition in photoreduction of nitroblue tetrazolium (NBT) according to Giannopolitis and Ries (Citation1977). The reaction mixture contained 500 μL phosphate buffer (pH 7.8), 0.5 mL distilled H2O, 100 μL methionine (13 mM), 50 μL NBT (63 μM) and 50 μL enzyme extract and was exposed to light for 20 min. The OD of the irradiated aliquot was read at 560 nm. Activity that caused 50% inhibition in photoreduction of NBT was considered as one enzyme unit. The CAT (EC 1.11.1.6) activity was determined following method of Chance and Maehly (Citation1955). The reaction mixture contain 1.9 mL potassium phosphate buffer (50 mM; pH 7.0) and 1 mL H2O2 (5.9 mM) and 100 μL of the enzyme extract. Decrease in OD was observed at 240 nm for 2 min. The method of Chance and Maehly (Citation1955) was used for determination of POD (EC 1.11.1.7) activity. One milliliter of reaction mixture contained 50 mM potassium phosphate buffer (pH 5.0), 100 μL guaiacol (20 mM), 100 μL H2O2 (40 mM) and 100 μL enzyme extract. The increase in OD at 470 nm was monitored for 2 min and activity was expressed as EU mg−1 protein. The activity of GR (EC 1.6.4.2) was monitored by observing decrease in absorbance at 340 nm for 2 min (Carlberg & Mannervik Citation1985). The activity of GR was calculated using the extinction coefficient of NADPH of 6.2 mM−1 cm−1 and expressed as EU mg−l protein.
Glycine betaine content
The dry leaf sample (0.5 g) was shaken in 10 mL toluene (0.5%) and kept at 4°C overnight. After centrifugation, 1 mL of the filtrate was mixed with 1 mL of sulfuric acid (2N). 0.5 mL of solution was taken and then 200 μL of potassium tri-iodide (KI3) solution (containing 7.5 g Iodine and 10 g Potassium iodide in 100 mL of 1 N HCl) was added to the tubes. The content was cooled in a chiller. Afterwards 2.8 mL of ice cooled deionized H2O and 5 mL of 1–2 di-chloroethane was added to the samples. The absorbance of the organic layer (lower layer) was recorded spectrophotometrically at 365 nm. The concentrations of GB were recorded against a standard curve developed with different concentrations of GB following Grieve and Grattan (Citation1983).
Hydrogen peroxide
The fresh leaf tissue (0.5 g) was ground in a prechilled mortar with 5 mL of 0.1% (w/v) TCA. This homogenate was then centrifuged at 12,000 × g for 15 min. To the supernatant (0.5 mL), 0.5 mL of potassium phosphate buffer (pH 7.0) and 1 mL of KI was added. The mixture was vortexed and the OD of suspension was read at 390 nm. H2O2 in the samples was determined following Velikova et al. (Citation2000).
Malondialdehyde
The fresh leaf tissue (500 mg) was extracted in 5 mL 1.0% TCA. The homogenate was centrifuged at 20,000 × g for 15 min. The supernatant (500 μL) was added with 2 mL 0.5% 2-thiobarbituric acid (prepared in 20% TCA). The samples were heated at 100°C for 1 h. Thereafter, the reaction was stopped by cooling the samples in ice, centrifuged at 10,000 × g for 10 min and the supernatant was read at 532 and 600 nm (Carmak & Horst Citation1991). The level of thiobarbituric acid reactive substances was calculated using the absorption coefficient of 155 mmol cm−1.
Total phenolics
Total phenolics in leaf tissues were estimated following method of Julkenen-Titto (Citation1985). Fresh leaf (0.5 g) was extracted with 80% acetone (5 mL) and the extract was centrifuged at 10,000 × g for 10 min. Aliquot (0.1 mL) was diluted to 2 mL using distilled H2O.Thereafter, 1 mL of Folin and Ciocalteau's phenol reagent and 5 mL of 20% sodium carbonate was added and was read at 750 nm.
Free proline
Leaf samples (500 mg) were homogenized in 10 mL of 3% sulfosalicylic acid following the method of Bates et al. (Citation1973). The crushed sample was filtered and 2.0 mL of filtrate were taken in a test tube, mixed with 2.0 mL acid ninhydrin solution and 2.0 mL glacial acetic acid. The reaction was started by incubating tubes at 100°C and terminated by keeping tubes on ice. After cooling, 4 mL toluene was added to the tubes and the upper phase containing toluene was read at 520 nm.
Estimation of ion accumulation
The estimation of Na+, K+, Mg2+ and Ca2+ concentrations was analyzed by flame photometrically described by Wolf (Citation1982). The phosphorus (P) was extracted by nitric-perchloric acid digestion and measured using the Vanado-molybdophosphoric colorimetric method (Jackson Citation1962). Standard curve of each mineral (10–100 μg/mL) was used as reference.
Statistical analysis
Duncan's Multiple Range Test was performed using One-Way ANOVA for a completely randomized design by SPSS-21 software and the differences in means (three replicates were used) were determined by the least significant differences (LSD) (p = 0.05) test.
Results
Mycorrhizal status
The typical morphological characters of an intact crushed spores of AMF used in the current study was shown in (a)–(i), C. etunicatum (a, b, c,) [syn. G. etunicatum]; F. mosseae (d, e, f) [syn. G. mosseae]; and R. intraradices (g, h, i) [syn. G. intraradices]. The result of the root colonization of P. turgidum with AMF is presented in (a)–(f).
Figure 2. The colonization of AMF in the roots of P. turgidum. (a): vesicle (V) and spore (S) occurrence of the AMF-colonized root section, (b): Vesicles (V) formed in roots of P. turgidum, (c): Hyphae (H) and hyphal coils (HC) in roots of P. turgidum, (d): colonization of plant roots by AMF hyphae (H), vesicles (V) and spore density (S), (e): The root segments colonized by arbuscles (A), trank (T) and hyphae (H) of AMF, (f): Arbuscles (A), and hyphae (H) colonize the root.
