ABSTRACT
WDR proteins in plants regulate multiple aspects of plant growth and development, as well as plant responses to environmental stimuli. Here, we report the identification of AIW1 (ABA-induced WD40-repeat 1) and AIW2 as novel regulators of salt responses in Arabidopsis. RT-PCR results show that the expression of AIW1 and AIW2 were induced by ABA. Transfection assays in protoplasts show that AIW1 and AIW2 were nucleus proteins and functioned as transcription repressors. When overexpressed in Arabidopsis, AIW1 promoted root elongation, and reduced seedlings sensitivity to ABA. On the other hand, aiw1 aiw2 double mutants showed an increased sensitivity to ABA in seed germination assays. In addition, salt tolerance was reduced in the 35S:AIW1 transgenic plants in root elongation assays. Taken together, our results suggest that AIW1 and AIW2 are ABA-induced plant specific WD40 repeat-containing transcription repressors, and AIW1 and AIW2 function redundantly to regulate ABA and salt responses in Arabidopsis.
1. Introduction
The plant hormone ABA (abscisic acid) regulates plant responses to abiotic stresses via signal transduction (Fujii and Zhu Citation2009; Shang et al. Citation2010; Umezawa et al. Citation2010; Rushton et al. Citation2012; Yoshida et al. Citation2014). ABA signaling is controlled by several key regulators including the receptors PYR1 (Pyrabactin resistance 1)/PYL (PYR1-like)/RCAR (Regulatory component of ABA receptor) (Ma et al. Citation2009; Park et al. Citation2009; Santiago et al. Citation2009), the phosphatases PP2Cs (A-group PROTEIN PHOSPHATASE 2Cs) (Rodriguez et al. Citation1998; Gosti et al. Citation1999), the protein kinases SnRKs (NONFERMENTING 1 (SNF1)-RELATED PROTEIN KINASES) (Fujii et al. Citation2007), and several ABF/AREB/ABI5-type bZIP (basic region leucine zipper) transcription factors (Stone et al. Citation2006; Liu and Stone Citation2010; Chen et al. Citation2013). Signal transduction though the PYR/PYL/RCABR receptors, the PP2C phosphatases and the SnRK kinases activates the ABF/AREB/ABI5-type bZIP transcription factors, resulting in the activation/repression of ABA response genes and the responses of plants to abiotic stresses (Rodriguez et al. Citation1998; Gosti et al. Citation1999; Fujii et al. Citation2007; Umezawa et al. Citation2010; Song et al. Citation2016).
In addition to the ABF/AREB/ABI5-type bZIP transcription factors, members from several other transcription factor families inclduding the R2R3 MYB family, the bHLH (basic Helix–Loop–Helix) family, the NAC (NAM, ATAF1/2, and CUC) family, the GARP (Golden2, ARR-B, Psr1) family, the AITR (ABA-induced transcription repressor) family, and the WDR (WD40-repeat) family have also been reported to regulate ABA and abiotic stress responses in plants (Fujita et al. Citation2004; Tran et al. Citation2004; Jung et al. Citation2008; Jensen et al. Citation2010; Lee et al. Citation2010; Guo et al. Citation2011; Wang et al. Citation2013; Song et al. Citation2016; Tian et al. Citation2017; Ahmad et al. Citation2019; Zheng et al. Citation2019).
WDR proteins are characterized by the presence of tandemly repeated WD40 motifs, ∼40-amino acid stretches typically ending in W (Trp)-D (Asp) (Neer et al. Citation1994). WDR proteins are a superfamily of regulatory proteins found in all eukaryotic cells, with more than 200 members in plants and nearly 350 in human (van Nocker and Ludwig Citation2003; Ouyang et al. Citation2012; Jain and Pandey Citation2018; Feng et al. Citation2019). In addition, a few WDR proteins have also been identified in prokaryotes (Janda et al. Citation1996; Jain and Pandey Citation2018). In lower eukaryotes, WDR proteins are mainly involved in the regulation of growth and development, whereas in higher organisms, they are also involved in the regulation of other cellular functions such as signal transduction, transcriptional regulation, and immune responses (van Nocker and Ludwig Citation2003).
