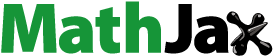
ABSTRACT
Effect of salt stress and subsequent re-watering on growth and electrophysiological characteristics of Orychophragmus violaceus and Brassica napus was investigated, to construct a model for prediction of an appropriate regime for dilution of saline water. Different concentrations of NaCl, Na2SO4 and blend of both salts were applied with Hoagland solution. Growth and electrophysiology traits were highly restricted at high concentration levels. According to the results, the best dilution point was 5–2.5% for NaCl and Na2SO4 alternatively, whereas it was 10–0.0% for blend of salts. After re-watering, O. violaceus restore better leaf tensity under medium levels, because the effect of NaCl concentration on leaf tensity (XNC–LT) was 1.66%, which was lower in O. violaceus than the effect of XNC–LT (2.88%) in B. napus, followed by the same trend for all treatments. Therefore, the effect of salinity in O. violaceus and B. napus may also be reduced effectively by dilution of saline irrigation.
Introduction
In abiotic stresses, drought and salinity are two environmental factors which are responsible for the huge loss in agricultural demand and productivity all over the world (Gul & Ahmad Citation2004). Water scarcities are expected to lead to loss of crop production globally up to 30% by 2025 compared to current yield. Salinity of agricultural soil is becoming a serious issue due to increasing shortage of fresh water and subsequent necessity to utilize saline water or low-quality water for irrigation (SEMİZ & Suarez Citation2015). Therefore, at the current situation of intentions of improving agricultural production under limited water resources, it is required to grow crops that are capable of tolerating drought and water stress environment (Yao et al. Citation2016). It would be the most economical approach to improve productivity based on limited water resources.
Orychophragmus violaceus and Brassica napus from Brassicaceae family are imperative oilseed crops, widely used for human food due to its improved oil content, high nutritious value, enrichment and stability in yield (Purty et al. Citation2008). Most species of brassica are considered to be moderate salt-tolerant (Masidur Alam et al. Citation2007). However, their growth and yield were markedly reduced due to stresses (Su et al. Citation2013) and also the production of edible oil crops was affected at a distressing rate due to rising issues of water and soil salinization (García-Sánchez et al. Citation2002). In order to provide attention to these aspects of these crops, studies were necessary according to variation in the morphological characteristics. Later on the experiment was conducted to emphasize on plants physiognomies under water and salt stress (Zhang et al. Citation2012; Benincasa et al. Citation2013; de Oliveira et al. Citation2013).
In the field, plants may have experienced several diverse abiotics, either contemporarily at different times throughout the growing season (Tester & Bacic Citation2005). Exposure of plants to a traumatic environment during development periods causes many physiological changes (Jamil et al. Citation2012). The most common adverse effect of salt stresses on the physiology is the reduction in plant growth and yield (Ashraf & McNeilly Citation2004; Abdul Qados Citation2011). Growth is the most important process inhibited, partially or completely by stresses. However, irrigation water quality may have a profound impact on plant growth including the series of processes. The first response of plants towards salinity stress is a reduction of leaf growth. If salt stress ultimately rises to toxic levels in the leaves, it initiates premature senescence and reduces leaf area to a level that cannot withstand growth characteristics (Munns Citation2002). Toxic effect of salt stress repressed leaf expansion (Roy et al. Citation2014) and reduces the production of new leaves (Mansour & Salama Citation2004). Salt tolerance in plants may be explained by functional and structural adaptations, such as growth regulation, water potential and osmotic adjustment. Osmotic adjustment is an adaptation mechanism for the plant to maintain their water stability (Zollinger et al. Citation2007).
Water stability and deficiency in plants can also be determined using their electrophysiological properties. In which, the water potential of a plant was reflected by water content, leaf air temperature and capacitance (Koide et al. Citation1989). Water potential describes the energy level of water movement (Vogt & Losch Citation1999). A significant relationship is observed between water content, water potential and physiological capacitance. Therefore, water status in leaves is found from the variations of physiological resistance and capacitance. The status of water in plants is also characterized by the tensity of the plant cell, which reflects the salts resistance of plants (Zhang et al. Citation2015). To overwhelm this situation, salt stress, subsequent re-watering or dilution of saline irrigation could be helpful for plants to recover their growth development through rebuilding of leaf tensity, maintain water potential and water content.
Prediction of re-watering or the dilution point of saline water is a new index, which could be helpful for the regulation of saline water, in order to sustain agricultural productivity and economical irrigation. An appropriate dilution of salt water on an appropriate point will save the water resource. O. violaceus and B. napus are hypothesized for better restorability after re-watering or dilution of salted water. Therefore, the intention of this present study was to observe the electrophysiological and growth responses of O. violaceus and B. napus under different salt stresses and subsequent re-watering conditions. Furthermore, to optimize plant growth and yield, a model needs to be constructed for predicting an appropriate regime for dilution of saline water on the basis of electrophysiological and growth responses.