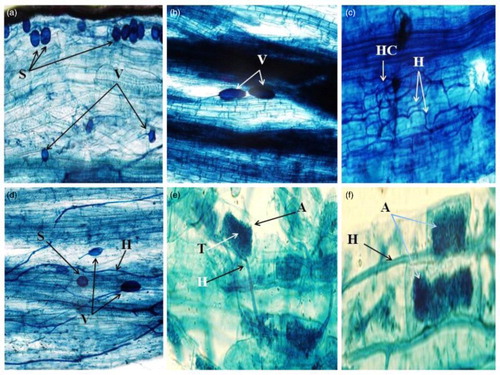
A different AMF infection patterns in the roots of P. turgidum was observed, including colonization of spores (S) and vesicles (V), arbuscles (A), trank (T) and hyphae (H). As shown in , salinity caused significant decrease in mycelia, number of spores, vesicles and arbuscules by 77.1%, 60.2%, 38% and 58.6%, respectively, as compared to the control plants.
Table 1. Effect of NaCl (250 mM) on total spores (spore/50 g soil) and structural colonization (%) of AMF in P. turgidum.
Photosynthetic pigments
Salinity reduced chlorophyll a, chlorophyll b and carotenoid contents by 26.1%, 48.5% and 51.2%, respectively (). However, AMF ameliorated the salinity-induced impact on these attributes (). The content of chlorophyll a, chlorophyll b and carotenoid in plants inoculated with AMF increased by 12.8%, 40.2%, and 21.9%, respectively, compared to the control plants.
Table 2. Effect of NaCl (250 mM) in presence and absence of AMF on photosynthetic pigment contents (mg/g fresh weight) in P. turgidum.
Antioxidative enzymes
Considerable increase in activities of SOD, POD, CAT and GR was observed in AMF-inoculated plants. Percent increase in SOD, POD, CAT and GR due to AMF was 41.6%, 21.25%, 9.9% and 7.3%, respectively ((a)–(d)). Salinity-induced increase in the level of SOD, POD, CAT and GR was 174.3%, 72.5%, 124.3% and 54.5%, respectively ((a)–(d)).
Activities of carbon metabolizing enzymes
The activity of PPDK, PEPC, NADP-malate dehydrogenase (NADP-MDH) and NAD-malate dehydrogenase (NAD-MDH) was increased by 51.4%, 93.3%, 130.7% and 68.3% in salt-stressed P. turgidum plants as compared to the control ((a)–(f)). However, AMF-inoculated plants maintained reduced activity of these enzymes. Percent decrease was 21.4%, 15.5%, 14.3% and 6.6% in PPDK, PEPC, NADP-MDH and NAD-MDH, respectively ((a)–(f)). An obvious reduction in activities of NADP-ME and Rubisco (up to 48.3% and 57%, respectively) was observed under salt stress conditions. However, AMF not only increased the activities of these enzymes but also ameliorated the salinity-induced changes to some extent ((a)–(f)). Percent increase in NADP-ME and Rubisco in AMF-inoculated plants was 22% and 10%, respectively.
Figure 4. (a)–(f) Effect of NaCl (250 mM) in presence and absence of AMF on activities of carbon metabolizing enzymes (μmol/mg chlorophyll/min) in P. turgidum. (a) pyruvate orthophosphate dikinase; (b) phosphoenolpyruvate carboxylase; (c) NADP-malate dehydrogenase; and (d) NAD-malate dehydrogenase; (e) NADP-malic enzyme and (f) Rubisco.
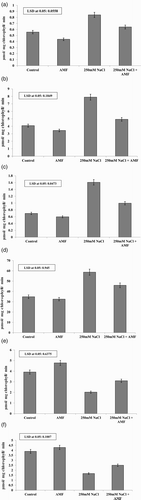
Hydrogen peroxide and malondialdehyde
In salt-stressed P. turgidum plants, H2O2 and malondialdehyde (MDA) content increased by 78.4% and 72.3%, while as in AMF-inoculated salt-stressed plants an increase observed by 5.2% and 24.1%, respectively (). In AMF-inoculated plants, H2O2 and MDA reduced by 2.9% and 35%, respectively, as compared to control plants ().
Table 3. Effect of NaCl (250 mM) in presence and absence of AMF on systemic resistance attributes [H2O2 (μMol/g fresh weight), MDA (nMol/g fresh weight), TP (mg/g fresh weight), GB (μMol/g fresh weight), and proline (μMol/g fresh weight) in P. turgidum.
Proline, glycine betaine and total phenols
P. turgidum plants subjected to salt stress showed increased accumulation of proline, GB and total phenols. An increase in proline, GB and total phenols due to salinity was 142.6%, 39.8% and 85.3%, respectively (). The plants inoculated with AMF showed a slight decrease in proline (25.1%), GB (6.9%) and total phenols (43.6%) content ().
Sugars
The salt-stressed P. turgidum plants showed reduced total sugar content than control plants. In salt-stressed plants, total sugars and polysaccharides decreased by 16% and 34.9%, respectively, while as in salt-stressed AMF (250mM + AMF) inoculated plants a reduction of 11.4% and 17.4% was observed (). However, AMF-inoculated plants showed 20.7% and 26.8% increase in total sugar and polysaccharide content (). Salt-stressed plants showed 84.3% and 114.1% enhancement in soluble sugar and monosaccharide contents, respectively (). However, in AMF-inoculated plants, soluble sugar and monosaccharide contents reduced by 6.03% and 26.4%, respectively.
Table 4. Effect of NaCl (250 mM) in presence and absence of AMF on sugars content (mg glucose/g dry weight) in P. turgidum.
