ABSTRACT
The search for potent, selective bioherbicides has been the focus of numerous studies for several decades. Developing an economically viable total chemical synthesis procedure has been the challenge for commercial-scale application of these nature-derived chemicals. An efficient and low-cost total synthesis of an allelopathic and antitumor N-trans-cinnamoyltyramine (NTCT) first reported in rice (Oryza sativa L.) was successfully achieved by one-step amidation from trans-cinnamic acid and tyramine. The synthesized NTCT inhibited root and hypocotyl growth of cress (Lepidium sativum L.) and barnyardgrass (Echinochloa crus-galli L.) at concentrations as low as 0.24 μM. The means of ED50 (the effective dose required for 50% plant growth inhibition) levels of the compound on cress and barnyardgrass hypocotyl and root elongations were 0.96 and 0.73 μM, respectively. Potential mechanisms underlying NTCT growth inhibition and its biosynthesis pathway were also suggested. The developed synthesis strategy could permit production of this synthesized allelochemical at a commercial scale as a bioherbicide.
Introduction
For more than 70 years, synthetic herbicides have been developed to meet the weed control needs of agronomic and horticultural crops in industrialized nations. Since the late 1990s, many weed biotypes have evolved resistance to one or more herbicide modes of action (Beckie et al. Citation2000) limiting effective options for growers. In addition, the misuse of several synthetic herbicides has negative implications for human, animal, and environmental health (Vyvyan Citation2002; Macías et al. Citation2006; Macías et al. Citation2007). Development of novel herbicide modes of action has been lacking for more than 16 years; this, combined with more frequent selection of herbicide-resistant weeds, suggests a need to develop weed control technologies based upon natural products (Fürstner Citation2004).
Allelochemicals are structurally complex and can pose significant challenges for synthesis by organic chemists (Dayan & Duke Citation2014). Isolation, structural elucidation, and synthesis of natural products are paramount to identification of new molecules with biological activity for controlling weeds (TAKIKAWA Citation2006). In some instances, the source for allelochemicals can be found in crops for which weed control can be challenging. For example, cultivated rice Oryza sativa L. ‘OM 5930’ is allelopathic against barnyardgrass, a troublesome weed in rice production (Thi et al. Citation2014a). Among the many compounds isolated from rice, β-phenylethylamine as N-trans-cinnamoyltyramine (NTCT) from OM 5930 was identified as a potent plant growth inhibitor (Thi et al. Citation2014b). The β-phenylethylamines have been detected in a wide variety of different plant species, as well as fungi and bacteria (Kim et al. Citation2012). These compounds result from decarboxylation of phenylalanine, and are known to have important roles in plant–plant, plant–pathogen, plant–insect, and plant–environment interactions (Negrel et al. Citation1993; Schraudner et al. Citation1993; Lajide et al. Citation1995; Cutillo et al. Citation2003).
Compound NTCT was also reportedly found in nightshade (Lycianthes biflora) and exhibited inhibitory effects on a murine P388 leukemia line (Yang et al. Citation2002). More recently, compound NTCT has been isolated in UV-treated rice leaves as a potent anti-cancer agent (Park et al. Citation2013).
Potential development of compound NTCT for field-scale applications must address the lack of economically viable methods for synthesis. This challenge is common for commercial-scale development of many naturally based allelopathic chemicals (Bhadoria Citation2010). The objectives of this study were to: (i) develop an efficient, low-cost, and scalable synthesis of compound NTCT; (ii) evaluate the allelopathic activity of synthetic compound NTCT; and (iii) describe possible inhibition mechanisms and a biosynthesis pathway.
Materials and methods
General experimental procedures
Compounds 2,3,N,N′-dicyclohexylcarbodiimide (C6H11N9C9NC6H11) and 4-(dimethylamino) pyridine(C7H10N2) were purchased from Sigma-Aldrich and used without additional purification. 1H and 13C NMR spectra were recorded at 400 MHz on a Varian Mercury-VX spectrometer with DMSO-d6 as the solvent and tetramethylsilane as the internal standard. High performance liquid chromatography (HPLC) was performed with a C18 HPLC column (4.6 mm i.d.×150 mm, Kinetex 2.6 μm C8 100Å; Phenomenex Co., Golden, CO, U.S.A) on a Shimadzu CBM-20A HPLC system coupled with a Shimadzu LC-20AT pump, SPD-20AV UV/VIS detector, CTO-20A column oven and LC-20AT microfractionation system (Shimadzu Co., Columbia, MD, U.S.A).
