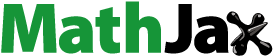
ABSTRACT
This study was aimed to elucidate the mitigation mechanism of an endophytic bacterium, Bacillus subtilis (BERA 71) against Macrophomina phaseolina (Tassi) Goid disease in mung bean. M. phaseolina reduced the plant growth by inducing disease, hydrogen peroxide (H2O2) and lipid peroxidation. The inoculation of B. subtilis to diseased plants increased chlorophyll, ascorbic acids, and superoxide dismutase, catalase, peroxidase, ascorbate peroxidase and glutathione reductase activities, and while inhibited H2O2 and lipid peroxidation for enhancing plant growth. In addition, B. subtilis association in plants mitigated the M. phaseolina infection due to increase of indole acetic acids and indole butyric acid, and also a decrease of abscisic acid. However, the nutrients (N, K, Ca, Mg, Zn, Cu, Mn and Fe) were increased, except Na in M. phaseolina diseased plants treated with B. subtilis. The result of this study suggests that B. subtilis interaction with plants can modulate the metabolism of pigments, hormones, antioxidants and nutrients against M. phaseolina to induce disease resistance in mung bean.
Introduction
Mung bean (Vigna radiata L.Wilczek) is an important food legume crop, and serves as a source of protein diet to human and animal population (Taylor et al. Citation2005; Yaqub et al. Citation2010). Macrophomina phaseolina (Tassi) Goid is one of the virulents and destructive pathogens (Sandhu and Singh Citation1998) and causes charcoal root-rot disease and has been reported as the most devastating disease of mung bean cultivation. The pathogen attacks on all parts of the plant, including roots, stems, branches, petioles, leaves, pods and seeds. Moreover, the infection of M. phaseolina on seeds (2.2–15.7%) causes the reduction of 10.8% in grain yield and 12.3% in protein content of mung bean (Kaushik et al. Citation1987). Soil- and seed-borne nature of the disease-causing pathogens pose a major problem for an effective disease management.
Pathogen-induced biotic stress affects the plant growth by their negative influence on the developmental patterns of roots show a reduction of root growth and uptake of water and mineral (Lee et al. Citation2016). Different tolerance mechanisms are involved in averting the negative effects of these stresses, including up and down-regulation of physiologically and biochemically important traits such as osmotic regulation, antioxidants and ion exclusion (Oliva et al. Citation2009). Pathogens attack plants and generates toxic reactive oxygen species (ROS), leading to a significant damage to cells by initiating the chain of damaging reactions (Vellosillo et al. Citation2010). Though, ROS plays an important role in plant growth regulation, their increased synthesis hampers the normal growth (Mittler Citation2002). Certain indigenous mechanisms are employed to detoxify free radicals for protecting cellular machinery and maintaining the plant growth (Egamberdieva et al. Citation2014). Antioxidant enzymes – including superoxide dismutase (SOD), ascorbate peroxidase (APX), catalase (CAT) and glutathione reductase (GR) – and non-enzymatic components composed of glutathione and ascorbic acid (AsA) are found to involve in scavenging of the ROS. The potential recovery of plants from oxidative damage is dependent on the detoxification of ROS for protecting vital cellular functions (Dong et al. Citation2012).
Although the application of agrochemicals controls the growth of pathogen and diseases in plants, their accumulation in soil and water causes detrimental effects on plants and other living organisms, and also risk for sustainable agriculture (Correa et al. Citation2009). An alternative, environmental-friendly bio-control method is needed for disease control. Microbes in soil or plants help prevent the disease damages in plants. Specifically, plant-growth-promoting rhizobacteria (PGPR) are the important group of microbes able to colonize plant roots (Lugtenberg and Kamilova Citation2009). Among PGPR, Bacillus subtilis is a nonpathogenic bacterium that lives in soil, often in association with roots of higher plants. B. subtilis are capable of forming dormant spores that are resistant to extreme conditions and thus can be easily formulated and stored (Piggot and Hilbert Citation2004). It also produces a variety of biologically active compounds for improving plant growth and a broad spectrum of antimicrobial compounds against phytopathogens to induce host systemic resistance (Nagorska et al. Citation2007; Ongena and Jacques Citation2008). Several strains of B. subtilis have been reported to be capable of forming multicellular structures or biofilms in rhizosphere and roots to enhance the plant growth (Bais et al. Citation2004). The commercial B. subtilis fertilizers (Kodiak, Quantum-400 and Serenade) are successfully employed in the agricultural field to enhance the crop yield (Brannen and Kenney Citation1997; Ngugi et al. Citation2005; Cawoy et al. Citation2011). The bio-control aspects of B. subtilis are studied against few plant pathogens, but there is a lack of understanding the mitigation strategy of B. subtilis in crop plants infected with M. phaseolina. Therefore, this study was aimed to elucidate the impact of B. subtilis on growth and biochemical regulation of mung bean infected with M. phaseolina. Moreover, the mitigation mechanism of B. subtilis against M. phaseolina was determined by analysis of pigments, hormones, antioxidants and nutrients in mung bean.
Material and methods
Experimental seeds and soil
The seeds of mung bean (V. radiata L. Wilczek) cultivar Kawmy-1 were procured from the Legume Research Department, Field Crop Institute, Agricultural Research Center, Giza, Egypt. The soil used in the experiment was clay soil (silt, 34.2%; clay, 55%; pH 7.5; organic matter, 2.3%; calcium carbonate, 2.01%; EC, 1.3 mmhos/cm2; nitrogen, 42.07 ppm; potassium, 227 ppm; phosphorus, 20.11 ppm). The soil mixed with acid washed pure sand as 2:1 (w/w) and autoclaved at 137.9 kPa for 20 min.
Endophytic bacterium and its formulation
B. subtilis, BERA 71 was isolated from roots of Talh trees (Acacia gerrardii) as described in our previous study (Hashem et al. Citation2016). The 16S rDNA sequence of B. subtilis BERA 71 was submitted to the Gen Bank nucleotide sequence database and the accession number was given as KX090253. The formulation of B. subtilis was done by following the method of Hashem et al. (Citation2013). The Erlenmeyer flasks (250 mL) containing 100 mL of MCF (magnesium sulfate [0.25 g], dipotassium hydrogen phosphate [2.5 g], yeast extract [13.8 g], sodium chloride [2.5 g], manganese sulfate [0.1 g], anhydrous potassium phosphate monobasic [1 g] and sucrose [6.5 g]) liquid medium (Freitas et al. Citation2015) were inoculated with 106 CFU (colony forming unit)/mL (1.0 mL/flask) and incubated at 25 ± 1°C for 48 h under continuous shaking at 150 rpm. After incubation, the bacterial samples were lyophilized under vacuum and the lyophilized powder was mixed with talc powder and 1.0% carboxy methyl cellulose (w/w) as an adhesive agent to give a final concentration of 3.6 × 109 CFU of B. subtilis/g of formulated material (Hashem et al. Citation2013).
Isolation and identification of phytopathogen strain
M. phaseolina (Tassi) Goid was isolated from diseased root samples of mung bean from Hehia, Zagazig, Sharkia governorate, Egypt. The root samples were washed thrice in sterilized double distilled water to remove adhering soil particles. The collected roots were cut into small pieces (0.5 cm) and surface sterilized with 2% sodium hypochlorite (v/v) for 2 min. After surface sterilization, root pieces were rinsed in sterilized distilled water, dried on sterilized filter paper and plated aseptically on potato dextrose agar (PDA) media (Difco Laboratories, Sparks, MD) for seven days at 27 ± 1°C. The mycelium growth was observed in and around the root segments and was purified using the method of Zhang et al. (Citation2009). The fungus was identified as M. phaseolina using microscopic morphological characteristics (Dhingra and Sinclair Citation1978; Barnett and Hunter Citation1987) and confirmed by the sequence of Internal Transcribed Spacer (ITS), according to the methods of White et al. (Citation1990) and Linhai et al. (Citation2011).