Elements accumulation
AMF inoculation resulted in increased uptake of essential elements like phosphorous, potassium and calcium, while as reduced the sodium ion. Percent increase in phosphorous, potassium and calcium content due to AMF was 228.3%, 20.09% and 7%, respectively (). Salinity stress increased sodium by 55.8%, while reduced phosphorous, potassium and calcium by 52.4%, 40.4% and 18.4%, respectively. In salinity-stressed AMF plants, percent decrease in phosphorous, potassium and calcium was 63.6%, 29% and 3.2%, respectively ().
Table 5. Effect of NaCl (250 mM) in presence and absence of AMF on ion accumulation (mg/g dry weight) in P. turgidum.
Discussion
In our study, drastic decline in colonization and spore population in P. turgidum plants subjected to salt stress are in confirmation with the findings of Wu et al. (Citation2010) for citrus seedlings, Miranda et al. (Citation2011) for ground cherry and Aroca et al. (Citation2013) for lettuce. Salinity stress caused drastic decline in chlorophyll pigments. Our results of reduction in chlorophyll content due to salinity stress is in confirmation with the findings of Doganlar et al. (Citation2010), Rasool et al. (Citation2013) and Alqarawi et al. (Citation2014) for tomato, Cicer arietinum and Ephedra alata, respectively. In E. alata, Alqarawi et al. (Citation2014) demonstrated that chlorophyll contents reduced considerably with increasing salinity levels. Similarly, Azooz et al. (Citation2011) also observed drastic decline in chlorophyll contents due to saline irrigation. Reduced chlorophyll contents under stress is attributed to increased activity of chlorophyllase causing degradation of pigments and hence resulting in reduced photosynthesis and hence growth. High salinity restricts de novo synthesis of proteins as well as functioning of pigment protein complex (Levitt Citation1980; Sultana et al. Citation1999; El-Tayeb Citation2005). Compared with stressed plants, AMF-inoculated plants maintained higher contents of chlorophyll pigments. Our results of enhanced chlorophyll contents in AMF-colonized plants are in support of the findings of Hajiboland et al. (Citation2010) for Solanum lycopersicum L. and Aroca et al. (Citation2013) for lettuce. In pepper, Kaya et al. (Citation2009) demonstrated that inoculation of AMF increased chlorophyll content under normal as well as salt-stressed conditions. Enhancement in chlorophyll pigments due to AMF is because of enhanced mineral uptake especially magnesium, an important component of chlorophyll molecule (Sheng et al. Citation2008). Higher contents of chlorophyll pigments in AMF-inoculated plants contribute to greater photosynthetic activity leading to maintained growth. Hence, it is clear from our results that AMF inoculation enhances chlorophyll contents and also mitigates the negative impact of salinity to some extent.
Activities of different antioxidant enzymes are upregulated on exposure to environmental stress. Antioxidant enzymes mediate scavenging of toxic ROS and hence allaying the oxidative stress-induced deleterious effects on several sensitive molecules like proteins, nucleic acids and lipids. In our results, increase in activities of SOD, CAT, GR and POD as a result of salt stress is in concurrence with the findings of Koca et al. (Citation2007) for Sesamum indicum Mittal et al. (Citation2012) for Brassica juncea and Rasool et al. (Citation2013) for C. arietinum L. Enhancement in antioxidant enzyme activity under stress conditions helps in quick scavenging of ROS and maintaining their levels below the deleterious levels. SOD mediates scavenging of superoxide radicals into water and hydrogen peroxide and H2O2 produced is subsequently converted into water and oxygen by CAT (Mittler Citation2002). In our results, AMF inoculation increased the activity of the antioxidant enzymes studied thereby mediating quick scavenging of ROS so that metabolic processes are least affected. Increased activities of antioxidant enzymes in AMF plants support the findings of Ghorbanli et al. (Citation2004) and Abdel Latef and Chaoxing (Citation2011) for tomato. In tomato, Qun et al. (Citation2007) reported that salinity-stressed AMF-inoculated seedlings maintained higher activities of SOD, CAT and POD as compared to salt-stressed uninoculated seedlings. Similar results have been observed by Tang et al. (Citation2009) in maize. AMF-induced increase in CAT activity has been reported in soybean (Ghorbanli et al. Citation2004). GR is among active and important components of ascorbate–glutathione pathway where the net electron flow is from NADPH to H2O2 resulting in conversion of H2O2 into water (Mittler Citation2002). Increased GR activity results in enhanced glutathione (reduced) production which acts as electro donor during the conversion of dehydroascorbate into ascorbic acid (Mittler Citation2002). Upregulation of GR maintains higher ratio of GSH/GSSG and NADP+/NADPH. Higher ratio of NADP+/NADPH reduces the formation of superoxide radicals through maintaining the photosynthetic electron transport (Noctor & Foyer Citation1998; Mittler Citation2002).
Salinity stress induced increase in H2O2 production corroborate with the findings of Ahmad (Citation2010) and Ahmad et al. (Citation2012), who in mustard demonstrated that H2O2 production enhanced considerably due to exposure to salinity with concomitant increase in membrane leakage. Tuna et al. (Citation2008) confirmed that salinity enhances membrane leakage. Increased production of H2O2 causes disturbances in cellular homeostasis. AMF-treated plants showed lesser production of H2O2 which is possibly because of the increased activity of antioxidants in AMF-inoculated plants. In our results, increased peroxidation of membrane lipids in salt-stressed plants is in concurrence with the observations of Ahmad (Citation2010) for mustard and Rasool et al. (Citation2013) for chick pea. Exposure to salt stress enhances the production and accumulation of ROS. ROS cause peroxidation of unsaturated lipid component of membranes resulting in the loss of integrity, and hence leakage and desiccation. Present findings strongly support the ameliorative role of AMF in protecting the membrane lipids from peroxidation. Reduced MDA content in AMF-inoculated plants may be due to the substantial increase in antioxidant activities and phosphate metabolism (Qun et al. Citation2007; Tang et al. Citation2009).