Materials and methods
Experimental conditions
The experiment was carried out at the Institute of Agricultural Engineering, Jiangsu University, Zhenjiang, Jiangsu, China (32.20°N, 119.45°E). Intact seeds of O. violaceus and B. napus, which were identical in size and color, homogeneous and free from wrinkles, were chosen for this experiment. Seeds were cultivated in 20-cell trays, containing distilled water and washed vermiculite in equal amount. The seeds were left to grow inside the growth chamber under day/night temperature cycle of 25°C/20°C, and 60% of relative humidity.
Salt treatments
Plants were irrigated daily with Hoagland solution (Hoagland & Arnon Citation1950). After 20 days, plants were transferred to the greenhouse under natural lighting, (25/18) ± 2°C (day/night) and 70% relative humidity. Homogenous seedlings, showing healthy growth were selected for further treatments. They were exposed to salt stress, induced by NaCl, Na2SO4 and combination of both salts at different four levels, in which one is the control level. The concentration of NaCl (NC1:2.5, NC2:5.0, NC3:10.0 and 0 as control) g L−1; Na2SO4 (NS1:2.5, NS2:5.0, NS3:10.0 and 0 as control) g L−1 and in blend of salts (BS1: 2.5 g L−1 NaCl + 10.0 g L−1 Na2SO4; BS2: 10.0 g L−1 NaCl + 2.5 g L−1 Na2SO4; BS3: 5.0 g L−1 NaCl + 5.0 g L−1 Na2SO4 and 0 as control) were selected for treatments and applied to plants with Hoagland solution. The control plants only received a nutrient solution.
Re-watering orders
Re-watering was continued on day 14 from the onset of salt stress treatment. The order for re-watering was that, plants that were affected by high stress level (10.0 g L−1in both NaCl and Na2SO4) were irrigated with medium stress level NC3^2, NS3^2 (5.0 g L−1 in both NaCl and Na2SO4, respectively), afterwards medium stress level (5.0 g L−1in both NaCl and Na2SO4) irrigated with low stress level NC2^1, NS2^1 (2.5 g L−1 in both NaCl and Na2SO4, respectively) and then low stress level (2.5 g L−1 in both NaCl and Na2SO4, respectively) irrigated with controlNC1^0, NS1^0 (0 g L−1 in both NaCl and Na2SO4, respectively). In mixed treatments, all levels were re-watered with control (BS1^0, BS2^0, BS3^0). This experiment was designed in the randomized block design and a total of three replicates was chosen for each physiological measurements.
Determination of growth parameters
Growth measurements were considered after treatments and after re-watering three times per each week in both cases, respectively. The five replicates were chosen for each treatment and also used to analyze the mean of each measurement. The measurements taken for growth analysis were: plant height (PH); stem diameter (SD) and leaf area (LA). The leaf area was measured by a leaf area meter (a handheld laser leaf area meter, CI, 203).
Determination of leaf water potential and leaf water content
Leaves in salt stress phase and in subsequent re-watering phase were used for the determination of water potential (Ψ) and water content (WC). Leaves’ water potential were measured at 9:00–11:00 am with a dew point microvolt meter in a C-52-SF universal sample room (Psypro, Wescor, USA), every 3 days in both salt stress and re-watering phases, respectively. Five plants from each treatment group were selected for the measurement. The water content in leaves of O. violaceus and B. napus was measured through the relationship between the water potential (Ψ) of a plant tissue and cell SAP solute concentration (Q), and is(1)
(1) where Ψ is the water potential (MPa) of a plant tissue; I the dissociation coefficient (i = 1); Q the cell SAP solute concentration (mol L−1); R the gas constant (R = 0.0083 L MPa mol−1 K−1) and T the thermodynamic temperature (273 + t °C) K.
The relationship between proportion of solute quality in the total quality of leaf (P, %) and cell SAP solute concentration (Q) is(2)
(2) where M is the relative molecular mass of cell SAP solute, sugar C12H22O11, M was 342 g mol−1.
According to Equations (1) and (2), P could be rewritten as follows:(3)
(3) The proportion of water content in the total quality of leaf was 1 − P; it was expressed as WC (%)
(4)
(4)
Determination of leaf tensity
The leaf was clipped between two electrodes of the parallel plate capacitor, which formed a parallel plate-capacitor sensor. The water potential of the leaves changed with variations in cytosol solute concentration in the leaves. This behavior caused a change in the dielectric constant of the cytosol solute in the leaf tissue that was between the two electrodes. As a result, the physiological capacitance (CP) of the plant was affected.
The relationship between the proportion of solute quality in the total quality of the leaf (P) and the concentration (Q) was Q = 1000 P/M where M is the relative molecular mass of the cytosol solute. Comparing the relationship between P, Q, and CP, the values of leaf tensity (TL) is rewritten according to (Zhang et al. Citation2015) as shown below:(5)
(5) and
(6)
(6) For a given material, the relative dielectric constant (a = 3.3) and the molecular mass (M = 342) of the cytosol solute were all assigned values. The leaf tensity of the plants [TL = 1/y (i = 0, 1, 2, 3)] was determined to indicate the water status in the leaf. In this study, sugar C12H22O11 was identified as the solute in the cytosol; therefore, T is (K, T = 273 + t °C), R is the gas constant (R = 0.0083 L MPa mol−1 K−1) and i is the dissociation coefficient equal to 1.
