ABSTRACT
Both OFPs and ERECTA (ER) regulate plant response to environmental stresses as well as plant growth and development. Blunt-endeded siliques, the characterized phenotype of the er mutants, was observed in transgenic plants overexpressing Class III OFP genes, including OFP13, OFP15, OFP16 and OFP18. We found here that overexpression of ER restored the blunt-endeded siliques phenotypes observed in 35S:OFP15 or 35S:OFP16 plants, whereas er ofp15 and er ofp16 double mutants showed an overall phenotype similar to er single mutants, but their siliques were not blunt-endeded. By generating and characterizing transgenic plants overexpressing putative MAPK phosphorylation sites mutated OFP15 and OPF18, we found that phosphorylation status in Class III OFPs may affect their function in regulating plant growth and development. RT-PCR analysis showed that the expression levels of OFP15, OFP16 and OFP18 were increased in er mutants. Taken together, these results suggest that functions of Class III OFPs may depend on ER signaling.
Introduction
Ovate family proteins (OFPs) are characterized by their conserved C-terminal OVATE domain that consists of ∼70-aa (Liu et al. Citation2002, Citation2014; Wang et al. Citation2016). OFPs were first identified in tomato (Liu et al. Citation2002), and then were found distributed widely in plant kingdoms (Hackbusch et al. Citation2005; Gui and Wang Citation2007; Wang, Chang, et al. Citation2007, Citation2011, Citation2016; Rodriguez et al. Citation2011; Tsaballa et al. Citation2011; Huang et al. Citation2013; Liu et al. Citation2014; Wang et al. Citation2016). Genome-wide searching of available genome databases by using the amino acid sequences of OFPs in Arabidopsis and in tomato indicated that OFPs are presented in all the land plants examined including trees, crops, seedless vascular and non-vascular plants, but not in chlorophytes, a division of the green algae, suggesting that OFPs are plant-specific proteins (Liu et al. Citation2014).
OVATE is the first identified OFP, and it was identified as a key regulator of fruit shape in tomato (Liu et al. Citation2002). Since then, OFPs have been shown to regulate fruit shape in several different plants. As examples, pepper OFP CaOvate was found to regulate pepper fruit shape (Tsaballa et al. Citation2011), and several melon OFPs (CmOFPs) were found to co-mapped with several fruit shape controlling QTLs in melon, suggesting that CmOFPs may be involved in the regulation of melon fruit shape (Monforte et al. Citation2014). In addition, rice OFP1 (OsOFP1) was able to regulate rice grain shape, and two addition rice OFPs, OsOFP8 and OsOFP14 was found to interact with GS9, a rice grain shape regulator, indicating that they may also involve in the regulation of rice grain shape (Xiao et al. Citation2017; Zhao et al. Citation2018).
OFPs have also been shown to regulate several other aspects of plant growth and development. For example, Arabidopsis OFP1 and OFP4, and cotton OFP4 are able to regulate secondary cell wall formation (Li et al. Citation2011; Gong et al. Citation2014; Liu and Douglas Citation2015). Banana OFP1 (MaOFP1) is able to regulate postharvest banana fruit ripening (Liu, Zhang, et al. Citation2015). OsOFP1, OsOFP2, OsOFP6 and OsOFP19 are able to regulate plant architecture in rice (Schmitz et al. Citation2015; Ma et al. Citation2017; Xiao et al. Citation2017; Yang et al. Citation2018).
In addition to regulate plant growth and development, recent studies indicated that OFPs are also involved in the regulation of plant response to hormone signaling and environmental stresses. For example, OsOFP1, OsOFP8 and OsOFP19 have been shown to regulate brassinosteroid signaling in rice (Yang et al. Citation2016, Citation2018; Ma et al. Citation2017), OsOFP6 has been reported to regulate plant resistance to drought and cold stresses (Ma et al. Citation2017).
Available experimental evidence suggested that functions of OFPs in regulating plant growth and development, as well as plant response to hormone signaling and environmental stresses are likely achieved via transcriptional regulation of downstream genes, even though no DNA binding domain was identified in OFP proteins (Liu et al. Citation2002; Wang, Chang, et al. Citation2007, Citation2011, Citation2016; Liu, Zhang, et al. Citation2015, Citation2018; Yang et al. Citation2016, Citation2018; Ma et al. Citation2017; Xiao et al. Citation2017). Consistent with this, OFPs has been shown to interact with several different types of transcription factors. For instance, Arabidopsis OFPs, cotton OFP4 and OsOFP2 are able to interact with BELL and KNAT transcription factors (Hackbusch et al. Citation2005; Li et al. Citation2011; Gong et al. Citation2014; Liu and Douglas Citation2015; Schmitz et al. Citation2015; Zhang et al. Citation2016), banana OFP1 with a MADS transcription factor (Liu, Zhang, et al. Citation2015), and OsOFP8 and OsOFP14 with a novel transcription factor GS9 (Zhao et al. Citation2018). Yet the function mechanisms of OFPs remained largely unknown.
Although all single and double mutants of the Arabidopsis OFP genes are morphological similar to wild-type plants, transgenic plants overexpression of Arabidopsis OFP genes showed pleiotropic phenotypes (Wang et al. Citation2011). Based on the phenotypes in transgenic plants, Arabidopsis OFPs were classified into several different classes, and OFP13, OFP15, OFP16 and OFP18 belong to Classs III OFPs (Wang et al. Citation2011). Transgenic Arabidopsis overexpressing Class III OFP genes displayed blunt-ended siliques, a phenotype observed in the putative receptor protein kinase gene mutant erecta (er), and heterotrimeric G-protein β subunit gene mutant agb1-1 (Torii et al. Citation1996; Lease et al. Citation2001). AGB1 has been shown to function via an ER-type Leucine-rich repeat (LRR) receptor-like kinase signaling pathway to regulate silique morphology (Lease et al. Citation2001). It is likely that Class III OFPs may also regulate silique morphology via a similar signaling pathway.
ER LRR receptor-like kinases regulate plant response to abiotic stresses, as well as several different aspects of plant growth and development, such as stomata initiation and patterning, inflorescence morphology and ovate development, via mitogen-activated protein kinase (MAPK) signaling cascades (Torii et al. Citation1996; Shpak et al. Citation2003, Citation2004, Citation2005; Lee et al. Citation2012, Citation2015; Meng et al. Citation2012; Shen et al. Citation2015). Perception of extracellular signaling by ER LRR receptor-like kinases will result in phosphorylation and therefore activation of MAPK kinase kinases (MAPKKKs), phosphorylation signaling will then pass through MAPK kinases (MAPKKs) to MAPKs, whereas activated MAPKs are able to phosphorylate their downstream targets (MAPK Group Citation2002). Transcription factors are one of the several different types of phosphorylation targets of MAPKs, and phosphorylation status affects their functions (Mizoguchi et al. Citation1997; Jonak et al. Citation1999; Andreasson and Ellis Citation2010). As an example, SPEECHLESS has been shown to be a phosphorylation target of MAPKs, and phosphorylation status affects its function in regulating stomata development (Lampard et al. Citation2008).