Liquid chromatography–mass spectrometry coupling with electrospray ionization (LCMS-ESI) analysis was conducted on a Shimadzu LCMS 2010A using an Acclaim 120 C18 reversed-phase LC column (4.6 × 50 mm) (Thermo Scientific) with a gradient of 10–95% CH3CN–H2O (v/v) (with 0.1% trifluoro acetic acid) at a flow rate 0.8 mL min−1 in 10 min. Compound NTCT was dissolved in DMSO and the UV detector was set at 300 nm.
Synthesis of NTCT
Synthesis was achieved by a one-step amidation procedure revised from that described by Yang et al. (Citation2011) (). A solution of trans-cinnamic acid (0.98 g, 6.6 mmol) in dry dichloromethane (300 mL) was pre-activated with N,N′-dicyclohexylcarbodiimide (1.50 g, 7.3 mmol) () in the presence of the base 4-(dimethylamino) pyridine (C7H10N2) (0.033 g, 0.3 mmol) at room temperature for 30 min. The solids resulting from this reaction were dissolved in dichloromethane; tyramine (1.36 g, 9.9 mmol) () was then added to initiate amidation. The solution was stirred overnight at room temperature and volatiles were removed under reduced pressure. The residue was purified via normal phase flash chromatography with ethyl acetate/CH2Cl2 (1:5) as an eluent. Eluent fractions with the desired product were collected and concentrated on a rotary evaporator to afford NTCT (1.14 g, 64.3% yields) as a white solid. Compound NTCT was further purified by analytical reversed-phase HPLC using CH3CN/H2O (20:80), (30:70), (55:45) and subsequently (20:80) over a period of 35 min at the flow rate of 1.0 mL min−1, yielding compound NTCT with high purity (>95% by HPLC). A sample of NTCT was run on silica thin-layer chromatography developed with ethyl acetate/CH2Cl2 (1:5) (Rf = 0.23). Examination of the purified synthesis product NTCT by NMR provided the following results: 1H NMR (500 MHz, CDCl3) of NTCT: δ (ppm) 7.60 (d, 1H, J = 15.5 Hz), 7.46–7.48 (m, 2H), 7.33–7.30 (m, 3H), 7.08 (d, 2H, J = 8.5 Hz), 6.79 (d, 2H, J = 8.5 Hz), 6.30 (d, 1H, J = 15.5 Hz), 5.54 (s, 1H), 4.75 (s, 1H), 3.61 (t, 2H, J = 7 Hz), 2.81 (t, 2H, J = 7 Hz). 13C NMR (125 MHz, CDCl3) of NTCT: δ (ppm) 165.81, 154.26, 141.12, 134.80, 130.94, 129.95, 129.68, 128.80, 127.78, 120.53, 115.54, 40.96, and 34.75 (Figures S1 and S2, Supporting Information).
Bioassay
NTCT (5 mg) was dissolved in 5 mL methanol, and concentrations of 0.024, 0.048, 0.24, 0.48, 0.96, 2.4, and 4.8 μM were added onto a sheet of filter paper in the wells of 24-well plates (Corning HTS transwell-24 systems, 0.33 cm2/well, Thermo Fisher Scientific Ltd. Co, U.S.A). The bioassays on cress and barnyardgrass were conducted twice using a completely randomized design with four replications following the bioassay procedure described by Thi et al. (Citation2014b).
Statistical analysis
The analysis followed the method described by Thi et al. (Citation2014b). Briefly, all root or hypocotyl length data were transformed to a percentage of control as determined by the following formula: Percentage of control R = L/Lcontrol×100%, where R represents the length ratio, Lcontrol represents the average length of roots or hypocotyls of control plants, and L represents the average length of roots or hypocotyls of treated plants. Three-parameter logistic equation F(x) = d/{1+exp[b(log(x)–log(e)]}F(x) = d/{1+exp[b(log(x)–log(e))]} from dose–response curve package was used to identify ED50, where F displays the growth inhibition (%), ×indicates the concentration (μM), and e represents the ED50 (Knezevic et al. Citation2007).
Duncan’s multiple range tests at P ≤ .001 were conducted to separate treatment and ED50 for the biological activity of compound NTCT.