Model construction for prediction of re-watering levels
The exponential decay equation is used to construct the model between salt stress levels and CP, also to construct the model between salt stress levels and TL, these are shown in Equations (5) and (6). Here, a1, a2, b1, b2 are constants and XEC is the salt concentrations in the form of a percentage (%).(7)
(7)
(8)
(8)
Predictions of re-watering levels were calculated using Equations (7) and (8). Substituting the values of CP and TL which were obtained through after re-watering into Equations (7) and (8) gave the point of predicted re-watering levels, equivalent to salt stress levels.
Statistical analysis
All measurements were subjected to analysis of variance (ANOVA) to discriminate significant differences (defined as p ≤ 0.05) between group means. Data are shown as the mean ± standard error (SE) (n = 5). These mean data were analyzed statistically using a factorial design through SPSS software (version 13.0, SPSS Inc.), and mean results were compared through LSD at 5% significance level (p < 0.05).
Results
Effect of salt stress and re-watering on plant growth
The plant height (PH), stem diameter (SD) and leaf area (LA) of both species showed a noticeable decrease on being exposed to different concentrations of both salts (–). Of the different concentration of both salts, the lowest concentration (NC1 and NS1) showed a minimum reduction compared with the control. But, the highest concentration of both single salts and blend of both salts at NC3, NS3, BS1, and BS2 made severe reduction in PH, SD and LA, respectively.
Table 1. Effect of salt stress and re-watering on plant height of O. violaceus and B. napus.
Table 2. Effect of salt stress and re-watering on stem diameter of O. violaceus and B. napus.
Table 3. Effect of salt stress and re-watering on leaf area of O. violaceus and B. napus.
During treatment periods of O. violaceus at the BS1concententration level, it triggered a maximum decrease in PH (5.70 cm), SD (0.04 cm) and LA (11.35 cm2), compared with B. napus. However, the high concentration of blend of salts caused a more prominent decrease in the growth parameters of O. violaceus than B. napus as compared to single salts. Moreover, single Na2SO4 was found to be toxic to O. violaceus and B. napusat NS3 than NC3, high concentration level of NaCl, respectively (–).
A general trend appeared in the increments of plant growth in both species during re-watering periods. A statistical analysis specified that the increments which were observed during re-watering was significant, except for the concentration at high levels (NC3^2, NS3^2, BS1^0 and BS2^0). The increments in PH, SD and LA were obtained more under the medium concentration of single and blend of salts (NC2^1, NS2^1 and BS3^0) followed by the low concentration of salt (NC1^0 and NS1^0) as compared to control, respectively. But relatively, the increments of PH (58.27%, 56.20% and 55.95%), SD (62.16%, 60% and 60%) and LA (46.53%, 44.91% and 43.78%) were observed more in O. violaceus under NC2^1, NS2^1 and BS3^0, medium concentration of single and blend of salts, compared with the responses of B. napus under medium concentration of single and blend of salts, respectively. However, at a high concentration of single and blend of salts (NC3^2, NS3^2, BS1^0 and BS2^0), the adverse effect of salt stress on PH, SD and LA, was exhibited. Although, the addition of salts at BS3^0 revealed the same effect on PH, SD and LA as compared to PH, SD and LA under NC2^1 and NS2^1 concentration levels, respectively (–). In general, statistical analysis demonstrated that after re-watering O. violaceus showed better growth development than B. napus, respectively.
Water content and water potential
Results shown in and indicated that an increase in the concentration of salts decreased the water potential (Ψ) and water content (WC) of leaves in both species during treatments periods. The maximum loss in WC was observed in both species at high concentration levels of both single and blend of salts (NC3, NS3, BS1 and BS2) as compared to control. While the minimum decrease in both WC and Ψ was noted at under low concentration levels (NC1 and NS1) as compared with the control in both O. violaceus and B. napus, respectively. However, the maximum decrease in Ψ was noted at NC3, NS3, BS1 and BS2, high concentration levels, respectively. While in medium (NC2, NS2 and BS3) concentration of NaCl, Na2SO4 and blend of salts, the decrease in WC and in Ψ was found to be almost the same in both O. violaceus and B. napus, respectively. As a comparison between single salts and a mixture of salt, it was observed that WC and Ψ of B. napus were significantly less affected by NaCl concentrations than Na2SO4 and blend of salt concentrations. However, the high concentration blend of salts affects O. violaceus compared with single salts and caused a more prominent decrease in the WC and Ψ compared with B. napus.
Table 4. Effect of salt stress and salt stress subsequently re-watering on water potential of O. violaceus and B. napus.
Table 5. Effect of salt stress and salt stress subsequently re-watering on water content of O. violaceus and B. napus.
In the re-watering phase, the outcome of the results showed that WC and Ψ of both O. violaceus and B. napus were recovered ( and ). The increment in WC and Ψ increased under low (NC1^0 and NS1^0) to medium concentration levels (NC2^1, NS2^1 and BS3^0). But relatively, the increments of WC (10.41%, 12.50% and 12.40%) were observed more in O. violaceus under NC2^1, NS2^1 and BS3^0, medium concentration of single and blend of salts, compared with the results of B. napus (4.79%, 7.38% and 9.22%) under medium concentration of single and blend of salts, respectively.