Here we show that Class III AtOFPs may regulate plant growth and development via ER signaling pathway, that Class III AtOFPs may be phosphorylated by kinases downstream of the ER signaling pathway, and that phosphorylation status may affect the functions of Class III OFPs.
Materials and methods
Plant materials and growth conditions
Arabidopsis (Arabidopsis thaliana) ecotype Columbia-0 (Col) was used as wild type and for protoplasts isolation. The transgenic plants 35S:OFP15, 35S:OFP16 and 35S:OFP18, and the single mutants ofp15 and ofp16 have been described previously (Wang et al. Citation2011), the T-DNA insertion er mutant was obtained from the Arabidopsis Biological Resource Center (ABRC). All the transgenic plants and mutants are in Col background.
Double mutants er ofp15 and er ofp16 were generated by crossing er with ofp15 and ofp16 mutants respectively, and genotyping the corresponding F2 progeny obtained.
The 35S:ER/35S:OFP15 and 35S:ER/35S:OFP16 transgenic plants were generated by transformed 35S:OFP15 and 35S:OFP16 plants, respectively with PZP221-35S:ER construct, and select homozygous transgenic plants overexpressing ER.
The 35S:OFP15S216/219D, 35S:OFP15S216/219A, 35S:OFP18S70D and 35S:OFP18S70A transgenic plants were generated by transformed Col wild-type plants with corresponding constructs, and select homolzygous plants overexpressing mutated OFP15 or OFP18.
To obtain seedlings for DNA and RNA isolation, Arabidopsis seeds were surface sterilized and plated on plates containing 0.6% (w/v) phytoagar (PlantMedia) solidified ½ MS (Murashige & Skoog) with vitamins (PlantMedia) and 1% (w/v) sucrose. The plates were kept at 4°C in darkness for 2 days and transferred to a growth chamber. To obtain plants for phenotypic analysis, plant transformation, and protoplast isolation, Arabidopsis seeds were sown directly into soil pots. All the plants were grown in a growth chamber at 20°C with a long day photoperiod of 16 h/8 h (light/dark) at a light density of approximately 120 μmol m−2 s−1.
RNA isolation, RT-PCR
Total RNA were isolated from Arabidopsis seedlings as described before (Wang et al. Citation2014, Citation2015; Guo et al. Citation2015), and 2 μg total RNA was subjected to cDNA synthesis via Oligo (dT)-primer reverse transcription by using EasyScript First-Strand DNA Synthesis Super Mix (TransGen Biotech). RT-PCR was used to examine the expressing of OFP15, OFP16 and OFP18. The expression of ACTIN2 (ACT2) was used as a control for RT-PCR. The primers used have been described previously (Wang et al. Citation2011; Liu, Hu, et al. Citation2015).
Constructs
The reporter constructs LexA-Gal4:GUS and Gal4:GUS, and effector constructs GD, LD-VP, GD-OFP15, GD-OFP16 and GD-OFP18 used for protoplast transfection have been described preciously (Tiwari et al. Citation2004; Wang, Chang, et al. Citation2007, Citation2011).
To generate GD-OFP15VP, GD-OFP16VP and GD-OFP18VP constructs for protoplast transfection, the full-length OFP15, OFP16, and OFP18 coding regions without stop codons were amplified by PCR using plasmid DNA of GD-OFP15, GD-OFP16 or GD-OFP18 as templates, and cloned into the pUC19 vector in frame with an N-terminal GD tag and an C-terminal VP16 tag, under the control of the 35S promoter. The OFP15GFP, OFP16GFP and OFP18GFP constructs for protoplast transfection were generated by cloning the full-length OFP15, OFP16, and OFP18 coding regions without stop codons into the pUC19 vector in frame with a C-terminal GFP tag.
To generate OFP16VP and OFP18VP constructs for plant transformation, the GD tag in GD-OFP16VP and GD-OFP18VP was replaced by an HA tag, and the constructs in pUC19 were digested with proper enzymes and subcloned into the binary vector pPZP211 (Hajdukiewicz et al. Citation1994).
To generate ER construct for plant transformation, the full-length open reading frame of ER was amplified by RT-PCR using RNA isolated from Arabidopsis seedlings, and cloned into the pUC19 vector in frame with an N-terminal HA tag under the control of the 35S promoter. The construct was then digested with proper enzymes and subcloned into the binary vector pPZP221 (Hajdukiewicz et al. Citation1994).
The OFP15S216/219D, OFP15S216/219A, OFP18S70D and OFP18S70A constructs with a GD tag for protoplast transfection or an HA tag for plant transformation were generated by using a site-directed mutagenesis kit (TtansGen Biotech), and OFP15S216/219D and OFP15S216/219A constructs were obtained by two rounds of mutagenesis. The plasmid DNA of corresponding OFP15 and OFP18 constructs in pUC19 as templates. The point mutations in the constructs were confirmed by sequencing. The corresponding pUC19 constructs were then digested with proper enzymes and subcloned into the binary vector pPZP211 for plant transformation.
The primers used to generate OFP15S216/219D were S216D-F 5′-TAGTATGACTCTTGGCGAAGATCCATCGTCTCCC-3′, S216D-R 5′-TCTTCGCCAAGAGTCATACTATTACAACAAGAAGTGC-3′, S219D-F 5′-CTTGGCGAATCTCCATCGGATCCCTTGTCGTTTTAC-3′, and S219D-R 5′-TCCGATGGAGATTCGCCAAGAGTCATACTATTAC-3′. The primers used to generate OFP15S216/219A were S216A-F 5′-TAGTATGTCTGTTGGCGAAGCTCCATCGTCTCCC-3′, S216A-R 5′-CTTCGCCAAGAGTCATACTATTACTTATTGTTGGTGC-3′, S219A-F 5′-CTTGGCGAATCTCCATCGGCTCCCTTGTCGTTTTAC-3′, and S219A-R 5′-CCGATGGAGATTCGCCAAGAGTCATACTATTAC-3′. The primers used to generate OFP18S70D were S70D-F 5′-CACCGAGAAGCTTCTCCTCCGACCCCTCCTCC-3′ and S70D-R 5′-TCGGAGGAGAAGCTTCTCGGTGGTGGCTCGGG-3′. The primers used to generate OFP18S70A were S70A-F 5′-CGAAGAAGCTTCTCCTCCGCCCCCTCCTC-3′ and S70A-R 5′-CGGAGGAGAAGCTTCTCGGTGGTGGCTC-3′.