Results and discussion
Synthesized and natural NTCT
The synthesized NTCT was characterized relative to the natural NTCT isolated by Thi et al. (Citation2014b) using 13C NMR spectroscopy (Figures S1 and S2, Supporting Information) and LCMS-ESI analysis. LCMS-ESI analysis confirmed that the retention time of the synthesized NTCT is 3.595 min (the same as the natural one) with m/z 268 [M+H]+ (C17H17NO2, 267.3) (Figures S3 and S4, Supporting Information). Characterization by 13C NMR spectroscopy revealed general agreement of 17 carbon positions between synthesized NTCT (A) and natural NTCT (B) isolated from rice as follows: C1 (A = 154.4; B = 154.3); C2 (A = 115.5; B = 115.5); C3 (A = 129.9; B = 129.9); C4 (A = 130.9; B = 130.9); C5 (A = 129.9; B = 129.9); C6 (A = 115.5; B = 115.5); C7 (A = 34.7; B = 34.8); C8 (A = 40.9; B = 41.0); C9 (A = 165.6; B = 165.8); C10 (A = 120.6; B = 120.5); C11 (A = 141.0; B = 141.1); C12 (A = 134.8; B = 134.8); C13 (A = 127.8; B = 127.8); C14 (A = 128.8; B = 128.8); C15 (A = 129.6; B = 129.7); C16 (A = 128.8; B = 128.8); and C17 (A = 127.8; B = 127.8) (). Comparisons of LCMS-ESI and 13C NMR analyses for the synthesized and natural NTCT revealed identical structures. Therefore, the two sets of data are in reasonable agreement.
Table 1. The 13C NMR spectroscopic data for the natural N-trans-cinnamoyltyramine and the synthesized N-trans-cinnamoyltyramine.
Compound NTCT () was chemically synthesized by one-step amidation from trans-cinnamic acid and tyramine (). However, biosynthesis of NTCT in rice seedlings as proposed in is more complex; several steps to synthesize trans-cinnamic acid and tyramine from phenylalanine and tyrosine are required, followed by activation by cinnamoyl CoA, and finally conjugation by tyramine N-(hydroxycinnamoyl)-transferase (THT). Compound NTCT is likely derived from metabolites of the aromatic amino acid phenylalanine and tyrosine, which are produced in the shikimate pathway (Herrmann Citation1995). As illustrated in , phenylalanine ammonia-lyase converts phenylalanine into cinnamic acid by deamination (MacDonald & D’Cunha Citation2007). Cinnamoyl CoA is then generated by CoA ligases (Klempien et al. Citation2012). Tyrosine decarboxylase catalyzes the decarboxylation of tyrosine, which results in tyramine (Hosoi et al. Citation1970). The final step in the proposed scheme is the conjugation of cinnamoyl CoA and tyramine catalyzed by the enzyme THT, but THT activity is not specific to cinnamoyl CoA and tyramine. Previously, plant defense compounds, such as coumaroyltyramine and feruloyltyramine (), have been shown to be synthesized in response to pathogen infection and plant wounding. These compounds are conjugated by THT from hydroxycinnamoyl-CoA and tyramine (Back Citation2001), which could be similar to the reactions that generate NTCT.
Allelopathic activity of synthetic NTCT
Inhibition of hypocotyl and root growth of cress and barnyardgrass seedlings was detected with the synthesized NTCT at concentrations as low as 0.24 μM ( and ). At 4.8 μM, inhibition of hypocotyl and root growth reached 92.4% and 95.3% for cress () and 90.6% and 95.6% for barnyardgrass seedlings (), respectively. In comparing activities of the natural versus NTCT on the same test plant species (Thi et al. Citation2014b), the synthesized NTCT was 7.6% and 4.7% less active on cress hypocotyl and root growth than the natural NTCT. One possible explanation for differences in activity is a detected trace impurity in the synthesized NTCT (Materials and methods). For barnyardgrass, the activity of synthesized versus NTCT on hypocotyl growth was 4.8% lower, but inhibitory activity was similar for root growth. The ED50 values of the compound for cress hypocotyls and roots were 0.56 and 0.36 μM ( and ), respectively. The ED50 values of the compound for barnyardgrass hypocotyls and roots were 1.35 and 1.11 μM, respectively ( and ). Root elongation was more sensitive to the synthesized NTCT than shoot elongation and cress was more sensitive to the compound than barnyardgrass ( and ).
Figure 3. Effects of N-trans-cinnamoyltyramine on hypocotyl (shoot) and root growth of cress seedlings. ED50 values represent the effective dose to reduce the representative parameter (shoot or root growth) by 50%. Means followed by the same letter are not significantly different using Duncan’s multiple range test at P ≤ .001).