But the degree of salts still showed adverse effects at high concentration levels (NC3^2, NS3^2, BS1^0 and BS2^0) with an increment of WC and Ψ even during the re-watering phase. Consequently, the blend of salts at BS3^0 revealed the same effect on WC and Ψ as compared with WC and Ψ under NC2^1 and NS2^1 concentration levels, respectively. Overall, the statistical analysis revealed that O. violaceus showed better restorability of WC and Ψ after re-watering than B. napus, respectively.
Physiological capacitance and leaf tensity
From the study, it was found that the physiological capacitance (CP) and leaf tensity (TL) significantly decreased with increasing salt stress in comparison with the control ( and ) in both species. O. violaceus showed better tolerance than B. napus from low (NC1 and NS1) to medium (NC2, NS2 and BS3) concentration of NaCl, Na2SO4 and blend of salts. Maximum reduction of CP and TL was noted in both O. violaceus and B. napus under high concentrations at NC3, NS3, BS1 and BS2 levels of NaCl, Na2SO4 and blend of salts, respectively, as compared with control. While the minimum reduction of CP and TL was recorded in both O. violaceus and B. napus under low (NC1 and NS1) concentrations of NaCl and Na2SO4 as compared with CP and TL under control condition. O. violaceus exhibited the highest reduction in CP and TL under BS1concentration of a blend of salts as compared with B. napus. However, in medium (NC2, NS2 and BS3) concentration of NaCl, Na2SO4 and mixture of salts, the reduction in CP and TL was found to be almost the same.
Table 6. Effect of salt stress and salt stress subsequently re-watering on physiological capacitance of O. violaceus and B. napus.
Table 7. Effect of salt stress and salt stress subsequently re-watering on leaf tensity of O. violaceus and B. napus.
The physiological capacitance (CP) and leaf tensity (TL) significantly increased during salt stress followed by re-watering. It was observed that O. violaceus exhibited better results compared with B. napus from the stress phase to the re-watering phase. During re-watering, CP and TL increased under treatments with low concentration levels of NC1^0 and NS1^0, respectively. However, salt stress in both species at high concentration affected CP and TL adversely following the order NC3^2, NS3^2, BS1^0 and BS2^0, respectively. While in the re-watering phase, the increment in TL under low salt stress treatments (NC1^0 and NS1^0) was found to be the highest at 0.106 and 0.102 cm in O. violaceus, 0.113 and 0.113 cm in B. napus, respectively. Relatively, more increments of CP and TL were found in O. violaceus under NC2^1, NS2^1 and BS3^0, medium concentration levels, respectively. However, O. violaceus showed better recovery of CP and TL from low to medium concentration levels than B. napus ( and ).
Model construction relationship between leaf area and physiological capacitance
By using data of CP and TL under salt stress levels, a model using exponential decay equations can be given as shown in .
Table 8. Model between salt concentrations and physiological capacitance and leaf tensity.
It is noted that the relationship between salt stress concentrations (XC) and CP or leaf tensity and between XC and TL can characterize well the relationship between leaf water status and salt stress concentrations. The coefficient of determination (R2) showed that the relationship between CP and XC in O. violaceus fits better with the exponential decay equation as compared with the relationship between TL and XC. While in B. napus, R2 showed that the relationship between CP and XC is the same with the exponential decay equation as compared with the relationship between TL and XC.
Re-watering or dilute irrigation points were almost the same by using the CP and TL equation for both species. The values of dilute irrigation of both cultivars are shown in .
Table 9. Equivalent salt stress levels of both species based on physiological capacitance and leaf tensity.
But after dilution, B. napus showed sensitive behavior as compared to O. violaceus. According to CP and TL model equations, it is cleared that B. napus and O. violaceus have to re-water or dilution of salted water when XC was reached to 5%. When the plant was exposed to 10% of XC in the case of single NaCl (XNC) and Na2SO4 (XNS) and 12.5% in the case of the blend of salts (XMNC and XMNS) more reduction in plant growth was found (–). Consequently, the effect of dilution on CP and TL under 5–2.5% concentration was 1.66% (XNC–LT), 1.74% (XNC–CP), 1.45% (XNS–LT), 1.28% (XNS–CP), 1.60% (XMNC–LT), 1.94% (XMNC–CP), 1.45% (XMNS–LT) and 1.77% (XMNC–CP), respectively, in O. violaceus and 2.88% (XNC–LT), 2.98% (XNC–CP), 2.92% (XNS–LT), 3.01% (XNS–CP), 3.15% (XMNC–LT), 2.95% (XMNC–CP), 2.97% (XMNS–LT) and 2.81% (XMNC–CP), respectively, in B. napus. Therefore, recovery was more in O. violaceus under 5–2.5%, because of dilution effects, observed on plants was 1.66% of XNC–LT but in B. napus the effect was observed 2.88% of XNC–LT followed by the same trend for all the concentrations. Hence, this regime is considered the best for dilution of salted water for both the species. Therefore, the dilution point is the best condition to start re-watering or dilution of saline water for plant growth development because beyond that point plants will be suffer serious stress.