Plasmid DNA max preparation, protoplast isolation and transfection
Reporter and effector plasmids DNA were isolated by using a GoldHi EndoFree Plasmid Maxi Kit (Kangwei) by following the manufacture’s protocols. Arabidopsis protoplasts were isolated from rosette leaves of ∼4-week-old Col wild-type plants and transfected with plasmid DNA by following the procedures described previously (Wang et al. Citation2005, Citation2015; Dai et al. Citation2016). For GUS activity assays, plasmid DNA of corresponding reporter and effectors was co-transfected into protoplasts. For GFP fluorescence observation, plasmid DNA of the corresponding effector was transfected into protoplasts. The protoplasts were incubated at room temperature and under darkness for 20–22 h before GUS activity assay or GFP fluorescence observation.
GUS activity assays
GUS activities in transfected protoplasts were assayed as described previously (Wang et al. Citation2005), by using a SynergyTM HT fluorescence microplate reader (BioTEK).
Microscopy
Photographs of the Col wild type, transgenic and mutant plants were taken using an EOS 1100D digital camera. GFP fluorescence in transfected protoplasts was examined and photographed under an Olympus FV1000 confocal microscope.
Plant transformation and transgenic plants selection
Col wild-type plants or transgenic plants overexpressing OFP15 or OFP16 were used for plant transformation. Plants about ∼5-week-old with several mature flowers on the main inflorescence were transformed with corresponding constructs in Agrobacterium tumefaciens (strain GV3101) by using the floral dip method (Clough and Bent Citation1998). T1 seeds were collected and transgenic plants were selected by grown T1 seeds on 1/2 MS plates containing carbenicillin and kanamycin for plants transformed with PZP211 vectors, and carbenicillin and gentamycin for plants transformed with PZP221 vectors. Multiple transgenic lines were obtained. Phenotypes of the transgenic plants were examined in the T1 generation and confirmed in T2 up to T4 generations. For each construct, at least five independent overexpressing lines with similar phenotypes were obtained, and one or two homozygous lines were selected for phenotypic examination and photographing by growing side by side with corresponding control plants.
Results
ER and Class III OFPs function in the same pathway to regulate plant growth and development
Previously we have shown that overexpressing Class III OFP genes resulted in pleiotropic phenotypes including smaller rosette size, later flowering, reduced fertilization and blunt-ended siliques (Wang et al. Citation2011), and some of the phenotypes such as small rosette size and blunt-ended siliques are characterized phenotypes of the er mutants (Lease et al. Citation2001), indicating that ER and Class III OFPs may function in the same signaling pathway to regulate plants growth and development. To examine if that is the case, we first examined whether overexpression of ER may affect the phenotypes observed in the transgenic overexpressing Class III OFP genes. To do that, we generated 35S:ER transgenic plants in the 35S:OFP15 and 35S:OFP16 transgenic plant backgrounds, respectively. We found that blunt-ended silique phenotypes observed in the 35S:OFP15 and 35S:OFP16 transgenic plants were recovered by overexpressing ER (), and the overall morphology of the 35S:ER/35S:OFP15 and 35S:ER/35S:OFP16 transgenic plants was also similar to that of the wild-type plants ().
Figure 1. Phenotypes of 35S:ER/35S:OFP15 and 35S:ER/35S:OFP16 transgenic plants. Lower, morphology of the Col wild type, er mutant, 35S:OFP15, 35S:OFP16, 35S:ER/35S:OFP15 and 35S:ER/35S:OFP16 transgenic plants. Upper, close view of the silique end of the fourth silique from main inflorescence of Col wild type, er mutant, 35S:OFP15, 35S:OFP16, 35S:ER/35S:OFP15 and 35S:ER/35S:OFP16 transgenic plants. All the plants were grown side by side in soil pots. Plants ∼7-week-old were photographed by using a digital camera.
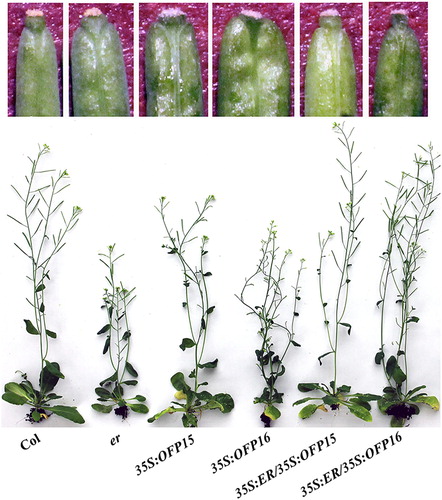
We then examined if loss-of-function of Class III OFP genes may affect the phenotypes observed in er mutants by generating er ofp15 and er ofp16 double mutants. We found that similar to er mutants, the er ofp15 and er ofp16 double mutants produced smaller rosette size ((A)). On the other hand, the siliques of the er ofp15 and er ofp16 double mutants were still shorter when compared to that in the wild-type plants, but no longer blunt-ended ((B)).
Figure 2. Phenotypes of er ofp15 and er ofp16 double mutants. (A) Morphology of the Col wild type, er, ofp15 and ofp16 single mutants, and er ofp15 and er ofp16 double mutants. All the plants were grown side by side in soil pots. Plants ∼4-week-old were photographed by using a digital camera. (B) The fourth silique from main inflorescence of Col wild type, er, ofp15 and ofp16 single mutants, and er ofp15 and er ofp16 double mutants. All the plants were grown side by side in soil pots. Morphology of siliques in ∼7-week-old plants were observed and photographed by using a digital camera.
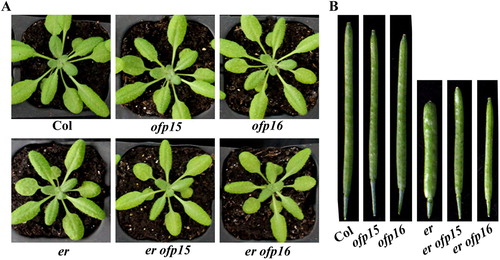
We examined whether the blunt-ended silique phenotypes observed in the er mutants may due to altered expression levels of Class III OFP genes. Total RNA was isolated from seedlings of Col wild type and er mutant, and RT-PCR was used to expression levels of Class III OFP genes. As shown in , the expression level of OFP15, OFP16 and OFP18 increased in the er mutant seedlings.