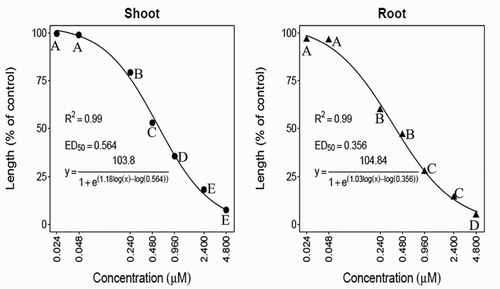
Figure 4. Effects of N-trans-cinnamoyltyramine on hypocotyl (shoot) and root growth of barnyardgrass seedlings. ED50 values represent the effective dose to reduce the representative parameter (shoot or root growth) by 50%. Means followed by the same letter are not significantly different using Duncan’s multiple range test at P ≤ .001.
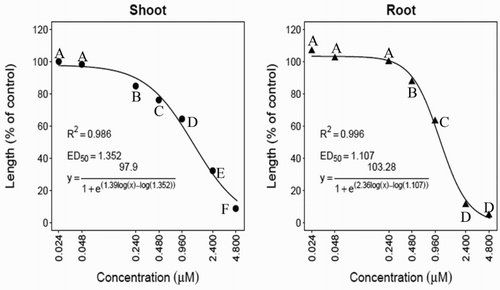
Figure 5. ED50 values of N-trans-cinnamoyltyramine on shoot and root growth of cress and barnyardgrass seedlings (results of repeated studies using four replications). Vertical bars indicate the standard error of the means. Means followed by the same letter are not significantly different using Duncan’s multiple range test at P ≤ .001.
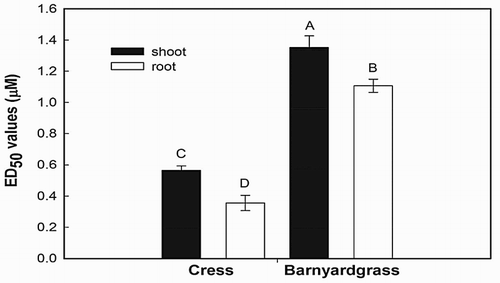
Figure 6. Effects of N-trans-cinnamoyltyramine on hypocotyl and root growth of cress (A) and barnyardgrass (B) seedlings following 48 hours of incubation at various concentrations.
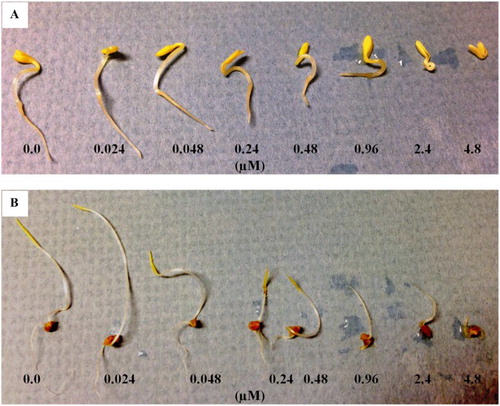
The response of plant species to an allelochemical under laboratory conditions principally depends upon the physiological and biochemical properties of each species. It was reported that the sensitivity of a receiver plant to phytotoxic extracts from a donor plant (allelochemical) is a function of plant organs and growth stage (Wolf et al. Citation1984; Hasegawa et al. Citation1992; Suzuki et al. Citation2001; Iqbal et al. Citation2002). The allelopathic activity of NTCT on cress and barnyardgrass appears similar based upon symptomology and ED50 values. Root growth is more sensitive to NTCT than shoot growth, and cress is more sensitive to NTCT than barnyardgrass (). This might be because roots initially absorb allelochemicals from the environment and the permeability of allelochemicals into root tissue is higher than that in shoot tissue (Nishida et al. Citation2005). Other explanations could include differences between enzyme profiles in shoots versus roots (Dam et al. Citation2008).