Discussion
Effect of salt stress on electrophysiological properties
O. violaceus and B. napus have varied inconsistency in their response to salt stress. The responses of the plants to salt stresses in terms of growth development are definitive expressions of many physiological processes. A surprising concentration of salts affected the capability of roots to extract water, resulting in the limitation of many physiological processes such as hindrance in nutrients uptake (Munns & Tester Citation2008) and reduction in the plant growth and development (Zapryanova & Atanassova Citation2009; Wang et al. Citation2011). Stress caused excessive reduction in PH, SD and LA at high stress levels (NC3, NS3, BS1 and BS2), due to leaf tissue dehydration and limiting photosynthesis activity through stomatal closure (–). At that point, there was a negative relationship between plant growth and salts’ concentration, in which the salts’ accumulation in leaves directly affect Ψ (Niu et al. Citation2008; Fu et al. Citation2010) by decreasing the water content of the leaves. However, the lowest Ψ values were recorded in plants when subjected to water stress (Álvarez et al. Citation2012).
Salt stress at high concentration levels in both species exerts its effect directly on CP by disturbing the water status of leaves, which was in response to the effect on leaf cell extension. The CP of O. violaceus was decreased and affected more as compared to B. napus at high concentration levels of single and blend of salts and Ψ also decreased, which indicates that water absorption ratios were disturbed due to leaf cell shrinkage. The decrease in leaf area was a response to the compact expansion of the leaf cell. However, minimum reduction in CP of O. violaceus and B. napus from low (NC2 and NS2) to medium concentration levels (NC2, NS and BS3) indicates that the water-holding capability of the leaf cell might have played a vital role to maintain leaf WC. However, the decrease and increase in the physiological capacitance of the leaves indicate the concentration of solute in the cytosol. The decrease in the concentration of solute in the cytosol describes the threshold cell’s value for dysfunction. Meanwhile the increase and decrease in Ψ indicate the plant’s physiological method for survival when the loss of water is continued (Zhang et al. Citation2015). Meinzer et al. (Citation2003) use the values of CP to measure water transport properties in trees of sapwood. As a whole, there is need to determine Ψ and CP together at the same time because both reflect the leaf water status of plants. The synergetic effect of Ψ and CP allows the plants to survive under water stress environment.
Leaves’ cell tension has the ability to sustain water in order to maintain development of plants. Leaf cell tensity is used to describe the plant’s water status. The variations in tension reflect the amount of water lost and the ability of the leaf cell to hold water (Irigoyen et al. Citation1992). The capability of holding water is directly linked with the drought tolerance of plants under stress conditions. The results in show that the minimum reduction found from low–medium stress levels in TL of B. napus indicates the minimum change in TL and the ability of B. napus to hold water. While the changes in TL of O. violaceus were also found to be minimum under low–medium stress levels, but water-holding capability of O. violaceus was not better as found in B. napus. However at high stress levels, TL of O. violaceus and B. napus reduced sharply and their ability to hold water was not good. Thus, this condition exposed the threshold flexibility and tolerance of O. violaceus and B. napus under low–moderate levels because of the mechanisms involved in osmotic tolerance related to water availability to sustain leaf cell’s energy demands for growth.
Re-watering or dilution point
Predicted re-watering salt stress levels based on CP and TL showed that O. violaceus and B. napus have to re-water when the plant goes under medium concentration levels (). In the absence of salt stress, predicted values for both species were near to zero, indicating that the present finding is credible. For O. violaceus, in addition to the control (no salt stress), the medium stress levels of NaCl and Na2SO4 from 5.0% rehydration to 2.5% treatment and from 10% rehydration to 0.0% treatment in blend of salts indicate that after dilution, adaptability of plants is greatly enhanced. Later, adaptability of B. napus is greatly enhanced, but not as observed in the case of O. violaceus. The results of growth parameters of both species were also found to be better in predicting these re-watering levels (–), indicating that these predicting re-watering levels techniques are very credible. Best predicting re-watering levels and dilution points of both species were also found in the same regime that also strengthens our findings. Because beyond that point up to high stress levels of single NaCl and Na2SO4 and blend of salts, growth was inhibited. When the plant was exposed to high salinity levels, 50% reduction is found in plant growth (Siringam et al. Citation2011, Aref Citation2013). Our results for O. violaceus and B. napus also agreed with their finding, because both species showed sudden reduction in plant growth at high stress levels (NC3, NS3, BS1 and BS2), and there was no recovery found when XNC and XNS were diluted to 10–5% and XMNC and XMNS were diluted from 12.5% to 0.0%, respectively (). Brodribb and Holbrook (Citation2005) explained that at 50% collapse of cells, the plant could not recover well during re-watering. Therefore, because of this reason 30% yield loss can be considered acceptable for re-watering in this study. According to 70% increment in plant growth during salt stress, medium concentration levels (NC2, NS2 and BS3) of NaCl, Na2SO4 and blend of salts is considered the best for the production of B. napus under salt-stressed conditions. But in the case of O. violaceus, it exposed the sensitive behavior but after re-watering or dilution, it showed better restoration. It might be that after re-watering of B. napus, CP and TL under medium to high concentration levels did not increase much and solute concentration in the cytosol could have reached below the threshold value due to continuous stress which destroyed the leaf cells (Zhang et al. Citation2015).