In Arabidopsis, there are nearly 240 genes encoding WDR proteins, and about half of them do not show clear homology with WDR proteins from other eukaryotes (van Nocker and Ludwig Citation2003). WDR proteins in Arabidopsis regulate multiple aspects of plant growth and development. For example, TTG1 (TRANSPARENT TESTA GLABRA 1), a plant specific WDR protein regulates trichome formation, root hair formation and anthocyanin biosynthesis (Galway et al. Citation1994; Walker et al. Citation1999; Bouyer et al. Citation2008; Zhao et al. Citation2008); RACK1 (Receptor for Activated C Kinase 1) proteins regulate seed germination, leaf production, and flowering (Chen et al. Citation2006; Guo and Chen Citation2008); and FRA3 (FRAGILE FIBER3) regulates cell wall formation (Zhong et al. Citation2004). WDR proteins in Arabidopsis also regulate signal transduction including light signaling and phytohormone signaling. For example, COP1 (CONSTITUTIVE PHOTOMORPHOGENIC 1) regulates light signaling (Yi and Deng Citation2005), whereas WDR5a (WD40-REPEAT 5a) regulates auxin signaling (Liu et al. Citation2018).
So far several WDR proteins including GHS40 (GLUCOSE HYPERSENSITIVE 40), MSI1 (MULTICOPY SUPRESSOR OF IRA1), DWA1 (DWD (DDB1-BINDING WD40) HYPERSENSITIVE TO ABA 1), DWD2, and DWD3 have been shown to be involved in the regulation of ABA responses (Lee et al. Citation2010, Citation2011; Hsiao et al. Citation2016; Mehdi et al. Citation2016). These WDR proteins function in different ways to regulate ABA responses in Arabidopsis. DWA1, DWA2, and DWA3 target ABF/AREB/ABI5-type bZIP transcription factors for proteasomal degradation (Lee et al. Citation2010, Citation2011), MSI1 regulates chromatin histone acetylation of the ABA receptor genes, thereby affecting their expression (Mehdi et al. Citation2016), whereas AtGHS40 modulates the expression of ABI4, and possibly ABI1 and SnRK2.6 (Hsiao et al. Citation2016).
We report here the identification of WDR proteins AIW1 (ABA-induced WD40-repeat 1) and AIW2 as novel regulators of ABA and salt responses in Arabidopsis.
2. Materials and methods
2.1. Plant materials and growth conditions
The Arabidopsis (Arabidopsis thaliana) ecotype Columbia-0 (Col) was used as wild-type, and all the mutants and the transgenic plants were in Col background. Seeds of SALK_202135, the T-DNA insertion line for AIW2 (At1g49450) was obtained from the ABRC (Arabidopsis Biological Resource Center), and used to isolate aiw2-1 mutant.
For protoplast isolation and plant transformation, seeds of the Col wild type were sown directly into soil pots and grew in a growth room. For RNA isolation, ABA response and salt tolerance assays, seeds of the Col wild type, mutants and transgenic plants were sterilized with 25% (v/v) bleach for 10 min, washed four times with sterilized water, and plated on 1/2 MS (Murashige & Skoog) plates with vitamins (PlantMedia) and 1% (w/v) sucrose, solidified with 0.6% (w/v) phytoagar (PlantMedia). The plates were kept at 4°C in darkness for 2 days, and then transferred to a growth chamber.
The growth conditions in the growth chamber were set at 22°C, and a long-day condition (16 h light/8 h dark) with photondensity at ~120 μmol m−2 s−1.
2.2. RNA isolation, RT-PCR, and qRT-PCR
To examine the expression of AIW1 and AIW2 in response to ABA, 12-day-old Col wild type seedlings were treated with 50 μM ABA for 4 h, frozen in liquid N2, and used for RNA isolation. Total RNA was isolated and cDNA was synthesizad as described previously (Wang et al. Citation2015a). To examine the expression of AIW1 in the transgenic plants, total RNA was isolated from 12-day-old seedlings. To examine the expression of ABA response genes in the mutants, total RNA was isolated from ABA or NaCl treated 12-day-old Col wild type and aiw1 aiw2 mutants. RT-PCR/qRT-PCR was used to examine the expression of AIW1 and AIW2. The primers used for AIW1 were 5′-CTCGTGTCTCTCGGTATTGATG-3′ and 5′-CAAGCTCCCACTGTACACTATC-3′, for AIW2 were 5′-CGTGAGGTACAAGGCAAAGA-3′ and 5′-CGTCTCCGTCGTTATGATCTTC-3′. The expression of ACT2 (ACTIN2) was used as a control for RT-PCR. The primers for RD22, ABI3, and ACT2 have been reported previously (Ding et al. Citation2014; Liu et al. Citation2015; Ma et al. Citation2019).