Preparation of fungal inoculum
The inoculum of M. phaseolina was prepared by Mihail (Citation1992). The seeds of sorghum (Sorghum bicolor) were soaked overnight in distilled water in Erlenmeyer flasks (500 mL capacity) at room temperature (25 ± 1°C) and autoclaved at 103.4 kPa for 20 min. The autoclaved flasks were inoculated with mycelial disc of M. phaseolina. The flasks were incubated for 15 days at 27 ± 1°C until completely colonized with the masses of micro-sclerotia within the sorghum seed substrate. The culture was dried and blended to a coarse powder. The powdered inoculum was mixed into the experimental soil to obtain a final inoculum density of 200 micro-sclerotia per gram dry weight of soil (Mihail Citation1992).
Pathogenicity test
Randomized complete block design experiment with five replicates was carried out in growth chamber to examine the pathogenicity of M. phaseolina by artificial inoculation. The seeds of mung bean were surface sterilized using 2% sodium hypochlorite (v/v) for 2 min, rinsed in sterilized distilled water. Five seeds were sown in each plastic pot (30 cm in diameter with 10 kg of soil mixture) inoculated with M. phaseolina and pots without M. phaseolina serve as control. Pots were incubated in a growth chamber at 27 ± 2°C with relative humidity of 65%, photocycle of 16 h light (700 µmol/m2/s) and 8 h dark. The percentage of pre-emergence damping-off symptom was recorded at 15 days after sowing. In addition, the post-emergence damping-off symptom and survival rate were recorded at 45 days after sowing. The charcoal rot index was determined by scoring the severity of disease on visual observations of disease symptoms and rated on a 1–4 scale (Bhattacharya et al. Citation1985).
Determination of disease index
The disease index of this study was calculated by the modification of Cralley’s system of disease measurement according to Bhattacharya et al. (Citation1985). The extent of M. phaseolina infection was indicated due to the presence of dark brown lesion and micro-sclerotia of the fungus on root-systems. The healthy and infected seedlings were divided into four groups as follows:
Healthy seedlings (0 = No charcoal root-rot symptom)
Slightly infected (1 = Slight charcoal root-rot symptom seedlings)
Heavily infected (3 = Heavy charcoal root-rot symptom seedlings)
Dead seedlings (4 = Dead seedlings).
Disease index was calculated as follows:where Hn
is the number of healthy seedlings, Sn
is the number of slightly infected seedlings, Hn
is the number of heavily infected seedlings and Dn
is the number of dead seedlings.
In vitro antifungal activity of B. subtilis and their metabolites
B. subtilis, BERA 71was grown in Brain Heart Infusion liquid medium (Oxoid CM 225, Basingstoke, Hampshire, England) at 27 ± 1°C for 60 h (Chitarra et al. Citation2002). The culture was centrifuged (13,000 rpm for 10 min) to harvest the bacterial cells, and the clear supernatant was acidified to pH 2.0 with concentrated HCl and kept in refrigerator at 5.0 ± 1°C for overnight (Chitarra et al. Citation2002). The precipitate was extracted twice with methanol and concentrated by vacuum evaporation, and re-dissolved in known volume of methanol and stored at −20°C. The methanolic extracts were analyzed by thin layer chromatography (TLC) on silica gel 60 F254 plates with layer thickness of 250 µm (Merck, Darmstadt, Germany) as described by Malfanova et al. (Citation2011). Plates were developed in chloroform/methanol/water 65:25:4 (v/v/v) for 1.5 h at room temperature. After drying, the pattern of compounds on the developed plate was visualized using UV254 and stained in an iodine chamber for 5 min at room temperature followed by dipping in 1% aqueous starch. The putative antifungals were preliminarily characterized by their Rf values. Pure iturin A from B. subtilis (Sigma-Aldrich, Steinheim, Germany) was used as a standard. The antifungal impact of iturin A from B. subtilis against M. phaseolina was assayed by disc diffusion method for antimicrobial activity (Bauer et al. Citation1966), PDA (Difco Laboratories, Sparks, MD) medium used as substrate for M. phaseolina culture.
B. subtilis treatment on M.-phaseolina-infected plants
The pots’ experiment was conducted in a completely randomized design with five replications. The seeds of surface-sterilized mung bean were divided into four groups: (1) Control (without any bacterial and fungal inoculation); (2) Bs (seeds treated with B. subtilis); (3) Mp (seeds sown in soil infested with M. phaseolina); (4) Bs + Mp (seeds treated with B. subtilis and sown in soil infested with M. phaseolina). The soil was inoculated by M. phaseolina (200 micro-sclerotia per gram dry soil) as described above in pathogenicity test according to Mihail (Citation1992). B. subtilis was introduced as seed treatment of formulated product described above @ 1.0 g B. subtilis/100 g seeds and 1.0 g of B. subtilis BERA 71 contain 3.6 × 109 CFU. The pots were incubated for eight weeks in growth chamber at 27 ± 2°C with relative humidity of 65%, photocycle of 16 h light (700 µmol/m2/s) and 8 h dark. The disease index was recorded as described above in detail. The plants were removed from the pots, and morphological characters (shoot length and root length) were measured. Samples of fresh leaves were taken from each treatment for estimation of photosynthetic pigments (Lichtenthaler and Wellburn Citation1983), enzymatic and non-enzymatic antioxidants, growth regulators, hydrogen peroxide (H2O2) and malonaldehyde (MDA) contents. The dry weight of shoot and root was measured after drying them in an electric oven at 110°C for 48 h. The dried leaf samples of each treatment were used for estimation of soluble sugars, proline, total free amino acids and elements.
H2O2 and lipid peroxidation assay
H2O2 levels were determined by homogenizing leaf tissue (0.5 g) in 0.1% trichloroacetic acid (TCA) (w/v) followed by centrifugation at 12,000 rpm for15 min. The supernatant (0.5 mL) was added to 0.5 mL potassium phosphate buffer (10 mM, pH 7.0) and 1 mL potassium iodide (1 M). The absorbance of supernatant was measured at 390 nm and H2O2 concentration was determined from standard curve (Sergiev et al. Citation1997). The obtained results were expressed as nmol/g FW. However, the lipid peroxidation in leaves was determined by estimating the formation of thiobarbituric acid (TBA) reactive substance, MDA using the method of Hodges et al. (Citation1999). Absorbance was read at 532 and 600 nm and lipid peroxidation was expressed as nM MDA formed using an extinction coefficient of 155 mM−1 cm−1.
Proline and phenol assay
For proline estimation, 0.5 g of leaf sample was homogenized in sulphosalicylic acid (3%, w/v) and was centrifuged at 10,000 rpm for 10 min. Thereafter, the supernatant was mixed with acid ninhydrin and glacial acetic acid, and later it was incubated at 100°C for 1 h in water bath. Samples were removed and reaction was stopped by keeping samples on ice bath. Proline was extracted with toluene and the absorbance was read at 520 nm (Bates et al. Citation1973). Proline concentration was determined using calibration curve and expressed as µmol proline/g FW. Total phenolics were extracted in ethanol (80%, v/v) and were estimated using Folin and Ciocalteau’s phenol reagent, and optical density of the mixture was read at 750 nm (Slinkard and Singleton Citation1977). Computation was done from the standard curve of pyrogallol.