From the results (), it is clear that the dilution point (5–2.5%) under NaCl concentrations was the same for both species which were 1.66% (XNC–LT) and 2.88% (XNC–LT) for O. Violaceus and B. napus, respectively. Because at 10% salt concentration, salt stress was too severe to be re-watered, and the irrigation effects using diluted salted water (2.5%) at 5% salt stress level in O. violaceus and B. napus were equivalent to the effects using 1.66% salted water and 2.88% salted water, which were equal to the salt stress level between 0% and 2.5%. Accordingly, when the concentration of salted water was 2.5% in both species, it could be used for irrigation directly, but when the concentration of salted water was 5% in both species, we need to dilute it to 2.5%, and then be used for irrigation, the same trend needs to be followed by Na2SO4 concentrations (XNS–LT) and blend of salts (XMNC–LT) and (XMNS–LT), respectively. Therefore, irrigation effects using diluted salted water equivalent to salt stress levels could be predicted through electrophysiological properties.
Restoration of O. violaceus and B. napus through re-watering
Re-watering had a better effect on O. violaceus and B. napus and plants successfully recovered. It showed the tolerance and maintenance of plants up to some threshold levels. A substantial increase occurred even during re-watering of O. violaceus in PH, SD and LA from low (NC1^0 and NS1^0) to moderate levels (NC2^1, NS2^1 and BS3^0) (–). The increments of PH, SD and LA were found more after re-watering in O. violaceus rather than B. napus, due to the increase in CP and recovery of Ψ. It indicates that the water-holding capability of the leaf cell of O. violaceus was better to maintain leaf WC. A little contribution was found in this research direction by Flexas et al. (Citation2009), focusing on photosynthetic acclimation followed by re-watering to induce recovery of the plant. In the previous study, O. violaceus was found to be shade-tolerant as compared to other Brassicaceae species because its photosynthetic activity was increased by regulation of higher CA activity (Wu et al. Citation2011). Though, O. violaceus exhibited better ability to resist salt stress than B. napus after re-watering.
Salinity affects the leaf characteristic of the plant by inhibiting leaf expansion and elongation (Marcelis & Van Hooijdonk Citation1999; Qiu et al. Citation2007). A considerable reduction was observed under high stress levels even during re-watering in growth development of O. violaceus and B. napus because of no recovery in CP and unstable status of Ψ ( and ). It also specifies that the ability of the plant to hold water by building leaf cell was not good to maintain water status of leaves. Later TL of O. violaceus and B. napus was sustained and recovered at medium stress levels of both single and blend of salts as compared with high stress levels. It is because of restorability of TL, which can be used to illustrate the water status of the plant and the capacity of holding water. The results in show that the maximum increase found under medium stress levels in TL of O. violaceus indicates the maximum recovery of TL and the ability of O. violaceus to hold water. While at the same time recovery of leaf tensity in B. napus was also found to be maximum under low–medium stress levels, but the water-holding capability of B. napus was not better as was found in O. violaceus after re-watering. Though, under moderate stress, TL directly reflected the ability to resist stress in terms of water loss, whereas salt stress subsequent re-watering reflected the ability to resist stress after dehydration. Thus, this condition exposed the threshold flexibility and more tolerance of O. violaceus under moderate levels after re-watering, because of the mechanisms involved in osmotic tolerance related to water availability to sustain leaf cell’s energy demands for growth.
Even in the re-watering phase, TL was still damaged at high concentration levels. This indicates the unhealthy status of plants due to limited water uptake movements, and poor water-holding ability. Plants tolerance to salt stress differs with the degree at which salt reached toxic levels in leaves because of the Ψ difference between the atmosphere and leaf cells (Carillo et al. Citation2011). It was hard for both O. violaceus and B. napus to be recovered from the excessive salt stresses. So, our results agreed with Brodribb and Holbrook (Citation2005), who described that at 50% collapse of cells, it is difficult for a plant to recover after re-watering. Consequently, O. violaceus and B. napus were thought to be the species with single and blend of salts tolerance flexibility under moderate stress conditions. So, re-watering of saline water should be done under moderate levels. The moderate stress level of both single and blended salts was considered the best for threshold tolerance and production of O. violaceus and B. napus under saline condition. The effect of salinity in both O. violaceus and B. napus was reduced. However, in both O. violaceus and B. napus negligible reduction was observed, which might be attributed to the dilution of saline irrigation (re-watering) and by the combination of salts. Furthermore, the toxic effect of blended salts at BS3^0 was found to be the same as it is noted under NC2^1 and NS2^1 concentration levels of single salts. Hence, B. napus can be grown under moderate soil salinity because of its salt tolerance adaptability nature and O. violaceus can be grown where saline water resources are existing because of its restoration after re-watering under moderate conditions.