2.3. Constructs
The nuclear indicator construct NLS-RFP, the reporter construct LexA-Gal4:GUS, and the effector construct GD and LD-VP used for protoplast transfection have been described previously (Lee et al. Citation2001; Tiwari et al. Citation2003; Wang et al. Citation2007).
To generate GD-AIW1 (At3g18950), GD-AIW2, AIW1-GFP, and AIW2-GFP constructs for protoplast transfection, the full-length ORF (open reading frame) sequences of AIW1 and AIW2 were amplified by RT-PCR using RNA isolated from 12 days Col wild type seedlings, digested with proper enzymes and cloned in frame with an N-terminal GD or a C-terminal GFP tag into pUC19 vector under the control of the CaMV 35S promoter (Tiwari et al. Citation2003; Wang et al. Citation2007).
To generate 35S:AIW1 construct for plant transformation, the full-length ORF sequence of AIW1 amplified by RT-PCR was digested with proper enzymes and cloned in frame with an N-terminal HA tag into pUC19 vector under the control of the CaMV 35S promoter. The pUC19-35S:AIW1 construct was then digested with proper enzymes and cloned into the binary vector pPZP211 (Hajdukiewicz et al. Citation1994).
To generate CRISPR/Cas9 constructs for gene editing of AIW1 and/or AIW2, exon sequences of AIW1 and AIW2 were subjected to CRISPRscan (http://www.crisprscan.org/?page=sequence) to identify potential target sequences. Selected target sequences were then evaluated on Cas-OFFinder (http://www.rgenome.net/cas-offinder/). The specific target sequences selected for AIW1 editing were 5′-TGGCCTCTGAGTGAAAGCGG(AGG)-3′ and 5′-GGAGGAAGAATTTGAGGCTA(GGG)-3′, for AIW2 were 5′-GTCACAACGATTATCTTAGG(AGG)-3′ and 5′-GTGGTAAGTTTCTTCCTCGG(CGG)-3′. The target sequences were inserted into the pHEE401E vector as described by Wang et al. (Citation2015b). The primers used to generate CRISPR/Cas9 construct for editing AIW1 were DT1-BsF (AIW1), 5′-ATATATGGTCTCGATTGGGAGGCGAAAGTGAGTCTCGTT-3′, DT1-F0 (AIW1), 5′-TGGGAGGCGAAAGTGAGTCTCGTTTTAGAGCTAGAAATAGC-3′, DT2-R0 (AIW1), 5′-AACCCTTCTTAAACTCCGATCCCAATCTCTTAGTCGACTCTAC-3′, and DT2-BsR (AIW1), 5′-ATTATTGGTCTCGAAACCCTTCTTAAACTCCGATCCC-3′. for AIW2 were DT1-BsF(AIW2), 5′-ATATATGGTCTCGATTGGCACAACGATTATCTTAGGGTT-3′, DT1-F0 (AIW2), 5′-TGGCACAACGATTATCTTAGGGTTTTAGAGCTAGAAATAGC-3′, DT2-R0 (AIW2), 5′-AACCCGAGGAAGAAACTTACCCCAATCTCTTAGTCGACTCTAC-3′, and DT2-BsR (AIW2), 5′-ATTATTGGTCTCGAAACCCGAGGAAGAAACTTACCCC-3′. U626-IDF and U629-IDR, the primers used for colony PCR and sequencing of the CRISPR/Cas9 constructs generated have been described previously (Chen et al. Citation2019).
2.4. Plant transformation, transgenic plant selection, and Cas9-free mutant isolation
About 5-week-old plants with several mature flowers were transformed by using the floral dip method (Clough and Bent Citation1998). The Col wild type plants were transformed with the 35S:AIW1 and the CRISPR/Cas9 constructs to generate 35S:AIW1 overexpression plants and aiw1 and aiw2 single mutants, respectively. The Cas9-free aiw2-c1 single mutant plants were transformed with AIW1 CRISPR/Cas9 construct to generate aiw1 aiw2 double mutants.