Possible inhibition mechanisms and a biosynthesis pathway
In the presence of NTCT, inhibition of cress and barnyardgrass may result from reduced cell division and/or expansion, the two principle components controlling growth (Tefera Citation2002). Although details of the biochemical mechanism underlying inhibition are lacking, inhibition of enzymes contributing to plant growth by allelochemicals has been reported (Sato et al. Citation1982; Santos et al. Citation2004). Interspecific differences may result from many reasons, including from variable responses in membrane potential, auxin dynamics or tyrosine phosphorylation. Plant symptomology in response to NTCT provides clues concerning the mechanisms of action. However, to date, the mechanism of activity for NTCT has not been established. Nonetheless, precursors to NTCT (), such as cinnamic acid and benzoic acid derivatives, alter membrane potential, mineral uptake, chlorophyll content, photosynthesis, carbon flow, and phytohormone activity (Einhellig Citation1994; Blum et al. Citation1999). Phenolic acids, such as cinnamic acid, could play a key role because different phenolic acids strongly inhibit seed germination (Blum et al. Citation1999). Einhellig (Citation1994) and Blum et al. (Citation1999) proposed that phenolic acids depolarized the cell membrane, affecting membrane ATPase activity and ion flux. However, substituted cinnamic acid amide analogues (), which lose their negative charge on the hydroxyl groups, result in greater inhibition of radish (Raphanus sativus) germination than substituted cinnamic acid alone (Vishnoi et al. Citation2009). Inhibition of substituted cinnamic acids (3-hydroxy, 4-hydroxy, 2-nitro, 3-nitro, 4-nitro, 3-chloro, and 4-methoxy) and four different types of substituted anilines (H, 2-chloro,4-methy,4-nito) on radish germination is similar (Vishnoi et al. Citation2009). Therefore, it appears likely that NTCT does not act by depolarizing the cell membrane; rather, other mechanisms underlying root and hypocotyl elongation are impacted.
Several of the cinnamic acid amines exhibit both cytotoxic and phytotoxic properties. In one study, compound NTCT was identified as one of the major cytotoxic agents induced in UV-treated rice leaves (Park et al. Citation2013). Induction of ‘free’ cinnamic acid amines in response to stress factors (antimicrobial, allelopathic) has been reported in different species, including pepper (Capsicum annuum L. cv. Early Calwonder) (Newman et al. Citation2001), tomato (Solanum lycopersicum L.) (López-Gresa et al. Citation2011) and canola (Brassica napus L.) (Jahangir et al. Citation2009). Since synthesis of various phenolic acids and NTCT can be induced by certain stresses (Pitts et al. Citation1998; Bibikova & Gilroy Citation2003), it may also be possible that NTCT accumulates in response to stresses resulting from competition (biological, chemical, or physical).
Auxin is a potent hormone that impacts root hair development (Bibikova & Gilroy Citation2003), and aberrations in auxin availability or signaling can cause defects in root hair growth and morphology (Liu et al. Citation1993; Ni et al. Citation1999). Compound cinnamic acid is also proposed to inhibit polar auxin transport by blocking the transport at the efflux carrier (Ni et al. Citation1999). In turn, reduced availability of auxin decreases root hair growth.
The tyramine moiety in NTCT () may mimic the activity of tyrosine. Hydroxycinnamic acid amides, including coumaroyltyramine and feruloyltyramine (), which contain tyramine moieties, inhibit tyrosinase (enzyme present in plant and animal tissues that catalyzes synthesis of melanin) (Feng et al. Citation1993; Tregear et al. Citation1996; Rudrabhatla et al. Citation2006; Wu et al. Citation2012). As a tyrosine mimic, NTCT may have both a beneficial and detrimental effect on tyrosine kinase. Dual-specific kinases (act as both tyrosine kinase and serine/threonine kinase) have been reported in many plants, including Arabidopsis thaliana ATN1 and soybean GmPK6 (Feng et al. Citation1993; Tregear et al. Citation1996; Rudrabhatla et al. Citation2006; Wu et al. Citation2012). Therefore, it is possible that the tyramine moiety of NTCT could block phosphorylation by dual-specific kinases, ultimately suppressing weed seedling growth.
Differential responses between cress and barnyardgrass as well as among specific tissues (shoot and root) were observed. The specific mechanism(s) of growth inhibition by NTCT are not known to date. However, based upon available literature, NTCT may affect membrane potentials, auxin dynamics and/or could inhibit phosphorylation. Differences in membrane potentials, auxin dynamics or tyrosine phosphorylation may explain variable responses by different species and among tissues within species. The concentration necessary to effectively inhibit plant growth with NTCT approaches the herbicidal activity of dinitroanilines, a family of herbicides that arrest cell division at prometaphase (Smeda & Vaughn Citation1994). Further research is necessary to elucidate if there is a specific mechanism of action of NTCT on plant growth processes.