Drastic decline in activities of photosynthetic carbon metabolizing enzymes Rubisco and NADP-dependent malic enzyme was obvious in salt-stressed P. turgidum seedlings. Similar reports of reduced activity of Rubisco due to salinity stress have earlier been reported by Soussi et al. (Citation1998) in C. arietinum L. and Fang et al. (Citation2013) in Ulva linza. Reduction in Rubisco activity is possibly due to its sensitivity to chloride ions (Seeman & Critchley Citation1985). In P. turgidum, reduced Rubisco activity due to salinity has been earlier demonstrated by Koyro et al. (Citation2013). Inoculation of AMF induced the activity of NADP-ME resulting in enhanced stress tolerance. Earlier enhanced NADP-ME activity has been reported to be correlated with enhanced stress tolerance. For example, in Oryza sativa, it has been demonstrated that exposure of stress enhances the transcript levels of NADP-ME (Cheng & Long Citation2007; Liu et al. Citation2007). NADP malic enzymes are believed to participate in several important metabolic pathways and plant defense through malate metabolism (Casati et al. Citation1999). Enhanced activity of NADP-ME in AMF-inoculated plants contributes to stress tolerance through enhancing carbon metabolism. PPDK, PEPC and malate dehydrogenase are among the key enzymes regulating carbon metabolism in C4 plants. Our results of increased activities of PPDK, PEPC and MDH in salt-stressed plants corroborate with the findings of Fang et al. (Citation2013) for U. linza. Winter (Citation1974) also reported induction of PEPC activity in water-stressed Mesembryanthemum crystallinum L. Higher activities of C4 enzymes is believed to be essential component for optimal growth under stress conditions (Doubnerova & Ryslava Citation2011).
Increase in accumulation of proline observed in our study corroborate with the findings of Ahmad (Citation2010), Azooz et al. (Citation2011) and Rasool et al. (Citation2012). Increased synthesis of GB in salt-stressed P. turgidum seedlings reported in our study support the findings of Ahmad (Citation2010) and Khan et al. (Citation2014). In Vigna radiata, Khan et al. (Citation2014) observed that enhanced GB accumulation results in improved salt tolerance and growth with subsequent increment in activity of photosynthetic attributes. GB is believed to stabilize the quaternary protein structures against the damaging effects of salt stress (Papageorgion & Murata Citation1995). In addition, GB protects several important components of photosynthetic apparatus including Rubisco and photosystem II from the deleterious impact of high salinity (Hossain & Fujita Citation2010). High salinity causes dissociation and inactivation of important photosynthetic components like Rubisco and PSII into subunits, and hence causing obstructions in normal photosynthetic pathway (Papageorgion & Murata Citation1995; Khan et al. Citation2014). GB has been reported to maintain higher activities of antioxidant enzymes, and hence lowering the levels of H2O2 (Hossain & Fujita Citation2010). Increased proline accumulation reported in our study may be due to the enhancement in the activity of proline synthesizing enzymes and reduction in catabolizing ones or its restricted incorporation during protein synthesis. Altered activities of proline synthesizing and proline degrading enzymes under salt-stress conditions has been observed by Ahmad et al. (Citation2010) and Jaleel et al. (Citation2007). Proline and GB help in maintaining the water balance of plants so that the stress-induced ravage is allayed (Ahmad Citation2010; Ahanger et al. Citation2014b). Proline influences protein turn over and directly regulates stress protective proteins (Thakur & Sharma Citation2005). AMF inoculation enhanced proline and GB accumulation. Further increment in proline and GB in AMF-inoculated salinity-stressed plants suggests the beneficial role of AMF in enhancing the stress tolerance by contributing to maintenance of cellular water content. Increased accumulation of compatible organic solutes is one of the important traits determining the tolerance potential of plant. Our findings of increase in proline due to AMF are in support of the finding of Jindal et al. (Citation1993) for V. radiata and Shekoofeh et al. (Citation2012) for Ocimum basilicum. Colonization of AMF induces stress tolerance by inducing different degree of osmotic adjustment.
Phenolics encompass several groups of secondary metabolites which are implemented in several important functioning like defense. Phenols are included within the nonenzymatic antioxidants which carry the scavenging of toxic radicals and are involved in the interactions with several biotic and abiotic factors (Michalak Citation2006; Bartwal et al. Citation2013; Tomar & Agarwal Citation2013). Phenols mediate amelioration of stress through their role in protecting oxidative stress and increasing membrane stability (Michalak Citation2006; Khattab Citation2007). Enhanced synthesis of phenolics under stressful conditions considerably averts the stress-induced deleterious changes on important molecules like proteins. Similar to our results in Anethum graveolens L., Mehr et al. (Citation2012) also demonstrated enhanced accumulation of phenols with increasing salt concentration. Greater accumulation of phenolics in plants subjected to stressful conditions is ascribed to the upregulation of the enzymes involved in their synthesis (Wada et al. Citation2014). In our study, increased phenols content in stressed plants corroborate with the findings of Mehr et al. (Citation2012) for A. graveolens L., Tomar and Agarwal (Citation2013) for wheat and Dawood et al. (Citation2014) for Vicia faba. In confirmation with our results, Nell et al. (Citation2009) also observed increased phenols content in AMF-inoculated Salvia officinalis L. plants as compared to uninoculated counterparts. Enhanced synthesis of phenols due to AMF inoculation under normal as well as stress conditions strongly supports the protective role of phenols in mitigating negative impact stressful environmental conditions.