Class III OFPs function as transcription factors in regulating plant growth and development
ER has been shown to regulate plant growth and development through mitogen-activated protein kinase (MAPK) cascades (Torii et al. Citation1996; Shpak et al. Citation2003, Citation2004, Citation2005; Lee et al. Citation2012; Meng et al. Citation2012), whereas transcription factors are among the phosphorylation targets of MAPKs (Mizoguchi et al. Citation1997; Jonak et al. Citation1999; Andreasson and Ellis Citation2010). We therefore wanted to examine if the phosphorylation status of Class III OFPs may affect their ability in regulating plant growth and development.
To do that, we first wanted to examine whether the functions of Class III OFPs in regulating plant growth and development were due to their transcriptional activities. Because even though all OFPs repressed reporter gene expression in transfected protoplasts (Wang et al. Citation2011), it has been shown that OFP1 is involved in the regulation of DNA double-stand break repair (Wang et al. Citation2010). We examined the subcellular localization of OFP15, OFP16 and OFP18 by using GFP fusion constructs in transfected protoplasts, and found that GFP inflorescence were observed mainly in the nucleus ((A)). Because transcription factor regulates plants growth and development by regulating the expression of down stream target genes, changes in their transcription activities will result in altered effects on plant growth and development. We examine if any of the Class III OFPs can be turned from transcriptional repressors to activators by fusing a VP16 strong activator. As shown in (B), when co-transfected with reporter gene into protoplasts, OFP18VP activated the Gal4:GUS reporter gene expression, whereas OFP15VP and OFP16VP failed to do so. We then generated transgenic plants overexpressing OFP18VP, we found that 35S:OFP18VP transgenic plants did not produce pleiotropic phenotypes observed in the 35S:OFP18 transgenic plants ((C)). As a control, we also generated transgenic plants overexpressing OFP16VP, and we found that pleiotropic phenotypes similar to that in the 35S:OFP16 transgenic plants were still observed in 35S:OFP16VP plants ((C)).
Figure 4. Effects of transcriptional activator VP on the functions of Class III OFPs. (A) Subcellular localization of Class III OFP proteins. Plasmid DNA of corresponding OFP effector was transfected into protoplasts, the transfected protoplasts were incubated at room temperature under darkness for 20–22 h, and then GFP fluorescence was examined and photographed under an Olympus FV1000 confocal microscope. (B) Transcriptional activities of VP fused Class III OFP transcription factors. Plasmid DNA of the Gal4:GUS reporter and corresponding OFP effector was co-transfected into protoplasts, the transfected protoplasts were incubated at room temperature under darkness for 20–22 h, and then GUS activity was measured by using a SynergyTM HT fluorescence microplate reader (BioTEK). Data represent the mean ± SD of three repeats. The experiment was repeated three times with similar results. (C) Phenotypes of 35S:OFP16VP and 35S:OFP18VP transgenic plants. All the plants were grown side by side in soil pots. Plants ∼3-week-old were photographed by using a digital camera.
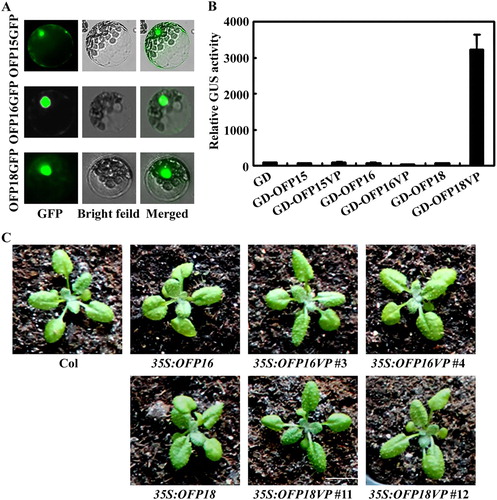
Phosphorylation status has little, if any effected on transcriptional activities of Class III OFPs
In the MAPK signaling cascades, the MAPKs are Ser/Thr protein kinases, and activated MAPKs can either remained in cytosol to phosphorylate cytoskeleton proteins and protein kinases in cytosol, or enter into nucleus to phosphorylate transcription factors (Mizoguchi et al. Citation1997; Jonak et al. Citation1999; Bethke et al. Citation2009; Andreasson and Ellis Citation2010). Several transcription factors such as WRKY22, WRKY29, WRKY33, EIN3, ERF104 and SPCH have been shown to be substrates of MAPKs, and the phosphorylation sites in these transcription factors including PXS/TP consensus motif and low stringency SP motif (Asai et al. Citation2002; Andreasson et al. Citation2005; Lampard et al. Citation2008; Yoo et al. Citation2008; Andreasson and Ellis Citation2010), By examined the amino acid sequence, we found that there are two potential MAPK phosphorylation sites in OFP15, a low stringency SP motif at S216 and a PXS/TP consensus motif at S219, and only one in OFP18, a low stringency SP motif at S70 ((A)). All of the three potential MAPK phosphorylation sites have high scores according to GPS phosphorylation sites prediction (http://gps.biocuckoo.org/), 11.41 and 24.986 for S216 and S219, respectively in OFP15, and 30.118 for S70 in OFP18. We therefore used OFP15 and OFP18 to examine the effects of phosphorylation status on the functions of Class III OFPs.
Figure 5. Effects of amino acid substitution of the putative MAPK phosphorylation sites on the transcriptional activity of OFP15 and OFP18. (A) Putative MAPK phosphorylation sites in OFP15 and OFP18. Stars indicate the putative phosphorylation sites. (B) Transcriptional activities of putative phosphorylation sites substituted OFP15 and OFP18. Plasmid DNA of the LexA-Gal4:GUS reporter, transcription activator LD-VP and corresponding OFP effector was co-transfected into protoplasts, the transfected protoplasts were incubated at room temperature under darkness for 20–22 h, and then GUS activity was measured by using a SynergyTM HT fluorescence microplate reader (BioTEK). Data represent the mean ± SD of three repeats. The experiment was repeated three times with similar results.