In conclusion, an efficient one-step synthesis of the naturally occurring allelopathic NTCT was successfully achieved using two commercial precursors. Synthesized NTCT inhibited root and hypocotyl growth of cress and barnyardgrass at micromolar concentrations. Potential modes of action of this compound including effects on membrane potentials, auxin dynamics and/or phosphorylation were proposed. Further research is needed to determine the molecular mechanisms of action and to study selectivity and suitability of bioherbicide.
Supplemental_Material.docx
Download MS Word (758.2 KB)Acknowledgements
The authors thank the Vietnam Education Foundation (VEF. 2111 Wilson Boulevard Suite 700, Arlington, VA 22201, USA) and American Association of University Women (AAUW. 111 Sixteenth St. NW Washington, DC 20036, USA) for supporting this research. The authors also appreciate Dr C. T. Nguyen in computational biology, Plant Sciences Division, University of Missouri, for his assistance in data analysis. This material is based upon work supported by the National Science Foundation under Award Number IIA-1355406. Any opinions, findings, conclusions, or recommendations expressed in this material are those of the author(s) and do not necessarily reflect the views of the National Science Foundation.
Disclosure statement
No potential conflict of interest was reported by the authors.
References
- Back K. 2001. Hydroxycinnamic acid amides and their possible utilization for enhancing agronomic traits. Plant Pathol J. 17:123–127.
- Beckie HJ, Heap IM, Smeda RJ, Hall LM. 2000. Screening for herbicide resistance in weeds. Weed Technol. 14:428–445. doi: 10.1614/0890-037X(2000)014[0428:SFHRIW]2.0.CO;2
- Bhadoria PBS. 2010. Allelopathy: a natural way towards weed management. Am J Exp Agric. 1:7–20.
- Bibikova T, Gilroy S. 2003. Root hair development. J Plant Growth Regul. 21:383–415. doi: 10.1007/s00344-003-0007-x
- Blum U, Shafer SR, Lehman ME. 1999. Evidence for inhibitory allelopathic interactions involving phenolic acids in field soils: concepts vs. an experimental model. Crit Rev Plant Sci. 18:673–693. doi: 10.1016/S0735-2689(99)00396-2
- Cutillo F, D’Abrosca B, DellaGreca M, Di Marino C, Golino A, Previtera L, Zarrelli A. 2003. Cinnamic acid amides from Chenopodium album: effects on seeds germination and plant growth. Phytochemistry. 64:1381–1387. doi: 10.1016/S0031-9422(03)00511-9
- Dam NM, Tytgat TOG, Kirkegaard JA. 2008. Root and shoot glucosinolates: a comparison of their diversity, function and interactions in natural and managed ecosystems. Phytochem Rev. 8:171–186.
- Dayan FE, Duke SO. 2014. Natural compounds as next-generation herbicides. Plant Physiol. 166:1090–1105. doi: 10.1104/pp.114.239061
- Einhellig FA. 1994. Mechanism of action of allelochemicals in allelopathy. Am Chem Soc. 582:96–116.
- Feng X-H, Zhao Y, Bottino PJ, Kung S. 1993. Cloning and characterization of a novel member of protein kinase family from soybean. Biochim Biophys Acta BBA – Gene Struct Expr. 1172:200–204. doi: 10.1016/0167-4781(93)90295-O
- Fürstner A. 2004. Total syntheses and biological assessment of Macrocyclic glycolipids. Eur J Org Chem. 2004:943–958. doi: 10.1002/ejoc.200300728
- Hasegawa K, Mizutani J, Kosemura S, Yamamura S. 1992. Isolation and identification of lepidimoide, a new allelopathic substance from mucilage of germinated cress seeds. Plant Physiol. 100:1059–1061. doi: 10.1104/pp.100.2.1059
- Herrmann K. 1995. The Shikimate pathway: early steps in the biosynthesis of aromatic compounds. Plant Cell. 7:907–919. doi: 10.1105/tpc.7.7.907
- Hosoi K, Yoshida S, Hasegawa M. 1970. L-Tyrosine carboxy-lyase of barley roots. Plant Cell Physiol. 11:899–906. doi: 10.1093/oxfordjournals.pcp.a074581
- Iqbal Z, Hiradate S, Noda A, Isojima S-I, Fujii Y. 2002. Allelopathy of buckwheat: assessment of allelopathic potential of extract of aerial parts of buckwheat and identification of fagomine and other related alkaloids as allelochemicals. Weed Biol Manag. 2:110–115. doi: 10.1046/j.1445-6664.2002.00055.x
- Jahangir M, Abdel-Farid IB, Kim HK, Choi YH, Verpoorte R. 2009. Healthy and unhealthy plants: the effect of stress on the metabolism of Brassicaceae. Environ Exp Bot. 67:23–33. doi: 10.1016/j.envexpbot.2009.06.007