Conclusion
It is concluded that salinity affects the growth of plants by disturbing electrophysiological characteristics of O. violaceus and B. napus, but the response was different at different salt levels. The maximum recovery of Cp and TL at medium stress levels after re-watering shows better water-holding capability and growth restorability of O. violaceus than B. napus. Because at 10% salt concentration, salt stress was too severe to be re-watered, and the irrigation effects using diluted salted water (2.5%) at 5% salt stress level in O. violaceus and B. napus were equivalent to the effects using 1.66% salted water and 2.88% salted water, respectively, which were equal to the salt stress level between 0% and 2.5%. Accordingly, when the concentration of salted water was 2.5% for both species, it could be used for irrigation directly, but when the concentration of salted water was 5% in both species, we need to dilute it to 2.5%, and then use it for irrigation, the same trend needs to be followed for Na2SO4 concentrations (XNS–LT) and blend of salts (XMNC–LT) and (XMNS–LT), respectively. However, from the results (), it is clear that the dilution point exists in NC2, NS2 and BS3, 5–2.5% for single NaCl and Na2SO4 and 10–0.0% for blend of salts. Hence, prediction of dilution point of saline irrigation based on electrophysiological properties could be helpful to maintain crop productivity, reduce irrigation cost, save water resources and could be a better approach to utilize existing water resources.
Disclosure statement
No potential conflict of interest was reported by the authors.
Additional information
Funding
References
- Abdul Qados AMS. 2011. Effect of salt stress on plant growth and metabolism of bean plant Vicia faba (L.). JSSAS. 10:7–15.
- Álvarez S, Gómez-Bellot MJ, Castillo M, Bañón S, Sánchez-Blanco MJ. 2012. Osmotic and saline effect on growth, water relations, and ion uptake and translocation in Phlomis purpurea plants. Environ Exp Bot. 78:138–145. doi: 10.1016/j.envexpbot.2011.12.035
- Aref F. 2013. Effect of saline irrigation water on yield and yield components of rice (Oryza sativa L.). Afr J Biotechnol. 12:3503–3513.
- Ashraf M, McNeilly T. 2004. Salinity tolerance in brassica oilseeds. Crit Rev Plant Sci. 23:157–174. doi: 10.1080/07352680490433286
- Benincasa P, Pace R, Quinet M, Lutts S. 2013. Effect of salinity and priming on seedling growth in rapeseed (Brassica napus var oleifera Del.). Acta Sci Agron. 35:479–486. doi: 10.4025/actasciagron.v35i4.17655
- Brodribb TJ, Holbrook NM. 2005. Water stress deforms tracheids peripheral to the leaf vein of a tropical conifer. Plant Physiol. 137:1139–1146. doi: 10.1104/pp.104.058156
- Carillo P, Annunziata MG, Pontecorvo G, Fuggi A, Woodrow P. 2011. Salinity stress and salt tolerance. In: A. Shanker, editor. Abiotic stress plants-mech adapt. Rijeka, Croatia: In Tech; p. 21–38.
- de Oliveira AB, Gomes-Filho E, Alencar NLM, Akinci S. 2013. Comparison between the water and salt stress effects on plant growth and development. In: A. Sener, editor. Response of organisms to water stress. Rijeka, Croatia: InTech; p. 67–94.
- Flexas J, Barón M, Bota J, Ducruet J-M, Gallé A, Galmés J, Jiménez M, Pou A, Ribas-Carbó M, Sajnani C, et al. 2009. Photosynthesis limitations during water stress acclimation and recovery in the drought-adapted Vitis hybrid Richter-110 (V. berlandieri × V. rupestris). J Exp Bot. 60:2361–2377. doi: 10.1093/jxb/erp069
- Fu A, Chen Y, Li W. 2010. Analysis on the change of water potential of Populus euphratica Oliv. and P. Russkii Jabl under different irrigation volumes in temperate desert zone. Chin Sci Bull. 55:965–972. doi: 10.1007/s11434-009-0459-x
- García-Sánchez F, Jifon JL, Carvajal M, Syvertsen JP. 2002. Gas exchange, chlorophyll and nutrient contents in relation to Na+ and Cl− accumulation in ‘sunburst’ mandarin grafted on different rootstocks. Plant Sci. 162:705–712. doi: 10.1016/S0168-9452(02)00010-9
- Gul H, Ahmad R. 2004. Effect of different irrigation intervals on growth of canola (Brassica napus L.) under different salinity levels. Pak J Bot. 36:359–372.
- Hoagland DR, Arnon DI. 1950. The water-culture method for growing plants without soil. Circ Calif Agric Exp Sta. 347:1–32.
- Irigoyen J, Einerich D, Sánchez-Díaz M. 1992. Water stress induced changes in concentrations of proline and total soluble sugars in nodulated alfalfa (Medicago sativd) plants. Physiol Plant. 84:55–60. doi: 10.1111/j.1399-3054.1992.tb08764.x
- Jamil M, Bashir S, Anwar S, Bibi S, Bangash A, Ullah F, Rha ES. 2012. Effect of salinity on physiological and biochemical characteristics of different varieties of rice. Pak J Bot. 44:7–13.
- Koide RT, Robichaux RH, Morse SR, Smith CM. 1989. Plant water status, hydraulic resistance and capacitance. In: Plant physiological ecology: field methods and instrumentation. Dordrecht: Springer; p. 161–183.