Exposure of plants to salt stress results in impeded uptake of essential mineral elements. Our results of salinity-induced reduction in uptake of potassium and phosphorous in P. turgidum seedlings corroborate with the findings of Ghazi and Al-Karaki (Citation2000) for tomato and Kohler et al. (Citation2009) for lettuce. Increased sodium ion concentration within the root zone has direct influence on the uptake of several essential elements like potassium. Sodium shares antagonistic relationship with potassium (Kohler et al. Citation2009). AMF-inoculated seedlings showed lesser contents of sodium as compared to the control counterparts. Moreover, enhanced uptake of potassium and phosphorous as well as subsequent amelioration of salinity-induced restricted uptake of potassium and phosphorous observed in our study support the findings of Ghazi and Al-Karaki (Citation2000), Kohler et al. (Citation2009) and Wu et al. (Citation2010). Wu et al. (Citation2010) demonstrated that AMF inoculation to salt-stressed citrus plants considerably mitigated the deleterious impact on the uptake of essential elements like phosphorous, potassium and calcium. In lettuce plants subjected to severe salt stress, Kohler et al. (Citation2009) also demonstrated that inoculation of AMF contributed significantly to growth maintenance by mediating enhanced uptake of essential mineral elements as compared to the uninoculated counterparts. Greater concentration of Na+ in cellular tissue hampers several growth and metabolic processes in plants. Maintaining higher K/Na ratio is believed to be important strategy adopted by plants to mitigate stress-induced deleterious changes (Wu et al. Citation2010; Azooz et al. Citation2011; Tomar & Agarwal Citation2013). Optimal concentration of potassium is essential for several important metabolic processes (Tomar & Agarwal Citation2013; Ahmad et al. Citation2014). Efficient compartmentation or exclusion of deleterious ions like Na contributes to maintenance of tissue osmotic potential so that hyper-osmotic effects can be averted. In the present study, enhanced uptake of phosphorous, potassium and calcium due to AMF may be as result of selective absorption of these essential ions over deleterious sodium ion and hence resulting in maintaining lower Na/K ratio.
Conclusion
Salinity resulted in altered growth of P. turgidum due to its obvious effects on the physiological and biochemical parameters studied. Salinity increased production of hydrogen peroxide and lipid peroxidation causing loss of membrane integrity and at the same time also reduced the uptake of important mineral elements. In the present study, AMF ameliorated the negative impact of salinity on physio-biochemical parameters studied. AMF alleviated the salt stress-induced changes by its active involvement in antioxidant defense system, thereby protecting cells from oxidative damage. Further preventing the excess uptake of Na+ and enhancing uptake of important ions confer the role of AMF in ensuring better growth under stress conditions. Besides this, AMF also increased proline and GB synthesis which have direct impact on the osmoregulation of the plants. Our study showed that salt stress-induced toxic effects on growth, antioxidant system and mineral nutrients in P. turgidum can be alleviated by AMF and the application of AMF technology is a practical option that could be used as tool for improving crop production under stress condition.
Acknowledgement
The authors would like to extend their sincere appreciation to the Deanship of Scientific Research at king Saud University for its funding this Research group NO. (RG 1435-014).
Disclosure statement
No potential conflict of interest was reported by the authors.
References
- Abdel Latef AAH, Chaoxing H. 2011. Effect of arbuscular mycorrhizal fungi on growth, mineral nutrition, antioxidant enzymes activity and fruit yield of tomato grown under salinity stress. Sci. Hort. 127:228–233.
- Ahanger MA, Hashem A, Abd Allah EF, Ahmad P. 2014a. Arbuscular mycorrhiza in crop improvement under environmental stress. In: Ahmad P, Rasool S, editors. Emerging technologies and management of crop stress tolerance; Vol. 2. Waltham, MA: Elsevier; p. 69–95. Available from: http://dx.doi.org/10.1016/B978-0-12-800875-1.00003-X
- Ahanger MA, Tyagi SR, Wani MR, Ahmad P. 2014b. Drought tolerance: role of organic osmolytes, growth regulators, and mineral nutrients. In: Ahmad P, Wani MR, editors. Physiological mechanisms and adaptation strategies in plants under changing environment; Vol. 1. New York, NY: Springer; p. 25–55.
- Ahmad P. 2010. Growth and antioxidant responses in mustard (Brassica juncea L.) plants subjected to combined effect of gibberellic acid and salinity. Arch. Agro. Soil Sci. 56(5):575–588.
- Ahmad P, Ashraf M, Azooz MM, Rasool S, Akram NA. 2014. Potassium starvation induced oxidative stress and antioxidant defense responses in Brassica juncea. J. Plant Int. 9(1):1–9.
- Ahmad P, Hakeem KR, Kumar A, Ashraf M, Akram NA. 2012. Salt induced changes in photosynthetic activity and oxidative defense system of three cultivars of mustard (Brassica juncea L.). Afr. J. Biotechnol. 11(11):2694–2703.
- Ahmad P, Jaleel CA, Sharma S. 2010. Antioxidant defense system, lipid peroxidation, proline metabolizing enzymes, and biochemical activities in two Morus alba genotypes subjected to NaCl stress. Russian J. Plant Physiol. 57(4):509–517.
- Ahmad P, Sharma S. 2008. Salt stress and phyto-biochemical responses of plants – a review. Plant Soil Environ. 54(3):89–99.
- Alqarawi AA, Abd Allah EF, Hashem A. 2014. Alleviation of salt-induced adverse impact via mycorrhizal fungi in Ephedra aphylla Forssk. J. Plant Interact. 9(1):802–810.
- Arnon DI. 1949. Copper enzymes in isolated chloroplast polyphenol oxidase in Beta vulgaris. Plant Physiol. 24:1–15.
- Aroca R, Ruiz-Lozano JM, Zamarreno A, Paz JA, Garcia-Mina JM, Pozo MJ, Lopez-Raez JA. 2013. Arbuscular mycorrhizal symbiosis influences strigolactone production under salinity and alleviates salt stress in lettuce plants. J. Plant Physiol. 170:47–55.
- Asghari HR, Marschner P, Smith SE, Smith FA. 2005. Growth response of Atriplex nummularia to inoculation with arbuscular mycorrhizal fungi at different salinity levels. Plant Soil. 273:245–256.