Antioxidant enzymes assay
Frozen leaf tissue (0.4 g) was homogenized in 4 mL ice-cold buffer (50 mM potassium phosphate, pH 7.0, 4% polyvinyl pyrrolidone) using pre-chilled mortar and pestle and the homogenate was centrifuged at 14,000 rpm at 4°C for 30 min. The supernatant was used as enzyme source for various enzymes. Activity of SOD (EC1.15.1.1) was assayed by measuring its ability to inhibit the photochemical reduction of nitrobluetetrazolium (NBT). Reaction mixture (1 mL) contained 50 mM phosphate buffer (pH 7.4), 13 mM methionine, 75 µM NBT, 0.1 mM ethylenediamine tetraacetic acid (EDTA), 2 µM riboflavin and 100 µL enzyme extract. Samples were incubated for 15 min under fluorescent tubes and reaction was terminated by switching off the light. Absorbance of the reaction mixture was read at 560 nm and amount of protein causing 50% inhibition of photochemical reduction of NBT was considered as one unit of SOD activity and was expressed as U/mg protein (Beauchamp and Fridovich Citation1971). CAT (EC1.11.1.6) activity was assayed from enzyme source by monitoring the decomposition of H2O2 at 240 nm. Reaction mixture was 100 mM phosphate buffer (pH 7.0), 0.1 mM EDTA, 0.1% H2O2 and 100 µL enzyme extract in a final volume of 1 mL. Molar extinction coefficient (ε = 39.4 mM−1 cm−1) was used for calculation of CAT activity and was expressed as U/mg protein (Aebi Citation1984). APX (EC1.11.1.1) activity was assayed by observing the change in absorbance at 290 nm. The reaction mixture (2 mL) contained 50 mM phosphate buffer (pH 7.8), 0.1 mM EDTA, 0.3 mM ascorbate and 100 µL enzyme extract and the reaction was initiated by addition of H2O2 (Nakano and Asada Citation1981). APX activity was calculated by using molar extinction coefficient for AsA (ε = 2.8 mM−1 cm−1). Activity was expressed as U/mg protein. Peroxidase (POD, EC 1.11.1.7) activity was estimated according to Chance and Maehly (Citation1955). In this method, 1 mL reaction mixture contained 750 μL 50 mM phosphate buffer (pH 5.0), 100 μL guaiacol, 100 μL H2O2 and 100 μL enzyme extract. Increase of absorbance at 470 nm was recorded for 3 min at interval of every 20 sec. The enzyme activity was expressed as EU/mg. GR (EC1.6.4.2) activity was determined by following Smith et al. (Citation1988). The reaction mixture consisted of 0.1 M sodium phosphate buffer (pH 7.5), 1 mM EDTA, 0.75 mM 5,5'-dithiobis nitro benzoic acid, 0.1 mM nicotinamide adenine dinucleotide phosphate and 1 mM oxidized glutathione (GSSG). The reaction was started by adding the enzyme source and the absorbance was observed at 412 nm (Smith et al. Citation1988).
Determination of non-enzymatic antioxidants
For estimation of AsA, the leaf samples were powdered in liquid nitrogen and extracted in 6% TCA (w/v). The homogenate was mixed with 2% (w/v) dinitrophenyl-hydrazine (dissolved in 50% H2SO4) and 10% (w/v) thiourea (dissolved in 70% ethanol) and mixture was boiled in a water bath for 15 min. Thereafter it was cooled at room temperature and centrifuged at 1000 rpm for 10 min at 4°C. The resulting pellet was dissolved with 80% H2SO4 and its absorbance was read at 530 nm (Mukherjee and Choudhuri Citation1983). A calibration curve of AsA was taken as standard for calculations. The results were expressed as µmol/g FW. The method of Yu et al. (Citation2003) was used for estimation of the glutathione and standard curves with known concentrations of reduced glutathione (GSH) and GSSG were used for measuring GSH and GSSG content in plants.
Extraction and quantification of plant growth regulators
The leaves were ground and extracted in 80% aqueous acetone (4:1, v/v) containing butylated hydroxytoluene (10 mg/L) and were purified using ethyl acetate and NaHCO3 (Kusaba et al. Citation1998) for quantifying the endogenous levels of plant growth regulators. Abscisic acid (ABA) was extracted as per the method of Qi et al. (Citation1998) as described by Kamboj et al. (Citation1999) and computations were done using standard ABA as reference. Kelen et al. (Citation2004) method was used for quantification of indole acetic acid (IAA) and indole butyric acid (IBA).The purified extract residue was subjected to High Performance Liquid Chromatography on a column of PEGASIL ODS (6 mm i.d. × 150 mm, Senshu Kagaku, Tokyo, Japan), and the concentrations of IAA and IBA were calculated from the standard curves of those hormones.
Nutrients analysis
Leaf samples were dried and powdered to analysis of elements. The samples were digested in concentrated sulfuric acid and nitric acid (1:5, v/v) at 60°C. The concentrations of nutrient extracts of leaf samples were measured according to the method described by Novozamsky et al. (Citation1983). The concentrations of Na+, K+, Mg2+ and Ca2+ were estimated according to the method of Wolf (Citation1982) using a flame photometer (Jenway Flame Photometer, Bibby Scientific Ltd, Stone, Staffordshire, St15 OSA, UK). To the digested samples, 1 M hydrochloric acid was added, and the dried leaf powder and their elemental (Mn, Fe, Cu and Zn) concentrations were determined by atomic absorption 436 spectrophotometry (AAS) (Z-5000, Hitachi, Japan). Standard curve of each element is used as reference. The element concentration was expressed as mg/g DW (Na+, K+, Mg2+ and Ca2+) and µg/g DW (Mn, Fe, Cu and Zn).
Statistical analysis
All experiments were repeated three times and obtained results were statistically analyzed. Least significant differences (LSD), the analysis of variance and Pearson correlation analysis between patterns of systemic acquired resistance were carried out according to SAS v9.1 software.
Results
In vitro bio-control efficacy of B. subtilis
In vitro dual culture of B. subtilis (Bera 71) and M. phaseolina was performed so as to know the potential of cyclic antifungal lipopeptide (3.0 mM iturin A) produced by B. subtilis Bera 71. The severe inhibition of M. phaseolina growth was observed by iturin A produced by B. subtilis Bera 71 ().
Bio-control effect of B. subtilis against M.-phaseolina-infected plants
M. phaseolina caused charcoal rot disease and severely damaged mung bean. Pre-mergence, post-emergence, survival of M.-phaseolina-infected seedlings and disease severity was recorded as 33.33%, 20.33%, 33.00% and 3.88%, respectively (). Application of B. subtilis showed a significant inhibition on disease incidence caused by M. phaseolina. However, the survival rate of healthy plants was significantly increased as 82.14% due to the effect of B. subtilis when compared M.-phaseolina-affected plants.
Table 1. Effect of B. subtilis (Bs) on disease status of mung bean infected by M. phaseolina (Mp).
Effect of B. subtilis on plant growth of diseased plants
Plant growth of mung bean is altered during the infection of M. phaseolina and treatment of B. subtilis. The detrimental effect of M. phaseolina on plants showed the phenotypic changes (). However, plants inoculated with B. subtilis exhibited an increase of plant growth and biomass. M.-phaseolina-infected plants showed a reduction of 68.15% and 69.34% in length of shoot and root and a reduction of 50.80% and 54.54% in dry weight of shoot and root over control. The interaction of B. subtilis to M.-phaseolina-infected plants mitigated the adverse effect by increasing dry weight of shoot (31.90%) and root (45.34%).