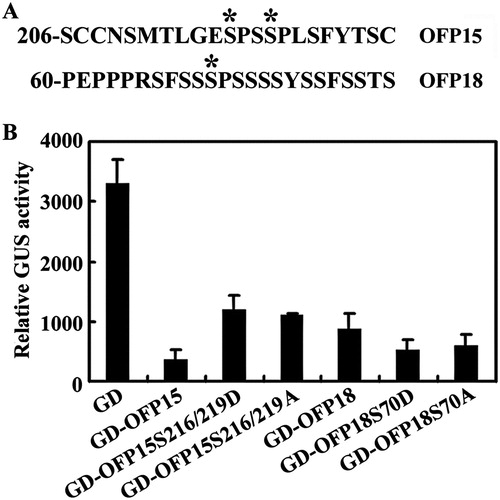
We generated two different mutant forms of OFP15 and OFP18 by site-directed mutagenesis, the phospho-mimic mutant form by mutating S residue to D, the nonphosphorylatable mutant form by mutating S residue to A. We then examined the transcription activities of the mutated forms of OFP15 and OFP18 by using protoplast transfection assays. In this system, the LexA-Gal4:GUS reporter gene was activated by transcriptional activator LD-VP, and co-transfection of transcription repressors will result in inhibition of reporter gene expression. As shown in (B), co-transfection of GD-fused OFP15 or OFP18 repressed reporter gene expression, a result similar to reported previously (Wang et al. Citation2011). Co-transfection of GD-fused mutated forms of OFP15 or OFP18 also resulted in repression of the reporter gene ((B)), suggesting the mutated forms of OFP15 and OFP18 were still functioned as transcriptional repressors.
Phosphorylation status affected Class III OFPs’ function in regulating plant growth and development
Phosphorylation of transcription factors has been shown to affect their function in plants, and transgenic plants expressing phospho-mimic and/or nonphosphorylatable mutant genes have been used to study the effects of phosphorylation on the functions of transcription factor (Andreasson and Ellis Citation2010). To examine the effects of phosphorylation on the functions of Class III OFPs, we generated transgenic plants overexpressing phospho-mimic as well as nonphosphorylatable OFP15 and OFP18 genes, respectively, and examined phenotypes of the transgenic plants generated.
As shown in (A), 35S:OFP15S216/219D transgenic plants showed a similar but more severe, whereas 35S:OFP15S216/219A transgenic plants showed a less severe phenotype when compared with that in the 35S:OFP15 transgenic plants. Significantly morphology different was also observed in siliques. The siliques in 35S:OFP15S216/219D transgenic plants were very short, and could only produce a few seeds, whereas that in the 35S:OFP15S216/219A transgenic plants were longer, although were still shorter when compared with that in the 35S:OFP15 transgenic plants, and no longer have blunt-ended phenotypes ((B)). Similar phenotype changes were observed in the 35S:OFP18S70D and 35S:OFP18S70A transgenic plants ().
Figure 6. Phenotypes of transgenic plants overexpressing phosphorylation sites substituted OFP15. (A) Morphology of the Col wild type, 35S:OFP15, 35S:OFP15S216/219D and 35S:OFP15S216/219A transgenic plants. All the plants were grown side by side in soil pots. Plants ∼4-week-old were photographed by using a digital camera. (B) The fourth silique from the main inflorescence of the Col wild type, 35S:OFP15, 35S:OFP15S216/219D and 35S:OFP15S216/219A transgenic plants. All the plants were grown side by side in soil pots, the morphology of siliques in ∼7-week-old plants was observed and photographed by using a digital camera.
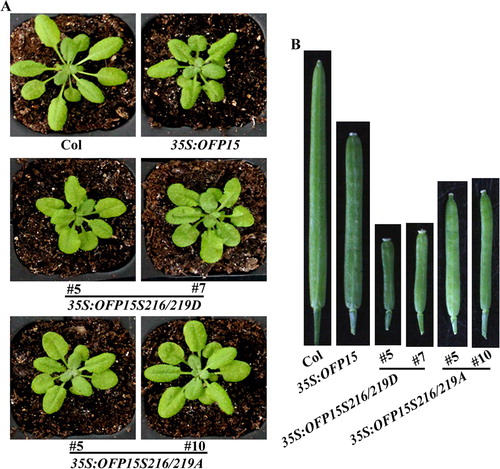
Figure 7. Phenotypes of transgenic plants overexpressing phosphorylation sites substituted OFP18. (A) Morphology of the Col wild type, 35S:OFP18, 35S:OFP18S70D and 35S:OFP18S70A transgenic plants. All the plants were grown side by side in soil pots. Plants ∼4-week-old were photographed by using a digital camera. (B) The fourth silique from the main inflorescence of the Col wild type, 35S:OFP18, 35S:OFP18S70D and 35S:OFP18S70A transgenic plants. All the plants were grown side by side in soil pots, the morphology of siliques in ∼7-week-old plants was observed and photographed by using a digital camera.
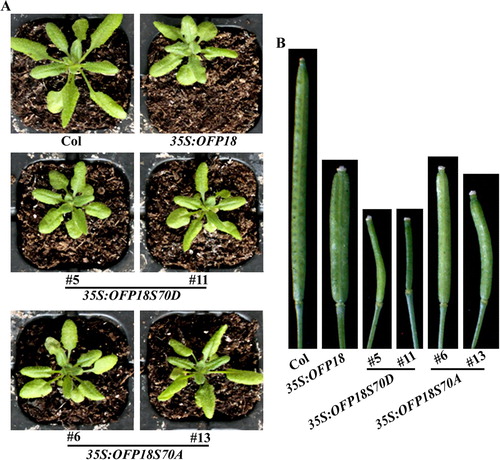
Discussion
Previously we showed that overexpression Class III OFP genes including OFP13, OFP15, OFP16 and OFP18 resulted in blunt-ended siliques, a phenotype similar to that in er mutants (Torii et al. Citation1996; Lease et al. Citation2001; Wang et al. Citation2011). We provide evidence here that phosphorylation status affects the function of Calss III OFPs, and its very likely that Class III OFPs function in the ER signaling pathway to regulate plant growth and development.
Three different lines of evidence indicate that Class III OFPs and ER function in the same signaling pathway to regulate plant growth and development. First, the overall phenotypes in the 35S:OFP15 and 35S:OFP16 transgenic plants were restored by overexpressing ER (). Second, although ofp15 and ofp16 mutants were morphologically similar to wild-type plants (Wang et al. Citation2011), blunt-ended silique phenotype in er mutants was no longer observed in er ofp15 and er ofp16 double mutants (). Third, the expression levels of OFP15, OFP16 and OFP18 were elevated in er mutants (). However, we also note that the rosettle size and the silique length in er ofp15 and er ofp16 double mutants were similar to that in the er mutants (). These results suggest that ER may regulate plant morphology via Class III OFPs, as well as some other regulators, which is consistent with the fact that ER regulate several different aspects of plant growth and development (Torii et al. Citation1996; Shpak et al. Citation2003, Citation2004, Citation2005; Lee et al. Citation2012, Citation2015; Meng et al. Citation2012). Considering that Class III OFPs may have redundant functions in regulating plant growth and development (Wang et al. Citation2011), generation and characterization of high order mutants of Class III OFP genes in both Col wild type and er mutant backgrounds may provide further information into the relationship between Class III OFPs and ER in the regulation of plant growth and development.