- Mansour MMF, Salama KHA. 2004. Cellular basis of salinity tolerance in plants. Environ Exp Bot. 52:113–122. doi: 10.1016/j.envexpbot.2004.01.009
- Marcelis L, Van Hooijdonk J. 1999. Effect of salinity on growth, water use and nutrient use in radish (Raphanus sativus L.). Plant Soil. 215:57–64. doi: 10.1023/A:1004742713538
- Masidur Alam M, Hayat S, Ali B, Ahmad A. 2007. Effect of 28-homobrassinolide treatment on nickel toxicity in Brassica juncea. Photosynthetica. 45:139–142. doi: 10.1007/s11099-007-0022-4
- Meinzer F, James S, Goldstein G, Woodruff D. 2003. Whole-tree water transport scales with sapwood capacitance in tropical forest canopy trees. Plant Cell Environ. 26:1147–1155. doi: 10.1046/j.1365-3040.2003.01039.x
- Munns R. 2002. Comparative physiology of salt and water stress. Plant Cell Environ. 25:239–250. doi: 10.1046/j.0016-8025.2001.00808.x
- Munns R, Tester M. 2008. Mechanisms of salinity tolerance. Annu Rev Plant Biol. 59:651–681. doi: 10.1146/annurev.arplant.59.032607.092911
- Niu G, Rodriguez DS, Aguiniga L. 2008. Effect of saline water irrigation on growth and physiological responses of three rose rootstocks. HortScience. 43:1479–1483.
- Purty RS, Kumar G, Singla-Pareek SL, Pareek A. 2008. Towards salinity tolerance in Brassica: an overview. Physiol Mol Biol Plants. 14:39–49. doi: 10.1007/s12298-008-0004-4
- Qiu D, Lin P, Guo S. 2007. Effects of salinity on leaf characteristics and CO2/H2O exchange of Kandelia candel (L.) Druce seedlings. J For Sci. 53:13–19.
- Roy SJ, Negrão S, Tester M. 2014. Salt resistant crop plants. Curr Opin Biotech. 26:115–124. doi: 10.1016/j.copbio.2013.12.004
- SEMİZ GD, Suarez DL. 2015. Tomato salt tolerance: impact of grafting and water compositionon yield and ion relations. Turk J Agric For. 39:876–886. doi: 10.3906/tar-1412-106
- Siringam K, Juntawong N, Cha-um S, Kirdmanee C. 2011. Salt stress induced ion accumulation, ion homeostasis, membrane injury and sugar contents in salt-sensitive rice (Oryza sativa L. spp. indica) roots under isoosmotic conditions. Afr J Biotechnol. 10:1340–1346.
- Su J, Wu S, Xu Z, Qiu S, Luo T, Yang Y, Chen Q, Xia Y, Zou S, Huang B-L. 2013. Comparison of salt tolerance in brassicas and some related species. AJPS. 4:1911–1917. doi: 10.4236/ajps.2013.410234
- Tester M, Bacic A. 2005. Abiotic stress tolerance in grasses. From model plants to crop plants. Plant Physiol. 137:791–793. doi: 10.1104/pp.104.900138
- Vogt UK, Losch R. 1999. Stem water potential and leaf conductance: A comparison of Sorbus aucuparia and Sambucus nigra. Phys Chem Earth Pt B: Hydrology, Oceans and Atmosphere. 24:121–123. doi: 10.1016/S1464-1909(98)00022-7
- Wang X, Geng S, Ri Y-J, Cao D, Liu J, Shi D, Yang C. 2011. Physiological responses and adaptive strategies of tomato plants to salt and alkali stresses. Sci Hortic-Amsterdam. 130:248–255. doi: 10.1016/j.scienta.2011.07.006
- Wu Y, Shi Q, Wang K, Li P, Xing D, Zhu Y, Song Y. 2011. An electrochemical approach coupled with Sb microelectrode to determine the activities of carbonic anhydrase in the plant leaves. In: D. Zheng, editor. Future intelligent information systems. Berlin: Springer-Verlag; p. 87–94.
- Yao X, Yang R, Zhao F, Wang S, Li C, Zhao W. 2016. An analysis of physiological index of differences in drought tolerance of tomato rootstock seedlings. J Plant Biol. 59:311–321. doi: 10.1007/s12374-016-0071-y
- Zapryanova N, Atanassova B. 2009. Effects of salt stress on growth and flowering of ornamental annual species. Biotechnol Biotech Equip. 23:177–179. doi: 10.1080/13102818.2009.10818394
- Zhang M, Wu Y, Xing D, Zhao K, Yu R. 2015. Rapid measurement of drought resistance in plants based on electrophysiological properties. ASABE. 58:1441–1446. doi: 10.13031/trans.58.11022
- Zhang X, Wang Z, Zhang X, Li M, Zou J. 2012. Effects of heavy metals and saline-alkali on growth, physiology and biochemistry of Orychophragmus violaceus. Agric Sci Technol (农业科学与技术). 13:1478–1483.
- Zollinger N, Koenig R, Cerny-Koenig T, Kjelgren R. 2007. Relative salinity tolerance of intermountain western United States native herbaceous perennials. HortScience. 42:529–534.