Table 2. Effect of B. subtilis (Bs) on plant growth of M.-phaseolina (Mp)-infected mung bean.
Influence of B. subtilis on chlorophyll pigments of diseased plants
The synthesis of chlorophyll in mung bean was modulated at an association of B. subtilis and M.-phaseolina-induced charcoal rot disease (). The application of B. subtilis induced the synthesis of chlorophyll pigments. The photosynthetic pigments such as chlorophyll a, chlorophyll b and total chlorophyll were reduced as 37.98%, 35.91% and 60.26%, respectively than their controls. However, these adverse effects on pigments synthesis were significantly mitigated during the inoculation of B. subtilis by stimulating an increase of total chlorophyll (33.61%) content.
Table 3. Effect of B. subtilis (Bs) on chlorophyll content of mung bean infected by M. phaseolina (Mp).
Correlation between plant growth and photosynthetic pigments
B. subtilis induced a positive effect on M. phaseolina diseased plants, which was revealed by the Pearson correlation between chlorophyll a, chlorophyll b, total chlorophyll, shoot and root length, and shoot and root dry weight (). Chlorophyll a showed a strong positive correlation with root dry weight (0.99064), root length (0.98000), shoot dry weight (0.94243), shoot height (0.88070), total chlorophyll (0.99812) and chlorophyll b (0.96454), whereas Pearson correlation coefficients (r) were <0.0001 in all above attributes except shoot height (0.0002). Chlorophyll b was recorded as a strong positive correlation with total chlorophyll, shoot length, shoot dry weight, root length and root dry weight. Total chlorophyll also showed a strong positive correlation with shoot height, shoot dry weight, root length and root dry weight. However, the result of shoot height expressed a strong positive correlation with shoot dry weight, root length, root dry weight. In addition, shoot dry weight of plants showed a positive correlation effect with root length and root dry weight.
Table 4. Pearson correlation coefficients (r) between chlorophyll a (Chla), chlorophyll b (Chlb), total chlorophyll (Tot Chl), shoot height (SH), shoot dry weight (Sdwt), root length (RL), root dry weight (Rdwt).
Effect of B. subtilis on H2O2 and lipid peroxidation in infected plants
The positive interaction of B. subtilis in plants was detected by reduction of H2O2 and membrane lipid peroxidation than control plants (). Charcoal rot induced by M. phaseolina resulted in a higher production of H2O2 (20.64%) and lipid peroxidation (38.34%) over control plants. However, the inoculation of B. subtilis to M.-phaseolina-infected plants alleviated the oxidative stress due to a reduction of H2O2 and MDA content as 15.05% and 31.60%, respectively.
Changes of non-enzymatic antioxidants in diseased plants by B. subtilis interaction
The effect of B. subtilis and M. phaseolina on total phenols (TPs), AsA, GSH and GSSG, in plants is presented in . Mung bean infected with M. phaseolina showed an increase of TP (44.47%), GSH (51.45%) and GSSG (64.72%). However, as synthesis was decreased when compared to their control. B. subtilis colonization with M.-phaseolina-infected plants significantly enhanced the content of AsA and reduced TP, GSH, GSSG content and, while no changes in GSH/GSSG ratio.
B. subtilis adjusted the osmolytes synthesis in infected plants
The osmolytes such as soluble sugars, proline and total free amino acids concentration was changed by the effect of pathogen and bio-control agents in plants (). The application of B. subtilis accelerated a synthesis of 7.17% and 8.45% in soluble sugars and proline content, respectively. M.-phaseolina-infected plants showed 8.3%, 28.1% and 20.64% of increase in soluble sugars, proline and total free amino acids, while those osmolytes and amino acids production were significantly declined due to the effect of B. subtilis association.
Table 5. Effect of B. subtilis (Bs) on soluble sugar, proline and total free amino acids in mung bean infected by M. phaseolina (Mp).
Effect of B. subtilis on plant hormones in infected plants
The results of the present study revealed that plant growth hormones IAA and IBA, and also the stress-responsible hormone ABA were affected by the infection of M. phaseolina and treatment of B. subtilis in mung bean (). The inoculation of B. subtilis was found to enhance the production of auxins (including IAA and IBA), with a considerable reduction in ABA biosynthesis. M.-phaseolina-infected plants showed a reduction of those auxins and ABA. However, the co-inoculation of B. subtilis to M.-phaseolina-infected plants increased the production of IAA (47.15%) and IBA (38.97%), and decreased ABA production to alleviate the biotic stress.
Table 6. Effect of B. subtilis (Bs) on IAA, IBA and ABA in mung bean infected by M. phaseolina (Mp).
Correlation between growth regulators and photosynthetic pigments
The bio-control and stress mitigation effect of B. subtilis against M. phaseolina in plants were revealed with a correlation matrix between chlorophyll a, chlorophyll b, total chlorophyll, IAA, IBA and ABA (). Chlorophyll a showed a positive correlation with chlorophyll b, total chlorophyll, IAA and IBA, while adverse negative effect was recorded on ABA. Similarly, chlorophyll b content positively correlated with the synthesis of total chlorophyll, IAA and IBA, and on the other hand, a negative relation was observed in ABA accumulation. The growth hormones had the positive effect as an increased synthesis of IAA and IBA. The results obtained from IAA analysis showed a strong positive correlation with IBA, and while it expressed a negative effect with ABA. In addition, IBA production had a significant negative relation with ABA in this experiment.
Table 7. Pearson correlation coefficients (r) between chlorophyll a (Chla), chlorophyll b (Chlb), total chlorophyll (Tot Chl), IAA, IBA and ABA.
Role of B. subtilis on antioxidant enzymes in infected plants
The biotic interaction of B. subtilis and M. phaseolina in mung bean significantly increased the antioxidant enzyme activities (). Activity of SOD, CAT, POD, APX and GR was significantly increased to 36.87%, 35.05%, 42.85%, 24.63% and 16.15%, respectively in M.-phaseolina-infected plants than their control, while further stimulation of SOD (34.01%), CAT (21.37%), POD (19.48%), APX (16.89%) and GR (39.66%) was observed due to B. subtilis inoculation to mitigate the stress effects.
Correlation between growth regulators and antioxidant enzymes activity
The interaction of plant hormones (IAA, IBA and ABA) and efficacy of antioxidant enzymes (SOD, CAT, POD, APX and GR) were correlated to estimate the bio-control effects of B. subtilis in M.-phaseolina-infected mung bean (). The production of SOD showed a positive correlation with CAT, POD, PPX and GR, and while it had negative relation with IAA and IBA with a positive effect with ABA. The results of CAT assay expressed as a significant positive interaction with POD, APX and GR, also showed a negative relation with IAA and IBA along with a positive correlation in ABA synthesis. POD activity positively correlated with APX and GR, and while negatively correlated with IAA and IBA production. However, APX showed a significantly positive relation with GR, while a positive and non-significant correlation with ABA. The activity of GR had negative and non-significant correlation with IAA and IBA, while it showed a positive and significant correlation with ABA. The IAA concentration expressed a significant positive correlation with IBA and negative relation with the concentration of ABA. IBA synthesis showed a negative and significant correlation in ABA production.
Table 8. Pearson correlation coefficients (r) between SOD, CAT, POD, APX, GR, IAA, IBA and ABA.