ER regulates several different aspects of plant growth and development via MAPK signaling cascades (Torii et al. Citation1996; Shpak et al. Citation2003, Citation2004, Citation2005; Lee et al. Citation2012; Citation2015; Meng et al. Citation2012). Transcription factors are one of the several different types of phosphorylation targets of MAPKs (Mizoguchi et al. Citation1997; Jonak et al. Citation1999; Andreasson and Ellis Citation2010), and consensus motif PXS/TP and low stringency motif SP have been shown to be the phosphorylation sites in these transcription factors (Asai et al. Citation2002; Andreasson et al. Citation2005; Lampard et al. Citation2008; Yoo et al. Citation2008; Andreasson and Ellis Citation2010). Amino acid substitution of S/T residue with nonphosphorylatable residue A or acidic residues D or E can create nonphosphorylatable and phosphor-mimic variants respectively, which have been used to probe the effects of phosphorylation on the transcription factors (Asai et al. Citation2002; Andreasson et al. Citation2005; Lampard et al. Citation2008; Yoo et al. Citation2008; Andreasson and Ellis Citation2010).
We found there are two and one phosphorylation sites respectively, in OFP15 and OFP18 (). By examining transcription activities of nonphosphorylatable and phosphor-mimic variants of OFP15 and OFP18 in transformed protoplasts, we found that these variants were still be able to repress the expression of the reporter gene (). However, when compared with 35S:OFP15 and 35S:OFP18 transgenic plants respectively, more severe pleitropic phenotypes were observed in transgenic plants overexpressing phosphor-mimic OFP15 and OFP18 genes, but less severe in the transgenic plants overexpressing nonphosphorylatable OFP15 and OFP18 genes ( and ). These results suggest that phosphorylation status affected Class III OFPs’ function in the regulation of plant growth and development.
ER is involved in the regulation of several different aspects of plant growth and development including stomata initiation and inflorescence morphology. All the components in the regulation of stomata development have been identified, they are the ER upstream components peptide hormone EPF1 and EPF2 (Hara et al. Citation2007; Hunt and Gray Citation2009; Lee et al. Citation2012, Citation2015), the ER downstream components MAPKKK protein YODA, MPKK protein MKK4 and MKK5, MAPK protein MPK3 and MPK6, and the transcription factor SPEECHLESS (Wang, Ngwenyama, et al. Citation2007; Lampard et al. Citation2008; Meng et al. Citation2012). Inflorescence morphology is regulated by the same singling pathway except that EPF1 and EPF2 were replaced by EPFL4 and EPFL6, and the transcription factors downstream of MAPKs have not been identified (Abrash et al. Citation2011; Meng et al. Citation2012; Uchida et al. Citation2012). Considering that Class III OFPs regulate silique morphology in Arabidopsis (, Wang et al. Citation2011), it will be of great interesting to examine whether Class III OFPs may be the targets of MPK3 and MPK6. Alternatively, identification of the MAPKs that may target Class III OFPs may help further elusive the mechanisms under the regulation of plant growth and development.
In addition to regulation plant growth and development, both ER and OFPs have also been reported to regulate plant responses to environment stresses. Loss-of-function or reduced expression of ER in Arabidopsis, rice and tomato resulted in heat sensitivity, whereas overexpression of Arabidopsis ER in rice and tomato enhanced heat tolerance (Shen et al. Citation2015). On the other hand, rice OFP6 has been shown to regulate plant resistance to drought and cold (Ma et al. Citation2017). Arabidopsis OFP8 has recently been shown to regulate epicuticular waxes accumulation (Tang et al. Citation2018), a character related to water loss. Our results indicate that Class III OFPs function in the ER signaling pathway to regulate plants growth and development in Arabidopsis. It is very likely that Class III OFPs may also integrate with ER signaling to regulate plant response to environmental stresses. It will be of great interesting to examine if that is the case.
Acknowledgments
We thank Dr. Tianya Wang and all the lab members for helpful discussion. The funders had no role in study design, data collection and analysis, decision to publish, or preparation of the manuscript.
Disclosure statement
No potential conflict of interest was reported by the authors.
ORCID
Shucai Wang http://orcid.org/0000-0001-7619-2385
Additional information
Funding
References
- Abrash EB, Davies KA, Bergmann DC. 2011. Generation of signaling specificity in Arabidopsis by spatially restricted buffering of ligand-receptor interactions. Plant Cell. 23:2864–2879. doi: 10.1105/tpc.111.086637
- Andreasson E, Ellis B. 2010. Convergence and specificity in the Arabidopsis MAPK nexus. Trends Plant Sci. 15:106–113. doi: 10.1016/j.tplants.2009.12.001
- Andreasson E, Jenkins T, Brodersen P, Thorgrimsen S, Petersen NH, Zhu S, Qiu JL, Micheelsen P, Rocher A, Petersen M, et al. 2005. The MAP kinase substrate MKS1 is a regulator of plant defense responses. EMBO J. 24:2579–2589. doi: 10.1038/sj.emboj.7600737
- Asai T, Tena G, Plotnikova J, Willmann MR, Chiu WL, Gomez-Gomez L, Boller T, Ausubel FM, Sheen J. 2002. MAP kinase signaling cascade in Arabidopsis innate immunity. Nature. 415:977–983. doi: 10.1038/415977a
- Bethke G, Unthan T, Uhrig JF, Pöschl Y, Gust AA, Scheel D, Lee J. 2009. Flg22 regulates the release of an ethylene response factor substrate from MAP kinase 6 in Arabidopsis thaliana via ethylene signaling. Proc Nat. Acad Sci USA. 106:8067–8072. doi: 10.1073/pnas.0810206106
- Clough SJ, Bent AF. 1998. Floral dip: a simplified method for Agrobacterium-mediated transformation of Arabidopsis thaliana. Plant J. 16:735–743. doi: 10.1046/j.1365-313x.1998.00343.x
- Dai X, Zhou L, Zhang W, Cai L, Guo H, Tian H, Schiefelbein J, Wang S. 2016. A single amino acid substitution in the R3 domain of GLABRA1 leads to inhibition of trichome formation in Arabidopsis without affecting its interaction with GLABRA3. Plant Cell Environ. 39:897–907. doi: 10.1111/pce.12695
- Gong SY, Huang GQ, Sun X, Qin LX, Li Y, Zhou L, Li XB. 2014. Cotton KNL1, encoding a class II KNOX transcription factor, is involved in regulation of fibre development. J Exp Bot. 65:4133–4147. doi: 10.1093/jxb/eru182
- Gui B, Wang Y. 2007. Cloning and sequence analysis of ovate Orthologous gene in Tobacco (Nicotiana tabacum L.). Plant Physiol Commu. 43:1050–1056.