- Kim B, Byun BY, Mah J-H. 2012. Biogenic amine formation and bacterial contribution in Natto products. Food Chem. 135:2005–2011. doi: 10.1016/j.foodchem.2012.06.091
- Klempien A, Kaminaga Y, Qualley A, Nagegowda DA, Widhalm JR, Orlova I, Shasany AK, Taguchi G, Kish CM, Cooper BR, et al. 2012. Contribution of CoA Ligases to benzenoid biosynthesis in petunia flowers. Plant Cell. 24:2015–2030. doi: 10.1105/tpc.112.097519
- Knezevic SZ, Streibig JC, Ritz C. 2007. Utilizing R software package for dose-response studies: the concept and data analysis. Weed Technol. 21:840–848. doi: 10.1614/WT-06-161.1
- Lajide L, Escoubas P, Mizutani J. 1995. Termite antifeedant activity in Xylopia aethiopica. Phytochemistry. 40:1105–1112. doi: 10.1016/0031-9422(95)92653-P
- Liu C, Xu Z, Chua NH. 1993. Auxin polar transport is essential for the establishment of bilateral symmetry during early plant embryogenesis. Plant Cell. 5:621–630. doi: 10.1105/tpc.5.6.621
- López-Gresa MP, Torres C, Campos L, Lisón P, Rodrigo I, Bellés JM, Conejero V. 2011. Identification of defence metabolites in tomato plants infected by the bacterial pathogen Pseudomonas syringae. Environ Exp Bot. 74:216–228. doi: 10.1016/j.envexpbot.2011.06.003
- MacDonald MJ, D’Cunha GB. 2007. A modern view of phenylalanine ammonia lyase. Biochem Cell Biol. 85:273–282. doi: 10.1139/O07-018
- Macías FA, Marín D, Oliveros-Bastidas A, Chinchilla D, Simonet AM, Molinillo JMG. 2006. Isolation and synthesis of allelochemicals from gramineae: benzoxazinones and related compounds. J Agric Food Chem. 54:991–1000. doi: 10.1021/jf050896x
- Macías FA, Molinillo JMG, Varela RM, Galindo JCG. 2007. Allelopathy – a natural alternative for weed control. Pest Manag Sci. 63:327–348. doi: 10.1002/ps.1342
- Negrel J, Javelle F, Paynot M. 1993. Wound-induced tyramine hydroxycinnamoyl transferase in potato (Solanum tuberosum) tuber discs. J Plant Physiol. 142:518–524. doi: 10.1016/S0176-1617(11)80392-5
- Newman M-A, von Roepenack-Lahaye E, Parr A, Daniels MJ, Dow JM. 2001. Induction of hydroxycinnamoyl-tyramine conjugates in pepper by Xanthomonas campestris, a plant defense response activated by hrp gene-dependent and hrp gene-independent mechanisms. Mol Plant Microbe Interact. 14:785–792. doi: 10.1094/MPMI.2001.14.6.785
- Ni DA, Wang LJ, Xu ZH, Xia ZA. 1999. Foliar modifications induced by inhibition of polar transport of auxin. Cell Res. 9:27–35. doi: 10.1038/sj.cr.7290003
- Nishida N, Tamotsu S, Nagata N, Saito C, Sakai A. 2005. Allelopathic effects of volatile Monoterpenoids produced by Salvia leucophylla: inhibition of cell proliferation and DNA synthesis in the root apical meristem of Brassica campestris seedlings. J Chem Ecol. 31:1187–1203. doi: 10.1007/s10886-005-4256-y
- Park J-H, Fu Y-Y, Chung IS, Hahn T-R, Cho M-H. 2013. Cytotoxic property of ultraviolet-induced rice phytoalexins to human colon carcinoma HCT-116 cells. J Korean Soc Appl Biol Chem. 56:237–241. doi: 10.1007/s13765-012-3238-3
- Pitts RJ, Cernac A, Estelle M. 1998. Auxin and ethylene promote root hair elongation in Arabidopsis. Plant J. 16:553–560. doi: 10.1046/j.1365-313x.1998.00321.x
- Rudrabhatla P, Reddy MM, Rajasekharan R. 2006. Genome-wide analysis and experimentation of plant serine/threonine/tyrosine-specific protein kinases. Plant Mol Biol. 60:293–319. doi: 10.1007/s11103-005-4109-7
- Santos WDD, Ferrarese MDLL, Finger A, Teixeira ACN, Ferrarese-Filho O. 2004. Lignification and related enzymes in Glycine max root growth-inhibition by ferulic acid. J Chem Ecol. 30:1203–1212. doi: 10.1023/B:JOEC.0000030272.83794.f0
- Sato T, Kiuchi F, Sankawa U. 1982. Inhibition of phenylalanine ammonia-lyase by cinnamic acid derivatives and related compounds. Phytochemistry. 21:845–850. doi: 10.1016/0031-9422(82)80077-0
- Schraudner M, Langebartels C, Negrel JHS, Jr. 1993. Plant defense reactions induced in tobacco by the air pollutant ozone. In: Fritig B, Legrand M, editors. Mechanisms of plant defense responses. Volume 2 of the series developments in plant pathology. Springer; p. 286–290.