B. subtilis induced changes of macro and micro nutrients in diseased plants
The nutrients uptake in leaves of mung bean was significantly influenced by the interaction of B. subtilis and the infection of M. phaseolina ( and ). B. subtilis association promoted the macro and micro nutrients (N, Na, K, Ca, Mg, Zn, Cu, Mn and Fe) uptake by mung bean roots. On the other hand, M. phaseolina decreased the uptake of those nutrients, except sodium. The accumulation of N, K, Ca, Mg, Zn, Cu, Mn and Fe was found to decline as 48.9%, 50.4%, 67.3%, 48.2%, 26.3%, 62.0%, 38.2% and 33.33%, respectively in charcoal-rot-diseased plants. However, B. subtilis significantly ameliorated the detrimental effect of M. phaseolina infection by enhancing N (23.2%), K (21.34%), Ca (32.3%), Mg (35.1%), Zn (12.8%), Cu (17.6%), Mn (12.7%) and Fe (12.0%) uptake.
Table 9. Effect of B. subtilis (Bs) on macro nutrients in M.-phaseolina (Mp)-infected mung bean.
Table 10. Effect of B. subtilis (Bs) on micro nutrients in mung bean infected by M. phaseolina (Mp).
Discussion
M. phaseolina caused charcoal rot disease in a wide range of host plant species, including corn, sunflower and some weeds (El-Hai et al. Citation2009).The endophytic bacteria produce antifungal metabolites to prevent the growth of pathogens. We found that B. subtilis produced the iturin A, which is involved in controlling M. phaseolina mycelial growth. Iturin A, similarly to antifungal peptide, destructs the pathogenic fungal cell wall and cause severe mortality of pathogen population in rhizosphere (Yamamoto et al. Citation2015). Current study results showed that M. phaseolina induce the disease and reduce the growth of mung bean. The infected plants inoculated with B. subtilis displayed less damaging effects of M. phaseolina. The plant growth promotion activity of B. subtilis was significant due to enhancement in the accumulation of sugars, proline and free amino acids, which are considered as the key osmolytes for maintaining the cellular water content to protect the structures and functions of cellular organelles (Ahanger and Agarwal Citation2017). In a previous report, it is suggested that the association of B. megaterium increased the plant growth by stimulating the synthesis of sucrose, glucose, fructose and several amino acids (Kang et al. Citation2014). The sugars, proline and amino acids accumulation involve as antioxidants for neutralizing the toxic ROS under stress conditions, which is leading to maintain the structure of proteins and membranes (Hayat et al. Citation2012). The osmotic constituents of cells reduce the need of water to active metabolism and extend plant survival (Zhifang and Loescher Citation2003). The accumulation of proline, sugars and amino acids in plants inoculated with B. subtilis provide the resistance against charcoal rot disease (Upadhyay et al. Citation2012). In addition, amino acids act as rich sources of organic nitrogen to stimulate plant metabolism and precursor for alkaloid biosynthesis (Meloni et al. Citation2001).
Inoculation of B. subtilis to M.-phaseolina-infected mung bean significantly increased the synthesis of auxins, including IAA and IBA, resulting in protection of the mung bean against charcoal rot disease. A stimulation of growth hormones, including auxins in host plants, efficiently induces the root growth (Patten and Glick Citation2002). Auxin plays a vital role in cell division, elongation, apical dominance and root initiation and growth (Teale et al. Citation2006). The activation of higher synthesis of auxins in infected plants during the interaction of B. subtilis might be the reason for increasing the length and biomass of roots in mung bean.
Another plant hormone (ABA) is considered as a stress-responsible signaling molecule, which involves in the regulation of stomatal functions and in adaptation of plants against adverse environmental conditions, and while their role in plant defense is not well defined (Mauch-Mani and Mauch Citation2005). In the present study, charcoal rot disease caused a considerable restriction in the endogenous production of auxins while accelerated the synthesis of ABA. B. subtilis mitigated the pathogenic stress effects by inhibiting the synthesis of ABA in M.-phaseolina-affected mung bean. Our results were correlated with a previous study showing that Rhizoctonia- solani-induced accumulation of ABA was declined in plants during the inoculation of B. amyloliquefaciens (Kang et al. Citation2015). Porcel et al. (Citation2014) has demonstrated that the optimal endogenous concentration of ABA is important for growth promotion through the regulation of photosynthetic attributes and. ABA signaling is interconnected with ROS production, Ca2+ signaling and activity of mitogen-activated protein kinase in cells and regulates the plasmodesmata to prevent the pathogen infection (Rezzonico et al. Citation1998).
M. phaseolina triggered the excess production of ROS such as H2O2 in mung bean plants, resulting in the peroxidation of membrane lipids, which was ameliorated by the effect of B. subtilis. The pathogenic-stress-induced lipid peroxidation affects the fluidity, proteins, receptors, enzymes and ion channels of membrane (Gill and Tuteja Citation2010). The bio-control agents can prevent the pathogen-generated oxidative burst in diseased plants by inhibiting lipid peroxidation (Radhakrishnan et al. Citation2013). The upregulation of antioxidant enzymes due to B. subtilis inoculation protected the M.–phaseolina-infected plants’ metabolism by imparting fast removal of ROS. The activity of SOD eliminates superoxide radicals, leading to protect the photosynthetic apparatus (Khan et al. Citation2015).The formation of H2O2 is scavenged by CAT, POD and APX in ascorbate-glutathione cycle due to the assistance of GR, AsA and glutathione (Ahanger and Agarwal Citation2017). Optimal production of AsA and GSH in B.-subtilis-inoculated plants resulted in improvement of the activity of APX and GR, leading to regulation of the ascorbate-glutathione pathway for the elimination of H2O2. Hence, the efficiency of B. subtilis was depicted an enhanced resistance potential of mung bean against charcoal rot disease. B. subtilis induced an increase in activity of SOD, APX and CAT, modulated the production of superoxide and H2O2, and therefore prevented the initiation of the Haber–Weiss reaction, hydroxyl (OH–) radical generation and dis-functioning of membrane. The enhanced activities of antioxidant enzymes in B.-subtilis-inoculated plants can mediate the plant growth by eliminating the free radicals and maintaining photosynthetic rate, cellular redox potential and membrane integrity (Abd_Allah et al. Citation2015; Ahanger et al. Citation2015). The activity of SOD was enhanced in B.- subtilis-inoculated plants, which showed that the strengthened antioxidant system for removal of ROS and hence amelioration of negative effects of M. phaseolina. The bio-control agents triggered the antioxidants’ activities to reduce the toxic effect of ROS and induce the disease resistance in infected plants (Radhakrishnan et al. Citation2013; Kang et al. Citation2015).
Pathogen infection declines the uptake of nutrients by plant roots. In the present study, M. phaseolina caused an inhibition of macronutrients and micronutrients (N, K, Ca, Mg, Zn, Cu, Mn and Fe), but enhanced the accumulation of Na+. Plant pathogenic fungi attack root system and limit the absorption, assimilation and translocation of nutrients in roots and other parts of diseased plant (Marschner Citation1995; Huber and Graham Citation1999; Dordas Citation2008). Moreover, pathogens utilize nutrients for their growth and survival, which results in deficiency of nutrients’ availability to plant, thereby increasing disease susceptibility (Spann and Schumann Citation2009).The secondary metabolites of plant pathogens such as mycotoxins enhance the opening of stomata via activating an H+-pump in the plasma membrane through stimulation of H+-ATPase, which creates an electrochemical gradient to drive elements as K+ influx into guard cells (Zeng et al. Citation2010; Dong et al. Citation2012; Dehgahi et al. Citation2015).