- Azooz MM, Youssef AM, Ahmad P. 2011. Evaluation of salicylic acid (SA) application on growth, osmotic solutes and antioxidant enzyme activities on broad bean seedlings grown under diluted seawater. Int. J. Plant Physiol. Biochem. 3(14):253–264.
- Bartwal A, Mall R, Lohani P, Guru SK, Arora S. 2013. Role of secondary metabolites and brassinosteroids in plant defense against environmental stresses. J. Plant Growth Regul. 32:216–232.
- Bates LS, Waldren RP, Teare ID. 1973. Rapid determination of free proline for water stress studies. Plant Sci. 39:205–207.
- Bradford MM. 1976. A rapid and sensitive for the quantitation of microgram quantities of protein utilizing the principle of protein-dye binding. Anal. Biochem. 72:248–254.
- Carlberg I, Mannervik B. 1985. Glutathione-reductase. Methods Enzymol. 113:484–490.
- Carmak I, Horst JH. 1991. Effects of aluminum on lipid peroxidation, superoxide dismutase, catalase, and peroxidase activities in root tips of soybean (Glycine max). Physiol. Plant. 83:463–468.
- Casati P, Drincovich MF, Edwards GE, Andreo CS. 1999. Malate metabolism by NADP-malic enzyme in plant defense. Photosynth. Res. 61:99–105.
- Chance M, Maehly AC. 1955. Assay of catalases and peroxidases. Methods Enzymol. 2:764–775.
- Cheng Y, Long M. 2007. A cytosolic NADP-malic enzyme gene from rice (Oryza sativa L.) confers salt tolerance in transgenic Arabidopsis. Biotechnol. Lett. 29:1129–1134.
- Daniels BA, Skipper HD. 1982. Methods for the recovery and quantitative estimation of propagules from soil. In: Schenck NC, editor. Methods and principles of mycorrhizal research. St. Paul, MN: The American Phytopathological Society; p. 29–36.
- Dawood MG, Taie HAA, Nassar RMA, Abdelhamid M, Schmidhalter U. 2014. The changes induced in the physiological, biochemical and anatomical characteristics of Vicia faba by the exogenous application of proline under seawater stress. South Afr. J. Bot. 93:54–63.
- Doganlar ZB, Demir K, Basak H, Gul I. 2010. Effects of salt stress on pigment and total soluble protein contents of the three different tomato cultivars. Afr. J. Agri. 5(15):2056–2065.
- Doubnerova V, Ryslava H. 2011. What can enzymes of C4 photosynthesis do for C3 plants under stress. Plant Sci. 180:575–583.
- Du YC, Nose A, Kawamitsu Y, Murayama S, Wasano K, Uchida Y. 1996. An improved spectrophotometric determination of the activity of ribulose 1,5-bisphosphate carboxylase. Jpn J. Crop Sci. 65:714–721.
- El-Tayeb MA. 2005. Response of barley grains to the interactive effect of salinity and salicylic acid. Plant Growth Regul. 45:215–224.
- Fang XJ, Wen ZX, Hao YN, Zhou Z, Li MS, Tao DM, Dong XU, Lai MJ. 2013. Activities of principal photosynthetic enzymes in green macroalga Ulva linza: functional implication of C4 pathway in CO2 assimilation. Sci. China Life Sci. 56(6):571–580.
- Fatma M, Masood MAA, Khan NA. 2014. Excess sulfur supplementation improves photosynthesis and growth in mustard under salt stress through increased production of glutathione. Environ. Exp. Bot. 107:55–63.
- Fukayama H, Tsuchida H, Agarie S, Nomura M, Onodera H, Ono K, Lee BH, Hirose S, Toki S, Ku MSB et al. Significant accumulation of C4-specific Pyruvate, orthophosphate dikinase in a C3 plant, rice. Plant Physiol. 127:1136–1146.
- Ghazi N, Al-Karaki. 2000. Growth of mycorrhizal tomato and mineral acquisition under salt stress. Mycorrhiza. 10:51–54.
- Ghorbanli M, Ebrahimzadeh H, Sharifi M. 2004. Effects of NaCl and mycorrhizal fungi on antioxidative enzymes in soybean. Biol. Plant. 48(4):575–581.
- Giannopolitis CN, Ries SK. 1977. Superoxide dismutase. I. Occurrence in higher plants. Plant Physiol. 59:309–314.
- Grieve CM, Grattan SR. 1983. Rapid assay for determination of water soluble quaternary ammonium compounds. Plant Soil 70(2):303–307.
- Hajiboland R, Aliasgharzadeh A, Laiegh SF, Poschenrieder C. 2010. Colonization with arbuscular mycorrhizal fungi improves salinity tolerance of tomato (Solanum lycopersicum L.) plants. Plant Soil. 331:313–327.
- Hameed A, Egamberdieva D, Abd-Allah EF, Hashem A, Kumar A, Ahmad P. 2014. Salinity stress and arbuscularmycorrhizal symbiosis in plants. In: Miransari M, editor. Use of microbes for the alleviation of soil stresses; Vol. 1. New York, NY: Springer; p. 139–159.
- Hashem A, Abd-Allah EF, Alqarawi AA, El-Didamony G, Alwhibi Mona S, Egamberdieva D, Ahmad P. 2014. Alleviation of adverse impact of salinity on faba bean (Vicia faba L.) by arbuscular mycorrhizal fungi. Pak. J. Bot. 46(6):2003–2013.
- Hoagland DR, Arnon DI. 1950. The water-culture method for growing plants without soil. Calif. Agric. Exp. Station Circular. 347:1–32.
- Hodge A, Storer K. 2015. Arbuscular mycorrhiza and nitrogen: implications for individual plants through to ecosystems. Plant Soil. 386:1–19.