The 35S:AIW1 overexpression plants were selected by plating the T1 seeds on 1/2 MS plates containing 50 μg/ml Kanamycin and 100 μg/ml Carbenicillin. Multiple independent overexpression lines were obtained and two homozygous T3 lines with confirmed high expression levels of AIW1 were used for the experiments.
Transegenic plants generated with the CRISPR/Cas9 constructs were selected by plating the T1 seeds on 1/2 MS plates containing 30 μg/ml Hygromycin and 100 μg/ml Carbenicillin. Gene editing in the T1 plants was examined by amplifying and sequencing the genomic sequence of AIW1 and AIW2. T2 seeds collected from gene edited T1 plants were germinated in soil pots directly and used to identify homozygous Cas9-free mutants.
2.5. DNA isolation and PCR
To examine the editing status of AIW1 and AIW2, DNA was isolated from leaves of T1 or T2 transgenic plants generated using the CRISPR/Cas9 constructs, and used for PCR amplification of the genomic sequences of AIW1 and AIW2, respectively. To isolate Cas9-free mutants, DNA was isolated from leaves of T2 offsprings of gene edited T1 plants, and used for PCR amplification of Cas9 gene fragment. The primers used for PCR amplification of Cas9 have been described previously (Chen et al. Citation2019).
2.6. Plasmid DNA isolation, protoplast isolation, and transfection
Plasmid DNA of the reporter and effector genes was isolated by using the GoldHi Endo Free Plasmid Maxi Kit (CWBIO). Protoplasts were isolated from rosette leaves of 3–4-week-old Col wild type plants, and transfected as described previously (Wang et al. Citation2005, Citation2015a; Dai et al. Citation2016; Tian et al. Citation2017).
For subcellular location assays, plasmids of AIW1-GFP and AIW2-GFP were transfected into protoplasts, respectively. Plasmids of NLS-RFP were co-transfected as a nuclear marker (Xu et al. Citation2013). For transcriptional activity assays, plasmids of the reporter gene LexA-Gal4:GUS and the effector genes GD, LD-VP, and GD-AIW1 or GD-AIW2 were co-transformed into protoplasts. The transfected protoplasts were incubated at room temperature in dark for 20–22 h before GFP florescence was observed, or GUS activities were measured.
GFP fluorescence was observed under an Olympus FV1000 confocal microscope. GUS activities were measured using a SynergyTM HT microplate reader.
2.7. Root elongation, ABA, and salt sensitivity assays
For root elongation assays, sterilized seeds of the Col wild type, the 35S:AIW1 transgenic plants, the aiw1 and aiw2 single, and the aiw1 aiw2 double mutants were generated on vertical placed 1/2 MS plates. The root length of 8-day-old seedlings was measured.
ABA inhibited seed germination, cotyledon greening and root elongation assays were performed as described previously (Guo et al. Citation2009; Tian et al. Citation2015; Zheng et al. Citation2019), unless indicated otherwise.
For salt tolerance assays, 4-day-old seedlings grown on vertical placed plates were transferred to new plates containing 150 mM NaCl, and grown vertically for 6 more days before pictures were taken. Root length before transfer and 6 days after transfer were measured, and the percentage of inhibition was calculated. All the experiments were repeated at least three times.
3. Results
3.1. Expression of AIW1 and AIW2 is regulated by ABA
In the process to identified novel players in ABA signaling using a strategy as described previously (Tian et al. Citation2017), we found that the expression level of a WDR gene AIW1 was increased in response to ABA treatment in our previously transcriptome data set (Guo et al. Citation2011).
To explore the possible roles of AIW1 in ABA response in Arabidopsis, we first wanted to confirm ABA response of AIW1. To do that, Arabidopsis seedlings were treated with ABA, and expression of AIW1 was examined by RT-PCR. As shown in (a), the expression level of AIW1 in Arabidopsis seedlings was dramatically increased in response to ABA treatment. According to CDD/SPARCLE analysis (Marchler-Bauer et al. Citation2017), AIW1 contains WD40 repeats ((b)), therefore it is a WDR protein, we thus named it ABA-induced WD40 repeat 1.