- Guo H, Zhang W, Tian H, Zheng K, Dai X, Liu S, Hu Q, Wang X, Liu B, Wang S. 2015. An auxin responsive CLE gene regulates shoot apical meristem development in Arabidopsis. Front Plant Sci. 6:295.
- Hackbusch J, Richter K, Müller J, Salamini F, Uhrig JF. 2005. A central role of Arabidopsis thaliana ovate family proteins in networking and subcellular localization of 3-aa loop extension homeodomain proteins. Proc Natl Acad Sci USA. 102:4908–4912. doi: 10.1073/pnas.0501181102
- Hajdukiewicz P, Svab Z, Maliga P. 1994. The small, versatile pPZP family of Agrobacterium binary vectors for plant transformation. Plant Mol Biol. 25:989–994. doi: 10.1007/BF00014672
- Hara K, Kajita R, Torii KU, Bergmann DC, Kakimoto T. 2007. The secretory peptide gene EPF1 enforces the stomatal one-cell-spacing rule. Genes Dev. 21:1720–1725. doi: 10.1101/gad.1550707
- Huang ZJ, Van Houten J, Gonzalez G, Xiao H, van der Knaap E. (2013). Genome-wide identification, phylogeny and expression analysis of SUN, OFP and YABBY gene family in tomato. Mol Genet Genomics. 288:111–129. doi: 10.1007/s00438-013-0733-0
- Hunt L, Gray JE. 2009. The signaling peptide EPF2 controls asymmetric cell divisions during stomatal development. Curr Biol. 19:864–869. doi: 10.1016/j.cub.2009.03.069
- Jonak C, Ligterink W, Hirt H. 1999. MAP kinases in plant signal transduction. Cellul Mol Life Sci. 55:204–213. doi: 10.1007/s000180050285
- Lampard GR, Macalister CA, Bergmann DC. 2008. Arabidopsis stomatal initiation is controlled by MAPK-mediated regulation of the bHLH SPEECHLESS. Science. 322:1113–1116. doi: 10.1126/science.1162263
- Lease KA, Wen J, Li J, Doke JT, Liscum E, Walker JC. 2001. A mutant Arabidopsis heterotrimeric G-protein b subunitaffects leaf, flower, and fruit development. Plant Cell. 13:2631–2641.
- Lee JS, Hnilova M, Maes M, Lin YC, Putarjunan A, Han SK, Avila J, Torii KU. 2015. Competitive binding of antagonistic peptides fins-turns stomatal patterning. Nature. 522:439–443. doi: 10.1038/nature14561
- Lee JS, Kuroha T, Hnilova M, Khatayevich D, Kanaoka MM, McAbee JM, Sarikaya M, Tamerler C, Torii KU. 2012. Direct interaction of ligand-receptor pairs specifying stomatal patterning. Genes Dev. 26:126–136. doi: 10.1101/gad.179895.111
- Li E, Wang S, Liu Y, Chen JG, Douglas CJ. 2011. Ovate family protein (OFP4) interaction with KNAT7 regulates secondary cell wall formation in Arabidopsis thaliana. Plant J. 67:328–341. doi: 10.1111/j.1365-313X.2011.04595.x
- Liu Y, Douglas CJ. 2015. A role for OVATE FAMILY PROTEIN1 (OFP1) and OFP4 in a BLH6-KNAT7 multi-protein complex regulating secondary cell wall formation in Arabidopsis thaliana. Plant Signal Behav. 10:e1033126.
- Liu S, Hu Q, Luo S, Li Q, Yang X, Wang X, Wang S. 2015. Expression of wild-type PtrIAA14.1, a poplar Aux/IAA gene causes morphological changes in Arabidopsis. Front Plant Sci. 6:388.
- Liu D, Sun W, Yuan Y, Zhang N, Hayward A, Liu Y, Wang Y. 2014. Phylogenetic analyses provide the first insights into the evolution of OVATE family proteins in land plants. Ann Bot. 113:1219–1233. doi: 10.1093/aob/mcu061
- Liu J, Van Eck J, Cong B, Tanksley SD. 2002. A new class of regulatory genes underlying the cause of pear-shaped tomato fruit. Proc Natl Acad Sci USA. 99:13302–13306. doi: 10.1073/pnas.162485999
- Liu J, Zhang J, Hu W, Miao H, Zhang J, Jia C, Wang Z, Xu B, Jin Z. 2015. Banana ovate family protein MaOFP1 and MADS-box protein MuMADS1 antagonistically regulated banana fruit ripening. PLoS One. 10:e0123870. doi: 10.1371/journal.pone.0123870
- Liu J, Zhang J, Wang J, Zhang J, Miao H, Jia C, Wang Z, Xu B, Jin Z. 2018. MuMADS1 and MaOFP1 regulate fruit quality in a tomato ovate mutant. Plant Biotechnol J. 16:989–1001. doi: 10.1111/pbi.12843
- Ma Y, Yang C, He Y, Tian Z, Li J. 2017. Rice OVATE family protein 6 regulates plant development and confers resistance to drought and cold stresses. J Exp Bot. 68:4885–4898. doi: 10.1093/jxb/erx309
- MAPK group. 2002. Mitogen-activated protein kinase cascades in plants: a new nomenclature. Trends in Plant Sci. 7:301–308. doi: 10.1016/S1360-1385(02)02302-6
- Meng X, Wang H, He Y, Liu Y, Walker JC, Torii KU, Zhang S. 2012. A MAPK Cascade downstream of ERECTA receptor-like protein kinase regulates Arabidopsis inflorescence architecture by promoting localized cell proliferation. Plant Cell. 24:4948–4960. doi: 10.1105/tpc.112.104695
- Mizoguchi T, Ichinura K, Shinozaki K. 1997. Evironmental stress response in plants: the role of mitogen-activated protein kinases. Trends Biotech. 15:15–19. doi: 10.1016/S0167-7799(96)10074-3
- Monforte AJ, Diaz A, Caño-Delgado A, van der Knaap E. 2014. The genetic basis of fruit morphology in horticultural crops: lessons from tomato and melon. J Exp Bot. 65:4625–4637. doi: 10.1093/jxb/eru017
- Rodríguez GR, Muños S, Anderson C, Sim SC, Michel A, Causse M, Gardener BB, Francis D, van der Knaap E. (2011). Distribution of SUN, OVATE, LC, and FAS in the tomato germplasm and the relationship to fruit shape diversity. Plant Physiol. 156:275–285. doi: 10.1104/pp.110.167577
- Schmitz AJ, Begcy K, Sarat GH, Walia H. 2015. Rice ovate family protein 2 (OFP2) alters hormonal homeostasis and vasculature development. Plant Sci. 241:177–188. doi: 10.1016/j.plantsci.2015.10.011