PGPR strains are actively colonizing the plant roots and exert valuable effects on development of plants either by using their own metabolites such as phosphate solubilization, phytohormone production and nitrogen fixation or may impart direct positive affects in plant metabolism by increasing the uptake of water and minerals and enzyme activity in their host plants (Perez-Montano et al. Citation2014). B.- subtilis-inoculated mung bean seedlings maintained the Na/K ratio in disease-infected and non-infected plants; thereby it is strongly suggested that the use of B. subtilis can promote the plant growth of mung bean during biotic stress conditions. The low K+-induced transcriptional up-regulation of the genes encoding HAK5-like transporters occurs through a signal cascade that includes changes in the membrane potential of root cells and increases in ethylene and ROS (Nieves-Cordones et al. Citation2016). The higher concentration of minerals in plants inoculated with B. subtilis might increase the production of metabolites, proteins and also the expression of defense genes against charcoal rot disease (Nadeem et al. Citation2014).
B. subtilis enhanced the uptake of N, K, Ca, Mg, Zn, Cu, Mn and Fe, which resulted to enhance the plant growth at disease condition might be the regulation of various metabolic pathways such as antioxidant system and chlorophyll synthesis. The optimal concentration of zinc helped upregulate antioxidant system and synthesize protective metabolites due to the activity of phenylalanine ammonia lyase and tyrosine ammonia lyase (Wadhwa et al. Citation2014). The results of the present study were correlated with a previous report suggesting that B. methylotrophicus enhances the uptake of several nutrients (N, K, P, Mg, Fe and Zn), and the synthesis of amino acids and chlorophylls (Radhakrishnan and Lee Citation2016). The present study revealed that an increase of Mg concentration might help synthesize the chlorophyll content in B.-subtilis-associated plants and accelerate the photosynthesis in pathogen-infected plants.
Conclusion
The endophytic bacterium, B. subtilis proved its ability to promote plant growth and disease resistance while stimulating mung bean plant growth under normal and M.-phaseolina-infected conditions. Mung bean plants treated with B. subtilis exhibited an ability to inhibit oxidative damage to membranes, which can be ascribed to up-regulation of antioxidant system and accumulation of osmolytes. This work suggested that B. subtilis can be exploited to protect mung bean from charcoal rot and to sustainably improve the growth and yield.
Disclosure statement
No potential conflict of interest was reported by the authors.
ORCID
Elsayed Fathi Abd_Allah http://orcid.org/0000-0002-8509-8953
Additional information
Funding
References
- Abd_Allah EF, Hashem A, Alqarawi AA, Alwathnani HA. 2015. Alleviation of adverse impact of cadmium stress in sunflower (Helianthus annuus L.) by arbuscular mycorrhizal fungi. Pak J Bot. 47(2):785–795.
- Aebi H. 1984. Catalase in vitro. Methods Enzymol. 105:121–126. doi: 10.1016/S0076-6879(84)05016-3
- Ahanger MA, Agarwal RM. 2017. Potassium improves antioxidant metabolism and alleviates growth inhibition under water and osmotic stress in wheat (Triticum aestivum L). Protoplasma. 254(4):1471–1486. doi: 10.1007/s00709-016-1037-0
- Ahanger MA, Agarwal RM, Tomar NS, Shrivastava M. 2015. Potassium induces positive changes in nitrogen metabolism and antioxidant system of oat (Avena sativa L. cultivar Kent). J Plant Int. 10(1):211–223.
- Bais HP, Fall R, Vivanco JM. 2004. Biocontrol of Bacillus subtilis against infection of Arabidopsis roots by Pseudomonas syringae is facilitated by biofilm formation and surfactin production. Plant Physiol. 134:307–319. doi: 10.1104/pp.103.028712
- Barnett HI, Hunter BB. 1987. Illustrated genera of imperfect fungi. 4th ed. New York (NY): Millan.
- Bates LS, Waldre RP, Teare ID. 1973. Rapid determination of free proline for water stress studies. Plant Sci. 39:205–207.
- Bauer AW, Kirby WM, Sherris JC, Turck M. 1966. Antibiotic susceptibility testing by a standardized single disk method. Am J Clin Pathol. 45(4):493–496.
- Beauchamp C, Fridovich I. 1971. Superoxide dismutase: improved assays and an assay applicable to acrylamide gels. Anal Biochem Rev. 44:276–287. doi: 10.1016/0003-2697(71)90370-8
- Bhattacharya D, Basu S, Chattapadhyay JP, Bose S. 1985. Biocontrol of Macrophomina root rot disease of jute by an antagonistic organism, Aspergillus versicolor. Plant Soil. 87:435–438. doi: 10.1007/BF02181910
- Brannen P, Kenney D. 1997. Kodiak – a successful biological control product for suppression of soil-borne plant pathogens of cotton. Indian J Microbiol Biotechnol. 19:169–171. doi: 10.1038/sj.jim.2900439
- Cawoy H, Bettiol W, Fickers P, Ongena M. 2011. Bacillus-based biological control of plant diseases. In: Stoytcheva, editor. Pesticides in the modern world – pesticides use and management. Croatia: Tech Academic Press; p. 273–302.
- Chance B, Maehly C. 1955. Assay of catalase and peroxidases. Methods Enzymol. 2:764–775. doi: 10.1016/S0076-6879(55)02300-8
- Chitarra SC, Breeuwer P, Nout RJM, van-Aelst CA, Rombouts MF, Abee T. 2002. An antifungal compound produced by Bacillus subtilis YM 10-20 inhibits germination of Penicillium roqueforti conidiospores. J Appl Microbiol. 96:159–166.
- Correa OS, Montecchia MS, Berti MF, Ferrari MCF, Pucheu NL, Kerber NL, Garcia AF. 2009. Bacillus amyloliquefaciens BNM122, a potential microbial biocontrol agent applied on soybean seeds, causes a minor impact on rhizosphere and soil microbial communities. Appl Soil Ecol. 41:185–194. doi: 10.1016/j.apsoil.2008.10.007
- Dehgahi R, Subramaniam S, Zakaria L, Joniyas A, BeikiFirouzjahi F, Haghnama K, Razinataj M. 2015. Review of research on fungal pathogen attack and plant defense mechanism against pathogen. Int J AgrSci Res. 2(8):197–208.
- Dhingra OD, Sinclair JB. 1978. Biology and pathology of Macrophomina phaseolina. Minosa: Universidade Federal de-Viscosa; p. 1–166.
- Dong X, Ling N, Wang M, Shen Q, Guo S. 2012. Fusaric acid is a crucial factor in the disturbance of leaf water imbalance in fusarium-infected banana plants. Plant Physiol Biochem. 60:171–179. doi: 10.1016/j.plaphy.2012.08.004
- Dordas C. 2008. Role of nutrients in controlling plant diseases in sustainable agriculture: a review. Agron Sustain Dev. 28:33–46. doi: 10.1051/agro:2007051
- Egamberdieva D, Hashem A, Abd_Allah EF. 2014. Biological control of fungal disease by rhizobacteria under saline soil conditions. In: Ahmad P, editor. Emerging technologies and management of crop stress tolerance. DOI:10.1016/B978-0-12-800875-1.00007-7.