Figure 1. AIW1 and AIW2 are closely related ABA responsive genes. (a) Expression of AIW1 and AIW2 in response to ABA. Twelve-day-old Col seedlings were mock-treated or treated with 50 µM ABA for 4 h, total RNA was isolated, and cDNA was synthesized and used for RT-PCR. ACT2 was used as a control. (b) Amino acid sequence alignment of AIW1 and AIW2. Identical amino acids were shaded in dark gray, and similar ones in light gray. Underlines indicate the WD40 repeats. (c) Amino acid identity and similarity of AIW1 and AIW2. Full-length amino acid sequences of AIW1 and AIW2 were subjected to SIAS (http://imed.med.ucm.es/Tools/sias.html) for identity and similarity assay.
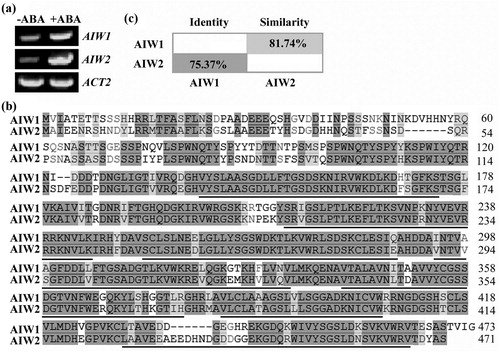
Amino acid sequence BLASTing indicated that AIW2 is closely related to AIW1, with a 75.37% identity and an 81.74% similarity on entire amino acid levels ((c)). RT-PCR results showed that the expression level of AIW2 in Arabidopsis seedlings was also dramatically increased in response to ABA treatment ((a)).
3.2. AIW1 and AIW2 are transcription repressors
According to previously report, homologs for AIW1 and AIW2 were not identified from budding yeast, fruit fly, and human (van Nocker and Ludwig Citation2003), suggesting that AIW1 and AIW2 are likely to plant specific WDR proteins, therefore may have specific functions in plants. To explore the functions of AIW1 and AIW2 in Arabidopsis, we examined if they may confer transcriptional activities.
Subcellular localization of AIW1 and AIW2 was examined by using Arabidopsis protoplast transient transfection assays. Plasmids of AIW1-GFP and AIW2-GFP were transfected into Arabidopsis protoplasts respectively, and GFP fluorescence was observed under a cofocal microscope after the transfected protoplasts were incubated overnight in darkness. As shown in (a), AIW1 and AIW2 were predominantly localized in the nucleus.
Figure 2. AIW1 and AIW2 are transcription repressors. (a) Subcellular localization of AIW1 and AIW2. Plasmids of the effector genes AIW1-GFP and AIW2-GFPS were transfected into Arabidopsis protoplasts, respectively. Plasmids of NLS-RFP were co-transfected as a nuclear marker. The transfected protoplasts were incubated in dark for 20–22 h at room temperature, then GFP and RFP fluorescence was observed under a confocal microscope. (b) Transcriptional activities of AIW1 and AIW2. Plasmids of the reporter gene LexA-Gal4:GUS and the effector genes GD, LD-VP and GD-AIW1 or GD-AIW2 were co-transfected into protoplasts. The transfected protoplasts were incubated in dark for 20–22 h at room temperature, then GUS activities were measured by using a microplate reader. Data represent the mean ± SD of three replicates. *Significantly different from the control (p < .005).
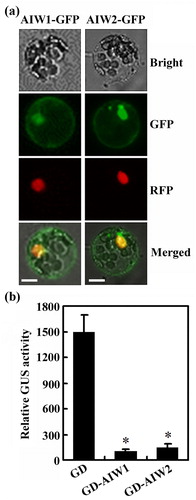
We then examined the transcriptional activities of AIW1 and AIW2 by using protoplast transfection. Plasmids of the reporter gene LexA-Gal4:GUS and the effect gene LD-VP, GD-AIW1, GD-AIW2, or the control gene GD were co-transfected into protoplasts, and GUS activities were measured by using a microplate reader after the transfected protoplasts were incubated overnight in darkness. As shown in (b), expression of the reporter gene was activated by co-transfection of the activator gene LD-VP and the control gene GD, while co-transfection of the effector gene GD-AIW1 or GD-AIW2 resulted in repression of the reporter gene activated by the transcription activator LD-VP, indicating that AIW1 and AIW2 function as transcription repressors.