- Shen H, Zhong X, Zhao F, Wang Y, Yan B, Li Q, Chen G, Mao B, Wang J, Li Y, et al. 2015. Overexpression of receptor-like kinase ERECTA improves thermotolerance in rice and tomato. Nat Biotechnol. 33:996–1003. doi: 10.1038/nbt.3321
- Shpak ED, Berthiaume CT, Hill EJ, Torii KU. 2004. Synergistic interaction of three ERECTA-family receptor-like kinases controls Arabidopsis organ growth and flower development by promoting cell proliferation. Development. 131:1491–1501. doi: 10.1242/dev.01028
- Shpak ED, Lakeman MB, Torii KU. 2003. Dominantnegative receptor uncovers redundancy in the Arabidopsis ERECTA Leucine-rich repeat receptor-like kinase signaling pathway that regulates organ shape. Plant Cell. 15:1095–1110. doi: 10.1105/tpc.010413
- Shpak ED, McAbee JM, Pillitteri LJ, Torii KU. 2005. Stomatal patterning and differentiation by synergistic interactions of receptor kinases. Science. 309:290–293. doi: 10.1126/science.1109710
- Tang Y, Zhang W, Yin YL, Feng P, Li HL, Chang Y. 2018. Expression of ovate family protein 8 affects epicuticular waxes accumulation in Arabidopsis thaliana. Bot Stud. 59:12. doi: 10.1186/s40529-018-0228-8
- Tiwari SB, Hagen G, Guilfoyle TJ. 2004. Auxin/IAA proteins contain a potent transcriptional repression domain. Plant Cell. 16:533–543. doi: 10.1105/tpc.017384
- Torii KU, Mitsukawa N, Oosumi T, Matsuura Y, Yokoyama R, Whittier RF, Komeda Y. 1996. The Arabidopsis ERECTA gene encodes a putative receptor protein kinase with extracellular leucine-rich repeats. Plant Cell. 8:735–746.
- Tsaballa A, Pasentsis K, Darzentas N, Tsaftaris AS. 2011. Multiple evidence for the role of an ovate-like gene in determining fruit shape in pepper. BMC Plant Biol. 11:46. doi: 10.1186/1471-2229-11-46
- Uchida N, Lee JS, Horst RJ, Lai HH, Kajita R, Kakimoto T, Tasaka M, Torii KU. 2012. Regulation of inflorescence architecture by intertissue layer ligand-receptor communication between endodermis and phloem. Proc Natl Acad Sci USA. 109:6337–6342. doi: 10.1073/pnas.1117537109
- Wang S, Chang Y, Ellis B. 2016. Overview of ovate family proteins, a novel class of plant-specific growth regulators. Front Plant Sci. 7:417.
- Wang S, Chang Y, Guo J, Chen JG. 2007. Arabidopsis ovate family protein 1 is a transcriptional repressor that suppresses cell elongation. Plant J. 50:858–872. doi: 10.1111/j.1365-313X.2007.03096.x
- Wang S, Chang Y, Guo J, Zeng Q, Ellis BE, Chen JG. 2011. Arabidopsis ovate family proteins, a novel transcription factor family, control multiple aspects of plant growth and development. PLoS ONE. 6:e23896. doi: 10.1371/journal.pone.0023896
- Wang S, Li E, Porth I, Chen JG, Mansfield SD, Douglas CJ. 2014. Regulation of secondary cell wall biosynthesis by poplar R2R3 MYB transcription factor PtrMYB152 in Arabidopsis. Sci Rep. 4:5054. doi: 10.1038/srep05054
- Wang YK, Liu PF, Hsiao MK, Lin CT, Lin SM, Pan RL. 2010. Ovate family protein 1 as a plant Ku70 interacting protein involving in DNA double-strand break repair. Plant Mol Biol. 74:453–466. doi: 10.1007/s11103-010-9685-5
- Wang H, Ngwenyama N, Liu Y, Walker JC, Zhang S. 2007. Stomatal development and patterning are regulated by environmentally responsive mitogen-activated protein kinases in Arabidopsis. Plant Cell. 19:63–73. doi: 10.1105/tpc.106.048298
- Wang S, Tiwari SB, Hagen G, Guilfoyle TJ. 2005. Auxin response factor7 restores the expression of auxin-responsive genes in mutant Arabidopsis leaf mesophyll protoplasts. Plant Cell. 17:1979–1993. doi: 10.1105/tpc.105.031096
- Wang X, Wang X, Hu Q, Dai X, Tian H, Zheng K, Wang X, Mao T, Chen JG, Wang S. 2015. Characterization of an activation-tagged mutant uncovers a role of GLABRA2 in anthocyanin biosynthesis in Arabidopsis. Plant J. 83:300–311. doi: 10.1111/tpj.12887
- Xiao Y, Liu D, Zhang G, Tong H, Chu C. 2017. Brassinosteroids regulate OFP1, a DLT interacting protein, to modulate plant architecture and grain morphology in rice. Front Plant Sci. 8:1698. doi: 10.3389/fpls.2017.01698
- Yang C, Ma Y, He Y, Tian Z, Li J. 2018. OsOFP19 modulates plant architecture by integrating the cell division pattern and brassinosteroid signaling. Plant J. 93:489–501. doi: 10.1111/tpj.13793
- Yang C, Shen W, He Y, Tian Z, Li J. 2016. OVATE family protein 8 positively mediates brassinosteroid signaling through interacting with the GSK3-like kinase in rice. PLoS Genet. 12:e1006118. doi: 10.1371/journal.pgen.1006118
- Yoo SD, Cho YH, Tena G, Xiong Y, Sheen J. 2008. Dual control of nuclear EIN3 by bifurcate MAPK cascades in C2H4 signalling. Nature. 451:789–795. doi: 10.1038/nature06543
- Zhang L, Zhang X, Ju H, Chen J, Wang S, Wang H, Zhao Y, Chang Y. 2016. Ovate family protein1 interaction with BLH3 regulates transition timing from vegetative to reproductive phase in Arabidopsis. Biochem Biophys Res Commun. 470:492–497. doi: 10.1016/j.bbrc.2016.01.135
- Zhao DS, Li QF, Zhang CQ, Zhang C, Yang QQ, Pan LX, Ren XY, Lu J, Gu MH, Liu QQ. 2018. GS9 acts as a transcriptional activator to regulate rice grain shape and appearance quality. Nat Commun. 9:1240. doi: 10.1038/s41467-018-03616-y