- El-Hai KM, El-Metwally MA, El-BAz SM, Zeid AM. 2009. The use of antioxidants and microelements for controlling damping off caused by Rhizoctonia solani and charcoal rot caused by Macrophomina phasoliana on sunflower. Plant Pathol J. 8(3):79–89. doi: 10.3923/ppj.2009.79.89
- Freitas MA, Medeiros FHV, Carvalho SP, Guilherme LRG, Teixeira WD, Zhang H, Pare PW. 2015. Augmenting iron accumulation in cassava by the beneficial soil bacterium Bacillus subtilis (GBO3). Front Plant Sci. 6:2148. doi: 10.3389/fpls.2015.00596
- Gill SS, Tuteja N. 2010. Reactive oxygen species and antioxidant machinery in abiotic stress tolerance in crop plants. Plant Physiol Biochem. 48:909–930. doi: 10.1016/j.plaphy.2010.08.016
- Hashem A, Abd_Allah EF, Al-Obeed RS, Mridha MAU, Al-Huqail AA. 2013. Non-chemical strategies to control postharvest losses and extend the shelf life of table grape fruits. Biol Agricult Horticult. 29(2):82–90. doi: 10.1080/01448765.2013.763735
- Hashem A, Abd_Allah EF, Alqarawi AA, Al-Huqail AA, Shah M. 2016. Induction of osmoregulation and modulation of salt stress in Acacia gerrardii Benth. by arbuscular mycorrhizal fungi and Bacillus subtilis (BERA 71). Bio Med Res. Article ID 6294098:11. DOI:10.1155/2016/6294098.
- Hayat S, Hayat Q, Alyemeni MN, Wani AS, Pichtel J, Ahmad A. 2012. Role of proline under changing environments: a review. Plant Sig Beh. 7:1–11. doi: 10.4161/psb.7.1.18574
- Hodges DM, DeLong JM, Forney CF, Prange RK. 1999. Improving the thiobarbituric acid-reactive substances assay for estimating lipid peroxidation in plant tissues containing anthocyanin and other interfering compounds. Planta. 207(4):604–611. doi: 10.1007/s004250050524
- Huber DM, Graham RD. 1999. The role of nutrition in crop resistance and tolerance to disease. In: Rengel Z, editor. Mineral nutrition of crops fundamental mechanisms and implications. New York: Food Product Press; p. 205–226.
- Kamboj JS, Blake PS, Quinlan JD, Baker DA. 1999. Identification and quantitation by GC-MS of zeatin and zeatin riboside in xylem sap from rootstock and scion of grafted apple trees. Plant Growth Reg. 28:199–205. doi: 10.1023/A:1006292309765
- Kang SM, Radhakrishnan R, Lee IJ. 2015. Bacillus amyloliquefaciens subsp. plantarum GR53, a potent biocontrol agent resists Rhizoctonia disease on Chinese cabbage through hormonal and antioxidants regulation. World J Microbiol Biotechnol. 31(10):1517–1527. doi: 10.1007/s11274-015-1896-0
- Kang SM, Radhakrishnan R, You YH, Joo GJ, Lee IJ, Lee KE, Kim JH. 2014. Phosphate solubilizing Bacillus megaterium mj1212 regulates endogenous plant carbohydrates and amino acids contents to promote mustard plant growth. Indian J Microbiol. 54(4):427–433. doi: 10.1007/s12088-014-0476-6
- Kaushik CD, Chand JN, Saryavir. 1987. Seedborne nature of Rhizoctonia bataticola causing leaf blight of mung bean. Indian J Mycol Plant Pathol. 17:153–157.
- Kelen M, Cubek-Demiralay E, Sen S, Ozkan G. 2004. Separation of abscisic acid, indole-3-acetic acid, gibberellic acid in 99 R (Vitis berlandieri x Vitis rupestris) and rose oil (Rosa damascena Mill.) by reversed phase liquid chromatography. Turkish J Chem. 28:603–610.
- Khan MIR, Nazir F, Asgher M, Per TS, Khan NA. 2015. Selenium and sulfur influence ethylene formation and alleviate cadmium-induced oxidative stress by improving proline and glutathione production in wheat. J Plant Physiol. 173:9–18. doi: 10.1016/j.jplph.2014.09.011
- Kusaba S, Kano-Murakami Y, Matsuoka M, Tamaoki M, Sakamoto T, Yamaguchi I, Fukumoto M. 1998. Alteration of hormone levels in transgenic tobacco plants over expressing a rice homeobox gene OSH1. Plant Physiol. 116:471–476. doi: 10.1104/pp.116.2.471
- Lee DK, Ahn S, Cho HY, Yun HY, Park JH, Lim J, Lee J, Kwon SW. 2016. Metabolic response induced by parasitic plant-fungus interactions hinder amino sugar and nucleotide sugar metabolism in the host. Sci Rep. 6:352. doi:10.1038/srep37434.
- Lichtenthaler HK, Wellburn AR. 1983. Determinations of total carotenoids and chlorophylls a and b of leaf extracts in different solvents. Biochem Soc Trans. 11:591–592. doi: 10.1042/bst0110591
- Linhai W, Yanxin Z, Donghua L, Junbin H, Wenliang W, Haixia L, Xiurong Z. 2011. Variations in the isolates of Macrophomina phaseolina from sesame in China based on amplified fragment length polymorphism (AFLP) and pathogenicity. Afr J Microbiol Res. 5(31):5584–5590. doi:10.5897/AJMR11.306.
- Lugtenberg B, Kamilova F. 2009. Plant-growth-promoting rhizobacteria. Ann Rev Microbiol. 63:541–556. doi: 10.1146/annurev.micro.62.081307.162918
- Malfanova N, Kamilova F, Validov S, Shcherbakov A, Chebotar V, Tikhonovich I, Lugtenberg B. 2011. Characterization of Bacillus subtilis HC8, a novel plant-beneficial endophytic strain from giant hogweed. Microbial Biotechnol. 4(4):523–532. doi: 10.1111/j.1751-7915.2011.00253.x
- Marschner H. 1995. Mineral nutrition of higher plants.2nd ed.London: Academic Press; p. 889.
- Mauch-Mani B, Mauch F. 2005. The role of abscisic acid in plant–pathogen interactions. Curr Opin Plant Biol. 8:409–414. doi: 10.1016/j.pbi.2005.05.015
- Meloni DA, Oliva MA, Ruiz HA, Martinez CA. 2001. Contribution of proline and inorganic solutes to osmotic adjustment in cotton under salt stress. J Plant Nutr. 24:599–612. doi: 10.1081/PLN-100104983
- Mihail JD. 1992. Macrophomina phaseolina. In: Singleton LL, Mihail JD, Rush CM, editors. Methods for research on soil borne phytopathogenic fungi. St. Paul: APS Press; p. 134–136.
- Mittler R. 2002. Oxidative stress, antioxidants and stress tolerance. Trends Plant Sci. 7:405–410. doi: 10.1016/S1360-1385(02)02312-9
- Mukherjee SP, Choudhuri MA. 1983. Implications of water stress-induced changes in the levels of endogenous ascorbic acid and hydrogen peroxide in Vigna seedlings. Physiol Plant. 58:166–170. doi: 10.1111/j.1399-3054.1983.tb04162.x
- Nadeem SM, Ahmad M, Zahir ZA, Javaid A, Ashraf M. 2014. The role of mycorrhizae and plant growth promoting rhizobacteria (PGPR) in improving crop productivity under stressful environments. Biotechnol Adv. 32:429–448. doi: 10.1016/j.biotechadv.2013.12.005
- Nagorska K, Bikowski M, Obuchowskji M. 2007. Multicellular behaviour and production of a wide variety of toxic substances support usage of Bacillus subtilis as a powerful biocontrol agent. Acta Biochimica Polonica. 54:495–508.
- Nakano Y, Asada K. 1981. Hydrogen peroxide is scavenged by ascorbate specific peroxidase in spinach chloroplast. Plant Cell Physiol. 22:867–880.
- Ngugi H, Dedej S, Delaplane K, Savelle A, Scherm H. 2005. Effect of flower-applied serenade biofungicide (Bacillus subtilis) on pollination-related variables in rabbit eye blueberry. Biol Control. 33:32–38. doi: 10.1016/j.biocontrol.2005.01.002
- Nieves-Cordones M, Rodenas R, Chavanieu A, Rivero RM, Martinez V, Gaillard I, Rubio F. 2016. Uneven HAK/KUP/KT protein diversity among angiosperms: species distribution and perspectives. Front Plant Sci. 7:127. doi:10.3389/fpls.2016.00127.