3.3. Overexpression of AIW1 in Arabidopsis promotes root elongation
Having shown that the expression levels of AIW1 and AIW2 were up-regulated by ABA treatment (), and AIW1 and AIW2 were able to repress reporter gene expression when recruited to the promoter region of the reporter gene (), we further explored the functions of AIW1 and AIW2 in plant by generating and analyzing overexpression plants and loss-of-function mutants of the AIW genes.
One T-DNA insertion line for AIW2 was available from TAIR, we thus isolated the single mutant aiw2-1 from the T-DNA insertion line ((a)). Since genome editing by CRISPR/Cas9 is able to generate transgene-free mutants (Ma et al. Citation2015; Gao et al. Citation2016; Lu et al. Citation2017; He et al. Citation2018). Recently, we have also successfully generated transgene-free Arabidopsis by using CRISPR/Cas9 (Chen et al. Citation2019), therefore we generated all other mutants need for the experiments, including the aiw1 and aiw2 single and the aiw1 aiw2 double mutants by using CRISPR/Cas9 genome editing ((a)). In the mutants, a single nucleotide insertion, two nucleotides deletion, or a small fragment deletion have occurred for AIW1, and a single nucleotide insertion was occurred for AIW2 ((b)), resulting in a few amino acid substitutions and premature stops for AIW1 and AIW2, respectively ((c)). Transgenic plants overexpressing AIW1 were generated by expressing AIW1 under the control of the 35S promoter (35S:AIW1) in the Col wild type plants. Quantitative RT-PCR results show that AIW1 was highly expressed in the 35S:AIW1 transgenic plants, whereas that in the gene edited mutants remained largely unchanged ((a)), consistent with small indel nature of the mutants.
Figure 3. Generation of the aiw1 and aiw2 single, and the aiw1 aiw2 double mutants. (a) T-DNA insertion position of the aiw2-1 single mutant, and the edited sites in the gene edited mutants. Seeds of the T-DNA line SALK_202135C was obtained from ABRC, and the aiw2-1 mutant was identified by genotyping. Gene edited mutants were obtained by CRISPR/Cas9 gene editing. (b) Alignment of the targeted nucleotide sequences of AIW1 and AIW2 by CRISPR/Cas9 in the Col wild type, the aiw1 and aiw2 single and the aiw1 aiw2 double mutants. The mutants were obtained by transforming Col wild type plants with AIW1 or AIW2 targeting pHEE constructs, examining the editing status in T1 generation, and isolating Cas9-free homozygous mutants in T2 generation. Numbers beside and above the sequences indicate the position of the nucleotides relative to the start codon. Underlines indicate the PAM sites. (c) Alignment of AIW1 and AIW2 amino acid sequences in the Col wild type, the aiw1 and aiw2 single and the aiw1 aiw2 double mutants. AIW1 and AIW2 sequences in the mutants were subjected to ORF analysis by using ORFfinder (https://www.ncbi.nlm.nih.gov/orffinder/), and predicted amino acid sequences were used for alignment with AIW1 and AIW2 amino acid sequences, respectively. Numbers beside the sequences indicate the position of the amino acid relative to the Met encoded by the start codon.