- Smeda RJ, Vaughn KC. 1994. Resistance to dinitroaniline herbicides. In: Powles SB, Holtum JAM, editors. Herbicide resistance in plants: biology and biochemistry. Boca Raton, FL: CRC Press; p. 215–228.
- Suzuki T, Usui I, Tomita-Yokotani K, Kono S, Tsubura H, Miki Y, Hasegawa K. 2001. Effects of acid extracts of tomato (Lycopersicon esculentum Mill.) and carrot (Daucus carota L.) wastes from the food industry on the growth of some crops and weeds. Weed Biol Manag. 1:226–230. doi: 10.1046/j.1445-6664.2001.00036.x
- Takikawa H. 2006. Synthetic studies on Breviones and structurally related natural products. Biosci Biotechnol Biochem. 70:1082–1088. doi: 10.1271/bbb.70.1082
- Tefera T. 2002. Allelopathic effects of Parthenium hysterophorus extracts on seed germination and seedling growth of Eragrostis tef. J Agron Crop Sci. 188:306–310. doi: 10.1046/j.1439-037X.2002.00564.x
- Thi HL, Lin C-H, Smeda RJ, Fritschi FB. 2014a. Isolation and purification of growth-inhibitors from Vietnamese rice cultivars. Weed Biol Manag. 14:221–231. doi: 10.1111/wbm.12050
- Thi HL, Lin C-H, Smeda RJ, Leigh ND, Wycoff WG, Fritschi FB. 2014b. Isolation and identification of an allelopathic phenylethylamine in rice. Phytochemistry. 108:109–121. doi: 10.1016/j.phytochem.2014.08.019
- Tregear JW, Jouannic S, Schwebel-Dugué N, Kreis M. 1996. An unusual protein kinase displaying characteristics of both the serine/threonine and tyrosine families is encoded by the Arabidopsis thaliana gene ATN1. Plant Sci. 117:107–119. doi: 10.1016/0168-9452(96)04400-7
- Vishnoi S, Agrawal V, Kasana VK. 2009. Synthesis and structure–activity relationships of substituted cinnamic acids and amide analogues: a new class of herbicides. J Agric Food Chem. 57:3261–3265. doi: 10.1021/jf8034385
- Vyvyan JR. 2002. Allelochemicals as leads for new herbicides and agrochemicals. Tetrahedron. 58:1631–1646. doi: 10.1016/S0040-4020(02)00052-2
- Wolf RB, Spencer GF, Kwolek WF. 1984. Inhibition of velvetleaf (Abutilon theophrasti) germination and growth by benzyl isothiocyanate, a natural toxicant. Weed Sci. 32:612–615.
- Wu Z, Zheng L, Li Y, Su F, Yue X, Tang W, Ma X, Nie J, Li H. 2012. Synthesis and structure–activity relationships and effects of phenylpropanoid amides of octopamine and dopamine on tyrosinase inhibition and antioxidation. Food Chem. 134:1128–1131. doi: 10.1016/j.foodchem.2012.02.152
- Yang GZ, Zhao S, Li YC. 2002. Studies on the chemical constituents of Lycianthes biflora. Yao Xue Xue Bao. 37:437–439.
- Yang Y, Song ZG, Liu ZQ. 2011. Synthesis and antioxidant capacities of hydroxyl derivatives of cinnamoylphenethylamine in protecting DNA and scavenging radicals. Free Radic Res. 45(4):445–453. doi: 10.3109/10715762.2010.540576