- Novozamsky VJG, Houba VJG, van-Eck R, van-Vark W. 1983. A novel digestion technique for multi-element plant analysis. Comm Soil Sci Plant Anal. 14:239–248. doi: 10.1080/00103628309367359
- Oliva J, Mulero J, Paya P, Cámara MA, Barba A. 2009. Influence of several fungicides on the antioxidant activity of red wines (Var. Monastrell). J Environ Sci Health B. 44(6):546–552. doi: 10.1080/03601230902997758
- Ongena M, Jacques P. 2008. Bacillus lipopeptides: versatile weapons for plant disease biocontrol. Trends Microbiol. 16:115–125. doi: 10.1016/j.tim.2007.12.009
- Patten CL, Glick BR. 2002. Role of Pseudomonas putida indole acetic acid in development of the host plant root system. Appl Environ Microbiol. 68:3795–3801. doi: 10.1128/AEM.68.8.3795-3801.2002
- Perez-Montano F, Alías-Villegas C, Bellogín RA, del Cerro P, Espuny MR, Jiménez-Guerrero I, López-Baena FJ, Ollero FJ, Cubo T. 2014. Plant growth promotion in cereal and leguminous agricultural important plants: from microorganism capacities to crop production. Microbiol. Res. 169:325–336. doi: 10.1016/j.micres.2013.09.011
- Piggot PJ, Hilbert DW. 2004. Sporulation of Bacillus subtilis. Curr Opin Microbiol. 7:579–586. doi: 10.1016/j.mib.2004.10.001
- Porcel R, Zamarreno AM, Garcia-Mina JM, Aroca R. 2014. Involvement of plant endogenous ABA in Bacillus megaterium PGPR activity in tomato plants. BMC Plant Biol. 14:36–48. doi: 10.1186/1471-2229-14-36
- Qi QG, Rose PA, Abrams GD, Taylor DC, Abrams SR, Cutler AJ. 1998. Abscisic acid metabolism, 3-ketoacyl-coenzyme a synthase gene expression and very-long-chain monounsaturated fatty acid biosynthesis in Brassica napus embryos. Plant Physiol. 117:979–987. doi: 10.1104/pp.117.3.979
- Radhakrishnan R, Lee IJ. 2016. Gibberellins producing Bacillus methylotrophicus KE2 supports plant growth and enhances nutritional metabolites and food values of lettuce. Plant Physiol Biochem. 109:181–189. doi: 10.1016/j.plaphy.2016.09.018
- Radhakrishnan R, Shim KB, Lee BW, Hwang CD, Pae SB, Park CH, Kim SU, Lee CK, Baek IY. 2013. IAA producing Penicillium sp. NICS01 triggers plant growth and suppresses fusarium induced oxidative stress in sesame (Sesamum indicum L.). J Microbiol Biotechnol. 23(6):856–863. doi: 10.4014/jmb.1209.09045
- Rezzonico E, Flury N, Meins FJ, Beffa R. 1998. Transcriptional down regulation by abscisic acid of pathogenesis-related beta-1,3-glucanase genes in tobacco cell cultures. Plant Physiol. 117:585–592. doi: 10.1104/pp.117.2.585
- Sandhu A, Singh RD. 1998. Role of seed borne inoculum in development of charcoal rot of cowpea. J Mycol Plant Pathol. 28:193–195.
- Sergiev I, Alexieva V, Karanov E. 1997. Effect of spermine, atrazine and combination between them on some endogenous protective systems and stress markers in plants. Comp Ren Del Academie Bul des Sci. 51:121–124.
- Slinkard K, Singleton VL. 1977. Total phenol analyses: automation and comparison with manual methods. Am J Enol Viticult. 28:49–55.
- Smith IK, Vierheller TL, Thorne CA. 1988. Assay of glutathione reductase in crude tissue homogenates using 5,5′-dithiobis (2-nitrobenzoic acid). Anal Biochem. 175:408–413. doi: 10.1016/0003-2697(88)90564-7
- Spann TM, Schumann AW. 2009. The role of plant nutrients in disease development with emphasis on citrus and huanglongbing. Proc Fla State Hort Soc. 122:169–171.
- Taylor SR, Weaver BD, Wood WC, van Edzard S. 2005. Nitrogen application increases yield and early dry matter accumulation in late-planted soybean crop. Sci J. 45:854–858.
- Teale W, Paponov I, Palme K. 2006. Auxin in action: signalling, transport and the control of plant growth and development. Nat Rev Mol Cell Biol. 7:847–859. doi: 10.1038/nrm2020
- Upadhyay SK, Singh JS, Saxena AK, Singh DP. 2012. Impact of PGPR inoculation on growth and antioxidant status of wheat under saline conditions. Plant Biol. 14:605–611. doi: 10.1111/j.1438-8677.2011.00533.x
- Vellosillo T, Vicente J, Kulasekaran S, Hamberg M, Castresana C. 2010. Emerging complexity in reactive oxygen species production and signaling during the response of plants to pathogens. Plant Physiol. 154:444–448. doi: 10.1104/pp.110.161273
- Wadhwa S, Singhal S, Rawat S. 2014. Bioavailability enhancement by piperine: a review. Asian J Biomed Pharma Sci. 4(36):1–8.
- White T, Bruns T, Lee S, Taylor J. 1990. Chap 38. Amplification and direct sequencing of fungal ribosomal RNA genes for phylogenetics. In: Inns MA, Gelfand DH, Sninsky JJ, White TJ, editors. PCR protocols: a guide to methods and applications. Orlando,FL: Academic press; p. 315–322.
- Wolf B. 1982. A comprehensive system of leaf analysis and its use for diagnosing crop nutrient status. Comm Soil Sci Plant Anal. 13:1035–1059. doi: 10.1080/00103628209367332
- Yamamoto S, Shiraishi S, Suzuki S. 2015. Are cyclic lipopeptides produced by Bacillus amyloliquefaciens S13-3 responsible for the plant defence response in strawberry against Colletotrichum gloeosporioides? Lett Appl Microbiol. 60:379–386. doi: 10.1111/lam.12382
- Yaqub M, Mahmood T, Akhtar M, Iqbal MM, Ali S. 2010. Induction of mungbean [Vigna radiate (L.) Wilczek] as a grain legume in the annual rice-wheat double cropping system. Pak J Bot. 42(5):3125–3135.
- Yu CW, Murphy TM, Lin CH. 2003. Hydrogen peroxide-induced chilling tolerance in mung beans mediated through ABA-independent glutathione accumulation. Funct Plant Biol. 30:955–963. doi: 10.1071/FP03091
- Zeng F, Qiu B, Ali S, Zhang G. 2010. Genotypic differences in nutrient uptake and accumulation in rice under chromium stress. J Plant Nutr. 33:518–528. doi: 10.1080/01904160903506258
- Zhang J, Cui Y, Duan C, Wu X, Wang X, Zhu Z 2009. Identification of pathogen causing soybean charcoal rot. Acta Agric Boreali Sin. 5:192–196.
- Zhifang G, Loescher WH. 2003. Expression of a celery mannose 6-phosphate reductase in Arabidopsis thaliana enhances salt tolerance and induces biosynthesis of both mannitol and a glucosyl-mannitol dimer. Plant Cell Environ. 26:275–283. doi: 10.1046/j.1365-3040.2003.00958.x