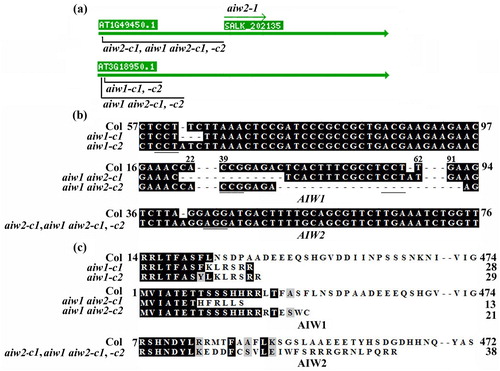
Figure 4. Root elongation of the 35S:AIW1 transgenic plants, the aiw1 and aiw2 single and the aiw1 aiw2 double mutant seedlings. (a) Expression of AIW1 in Col wild type, the 35S:AIW1 transgenic plants, the aiw1 and aiw2 single and the aiw1 aiw2 double mutants. Total RNA was isolated from 12-day-old seedlings, and qRT-PCR was used to examine the expression of AIW1. ACT2 was used as an inner control, and the expression of AIW1 in the Col wild type was set as 1. Data represent the mean ± SD of three replicates. *Significantly different from that in the Col wild type (p < .0001). (b) Primary root length of 8-day-old seedlings of the Col wild type, the 35S:AIW1 transgenic plants, the aiw1 and aiw2 single and the aiw1 aiw2 double mutants. Sterilized seeds were plated on 1/2 MS plates, the plates were kept at 4°C and in darkness for 2 days before transferred to and grown vertically in a growth room. Root length of the seedlings was measure 8 days after the transfer, and average root length was calculated. Data represent the mean ± SD of 25–31 seedlings. *Significantly different from the Col wild type (p < .0001).
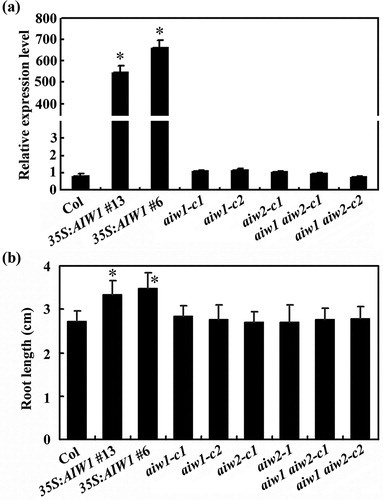
By analyzing the overexpression plants and the mutants obtained, we found that primary root length in the 35S:AIW1 transgenic plant seedlings were increased when compared with the Col wild type seedlings. As shown in (b), the primary root length of the 35S:AIW1 transgenic plant seedlings increased ∼20% when compared with the Col wild type seedlings. On the other hand, the primary root length of the aiw1 and aiw2 single, and the aiw1 aiw2 double mutant seedlings was largely similar to that of the Col wild type seedlings ().
3.4. The 35s:AIW1 transgenic plants are hyposensitivity, whereas the aiw1 aiw2 double mutants are hypersensitive to ABA
We next examined if AIW1 and AIW2 may regulate ABA response by using three common used assays for plant response to ABA, i.e. seed germination, cotyledon greening, and root elongation assays.
As shown in , in the control plates, all the seeds including seeds of the Col wild type, the 35S:AIW1 transgenic plants, the aiw1 and aiw2 single, and the aiw1 aiw2 double mutants germinated 36 h after the plates were transferred to growth room. All the seeds germinated much slower in the ABA-containing plates. However, lower germination rate was observed for the aiw1 aiw2 double mutant seeds at nearly all the time points (). There results suggest that the aiw1 aiw2 double mutants are more sensitive to ABA.
Figure 5. Effects of ABA on seed germination of the Col wild type, the 35S:AIW1 transgenic plants, the aiw1 and aiw2 single and the aiw1 aiw2 double mutants. Sterilized seeds of the Col wild type, the 35S:AIW1 transgenic plants, the aiw1 and aiw2 single, and the aiw1 aiw2 double mutants were plated on 1/2 MS plates with or without 1 µM ABA. The plates were kept at 4°C and in darkness for 2 days before transferred to a growth room. The number of seeds germinated was counted at indicated time points, and percentage of germination was calculated. Data represent the mean ± SD of three replicates.
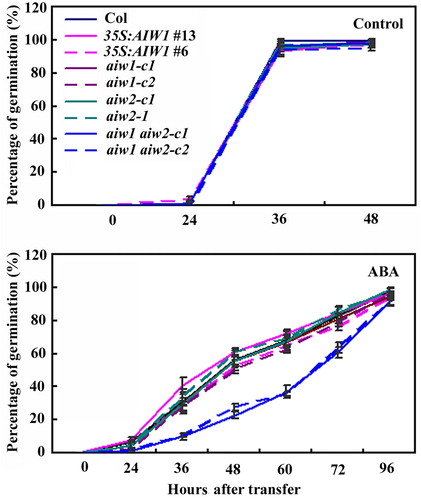
In the cotyledon greening assays, the 35S:AIW1 plants showed reduced sensitivity to ABA treatment ((a)). Quantitative assays showed that the green cotyledon rate of the 35S:AIW1 plants was nearly twice of the Col wild type plants, whereas that of the mutants was largely indistinguishable to the Col wild type plants ((b)).