- Hossain MA, Fujita M. 2010. Evidence for a role of exogenous glycine betaine and proline in antioxidant defense and methylglyoxal detoxification systems in mung bean seedlings under salt stress. Physiol. Mol. Biol. Plants. 16:19–29.
- Jackson ML. 1962. Soil sampling. In: Jackson ML, editor. Soil chemical analysis. Soil chemical analysis. New York, NY: Prentice Hall; p. 263–268.
- Jaleel CA, Gopi R, Sankar B, Manivannan P, Kishorekumar A, Sridharan R, Panneerselvam R. 2007. Studies on germination, seedling vigour, lipid peroxidation and proline metabolism in Catharanthus roseus seedlings under salt stress. South Afr. J. Bot. 73:190–195.
- Jindal V, Atwal A, Sekhon BS, Rattan S, Singh R. 1993. Effect of vesicular-arbuscular mycorrhizae on metabolism of moong plants under NaCl salinity. Plant Physiol. Biol. 31:475–481.
- Julkenen-Titto R. 1985. Phenolic constituents in the leaves of northern willows: methods for the analysis of certain phenolics. J. Agric. Food Chem. 33:213–217.
- Kanai R, Edwards GE. 1973. Separation of mesophyll protoplasts and bundle sheath cells from maize leaves for photosynthetic studies. Plant Physiol. 51:1133–1137.
- Kaya C, Ashraf M, Sonmez O, Aydemir S, Tuna LA, Cullu AM. 2009. The influence of arbuscular mycorrhizal colonisation on key growth parameters and fruit yield of pepper plants grown at high salinity. Sci. Hort. 121:1–6.
- Khan MA, Ansari R, Ali H, Gul B, Nielsen BL. 2009. Panicum turgidum, a potentially sustainable cattle feed alternative to maize for saline areas. Agric. Ecosyst. Environ. 129:542–546.
- Khan MA, Qaiser M. 2006. Halophytes of Pakistan: characteristics, distribution and potential economic usages. In: Khan MA, Boer B, Kust GS, Barth HJ, editors. Sabkha ecosystems, tasks for vegetation science; Vol. 34. Dordrecht: Springer, p. 129–153.
- Khan MIR, Asgher M, Khan NA. 2014. Alleviation of salt-induced photosynthesis and growth inhibition by salicylic acid involves glycine betaine and ethylene in mungbean (Vigna radiata L.). Plant Physiol. Biol. 80:67–74.
- Khattab H. 2007. Role of glutathione and polyadenylic acid on the oxidative defense systems of two different cultivars of canola seedlings grown under saline conditions. Aust. J. Basic Appl. Sci. 1:323–334.
- Koca M, Bor M, Ozdemir F, Turkan I. 2007. The effect of salt stress on lipid peroxidation, antioxidative enzymes and proline content of sesame cultivars. Environ. Exp. Bot. 60:344–351.
- Kohler J, Hernandez JA, Caravaca F, Roldan A. 2009. Induction of antioxidant enzymes is involved in the greater effectiveness of a PGPR versus AM fungi with respect to increasing the tolerance of lettuce to severe salt stress. Environ. Exp. Bot. 65:245–252.
- Koyro HW, Hussain T, Huchzermeyer B, Khan MA. 2013. Photosynthetic and growth responses of a perennial halophytic grass Panicum turgidum to increasing NaCl concentrations. Environ. Exp. Bot. 91:22–29.
- Levitt J. 1980. Responses of plants to environmental stresses; Vol. 2. New York, NY: Academic Press.
- Liu L, Sun H, Chen J, Zhang Y, Li D, Li C. 2014. Effects of cadmium (Cd) on seedling growth traits and photosynthesis parameters in cotton. Plant Omic. J. 7(4):284–290.
- Liu S, Cheng Y, Zhang X, Guan Q, Nishiuchi S, Hase K, Takano T. 2007. Expression of an NADP-malic enzyme gene in rice (Oryza sativa L) is induced by environmental stresses; over-expression of the gene in Arabidopsis confers salt and osmotic stress tolerance. Plant Mol. Biol. 64:49–58.
- Mehr ZS, Khajeh H, Bahabadi SE, Sabbagh SK. 2012. Changes on proline, phenolic compounds and activity of antioxidant enzymes in Anethum graveolens L. under salt stress. Int. J. Agron. Plant Prod. 3:710–715.
- Michalak A. 2006. Phenolic compounds and their antioxidant activity in plants growing under heavy metal stress. Pol. J. Environ. Stud. 15(4):523–530.
- Miranda D, Fischer G, Ulrichs C. 2011. The influence of arbuscular mycorrhizal colonization on the growth parameters of cape gooseberry (Physalis peruviana L.) plants grown in a saline soil. J. Soil Sci. Plant Nutr. 11(2):18–30.
- Mittal S, Kumari N, Sharma V. 2012. Differential response of salt stress on Brassica juncea: photosynthetic performance, pigment, proline, D1 and antioxidant enzymes. Plant Physiol. Biochem. 54:17–26.
- Mittler R. 2002. Oxidative stress, antioxidants and stress tolerance. Trends Plant Sci. 7:405–410.
- Naguib MI. 1964. Effect of seivn on the carbohydrate and nitrogen metabolism during the germination of cotton seeds. Ind. J. Agric. Sci. 35:179–185.
- Nell M, Votsch M, Vierheilig H, Steinkellner S, Zitterl-Eglseer K, Franza C, Novaka J. 2009. Effect of phosphorus uptake on growth and secondary metabolites of garden sage (Salvia officinalis L.). J. Sci. Food Agric. 89:1090–1096.
- Nelson N. 1944. Photometric adaptation of Somagi method for the determination of glucose. J. Biol. Chem. 153:375–380.
- Noctor G, Foyer CH. 1998. Simultaneous measurement of foliar glutathione, γ-glutamyl cysteine and amino acids by high-performance liquid chromatography: comparison with two other assay methods for glutathione. Anal. Biochem. 264:98–110.
- Omoto E, Taniguchi M, Miyake H. 2012. Adaptation responses in C4 photosynthesis of maize under salinity. J. Plant Physiol. 169:469–477.
- Papageorgion GC, Murata N. 1995. The unusually strong stabilizing effects of glycine betaine on the structure and function of the oxygen-evolving photosystem II complex. Photosynth. Res. 44:243–252.
- Porcel R, Aroca R, Ruiz-Lozano JM. 2012. Salinity stress alleviation using arbuscular mycorrhizal fungi: a review. Agron. Sust. Dev. 32:181–200.
- Qun HZ, Xing HC, Bin ZZ, Rong ZZ, Song WH. 2007. Changes of antioxidative enzymes and cell membrane osmosis in tomato colonized by arbuscular mycorrhizae under NaCl stress Coll Sur B. Biointerfaces 59:128–133.
- Rasool S, Ahmad A, Siddiqi TO. 2012. Differential response of Chickpea genotypes under salt stress. J. Funct. Environ. Bot. 2(1):59–64.
- Rasool S, Ahmad A, Siddiqi TO, Ahmad P. 2013. Changes in growth, lipid peroxidation and some key antioxidant enzymes in chickpea genotypes under salt stress. Acta Physiol. Plant. 35:1039–1050.
- Ruiz-Lozano JM, Collados C, Barea J, Azcon MR. 2001. Arbuscular mycorrhizal symbiosis can alleviate drought induced nodule senescence in soybean plants. Plant Physiol. 82:346–350.
- Ruiz-Lozano JM, Porcel R, Azcon R, Aroca R. 2012. Regulation by arbuscular mycorrhizae of the integrated physiological response to salinity in plants. New challenges in physiological and molecular studies. J. Exp. Bot. 63:4033–4044.
- Said A, Naguib MI, Ramzy MA. 1964. Sucrose determination as a means of the “Darw back tax” exported Halawa Tehinia. Bull. Fac. Sci. Cairo. Univ. 39:209–214.
- Scheibe R, Stitt M. 1988. Comparison of NADP-malate dehydrogenase activation, QA reduction and O2 evolution in spinach leaves. Plant Physiol. Biochem. 26:473–481.
- Seeman JR, Critchley C. 1985. Effects of salt stress on growth, ion content, stomatal behaviour and photosynthetic capacity of salt-sensitive species Phaseolus vulgaris (L). Planta. 164:151–62.
- Shekoofeh E, Sepideh H, Roya R. 2012. Role of mycorrhizal fungi and salicylic acid in salinity tolerance of Ocimum basilicum resistance to salinity. J. Biot. 11(9):2223–2235.
- Sheng M, Tang M, Chan H, Yang B, Zhang F, Huang Y. 2008. Influence of arbuscular mycorrhizae on photosynthesis and water status of maize plants under salt stress. Mycorrhiza. 18:287–296.
- Sherstha YH, Ishii T, Kadoya K. 1995. Effect of vesicular–arbuscular mycorrhizal fungi on the growth, photosynthesis, transpiration and the distribution of photosynthates of bearing Satsuma mandarin trees. J. Jpn. Soc. Hortic. Sci. 64:517–525.
- Soussi M, Ocana A, Lluch C. 1998. Effects of salt stress on growth, photosynthesis and nitrogen fixation in chick-pea (Cicer arietinum L.). J. Exp. Bot. 49(325):1329–1337.
- Sultana N, Ikeda T, Itoh R. 1999. Effect of NaCl salinity on photosynthesis and dry matter accumulation in developing rice grains. Environ. Exp. Bot. 42:211–220.
- Tang M, Chen H, Huang JC, Tian ZQ. 2009. AM fungi effects on the growth and physiology of Zea mays seedlings under diesel stress. Soil Biol. Biochem. 41:936–940.
- Thakur M, Sharma AD. 2005. Salt stress induced proline accumulation in germinating embryos: evidence suggesting a role of proline in seed germination. J. Arid Environ. 62:517–523.
- Tomar NS, Agarwal RM. 2013. Influence of treatment of Jatropha curcas L. leachates and potassium on growth and phytochemical constituents of wheat (Triticum aestivum L.). Am. J. Plant Sci. 4:1134–1150.
- Tuna AL, Kaya C, Dikilitas M, Higgs D. 2008. The combined effects of gibberellic acid and salinity on some antioxidant enzyme activities, plant growth parameters and nutritional status in maize plants. Environ. Expt. Bot. 62:1–9.
- Utobo EB, Ogbodo EN, Nwogbaga AC. 2011. Techniques for extraction and quantification of arbuscular mycorrhizal fungi. Libyan Agric. Res. Cent. Int. 2:68–78.
- Velikova V, Yordanov I, Edreva A. 2000. Oxidative stress and some antioxidant systems in acid rain-treated bean plants. Protective role of exogenous polyamines. Plant Sci. 151:59–66.
- Wada KC, Mizuuchi K, Koshio A, Kaneko K, Mitsui T, Takeno K. 2014. Stress enhances the gene expression and enzyme activity of phenylalanine ammonia-lyase and the endogenous content of salicylic acid to induce flowering in Pharbitis. J. Plant Physiol. 171:895–902.
- Winter K. 1974. Effect of water stress on phosphoenolpyruvate carboxylase activity in Mesembryanthemum crystallinum (L.). Planta 121(2):147–153.
- Wolf B. 1982. A comprehensive system of leaf analyses and its use for diagnosing crop nutrient status. Comm. Soil Sci. Plant Anal. 13:1035–1059.
- Wu QS, Zou YN, He XH. 2010. Contributions of arbuscular mycorrhizal fungi to growth, photosynthesis, root morphology and ionic balance of citrus seedlings under salt stress. Acta Physiol. Plant. 32:297–304.