Figure 6. Effects of ABA on cotyledon greening of the Col wild type, the 35S:AIW1 transgenic plants, the aiw1 and aiw2 single and the aiw1 aiw2 double mutants. (a) Cotyledon greening of the Col wild type, the 35S:AIW1 transgenic plants, the aiw1 and aiw2 single and the aiw1 aiw2 double mutants. Sterilized seeds were plated on 1/2 MS plates with or without 1 µM ABA. The plates were kept at 4°C in darkness for 2 days before transferred to a growth room. Pictures were taken 14 days after the transfer. (b) Quantitative of green seedlings of the Col wild type, the 35S:AIW1 transgenic plants, the aiw1 and aiw2 single and the aiw1 aiw2 double mutants in response to ABA treatment. Seedlings with green cotyledons were counted 14 days after the transfer, and percentage of green cotyledons was calculated. Data represent the mean ± SD of three replicates. *Significantly different from the Col wild type (p < .001).
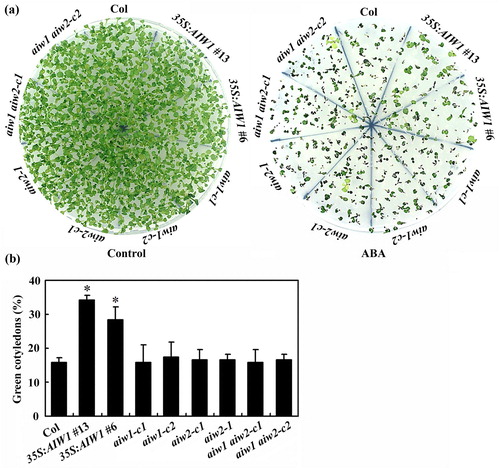
Similarly, the 35S:AIW1 transgenic plant seedlings also showed reduced sensitivity to ABA treatments in root elongation assays ((a)). Quantitative results showed that root elongation of wild-type seedlings was inhibited about 30% by 5 μM ABA, whereas that of 35S:AIW1 transgenic plant seedlings was only about 10% ((b)). We found that sensitive to ABA treatment was slightly increased in all the mutant seedlings when compared with the Col wild type seedlings, with a root elongation inhibition rate of ∼40% ((b)).
Figure 7. Effects of ABA and NaCl on root elongation of the Col wild type, the 35S:AIW1 transgenic plants, the aiw1 and aiw2 single and the aiw1 aiw2 double mutants. (a) Ten-day-old seedlings of the Col wild type, the 35S:AIW1 transgenic plants, the aiw1 and aiw2 single and the aiw1 aiw2 double mutants. Sterilized seeds were plated on 1/2 MS plates. The plates were kept at 4°C and in darkness for 2 days, transferred to a growth room and grown vertically for 4 days. The seedlings were then transferred to control plates and plates containing 5 μM ABA or 150 mM NaCl, and grown for 6 more days. (b) Quantitative of root elongation inhibition by ABA. Length of new elongated roots was measured, and percentage of inhibition was calculated. Data represent means ± SD of 20–29 seedlings. Significantly different from the Col wild type (*p < .0001, **p < .05). (c) Quantitative of root elongation inhibition by NaCl. Data represent means ± SD of 16–26 seedlings. *Significantly different from the Col wild type (p < .0001). (d) Expression of RD22 in response to ABA and ABI3 in response to NaCl in the Col wild type and the aiw1 aiw2 double mutants. Seedlings were treated with 50 µM ABA or 150 mM NaCl for 4 h, total RNA was isolated and qRT-PCR was used to examine the expression of RD22 and ABI3, respectively. ACT2 was used as an inner control, and the expression of corresponding gene in the Col wild type control seedlings was set as 1. Data represent the mean ± SD of three replicates. Significantly different from that in the Col wild type (*p < .05, **p < .005).
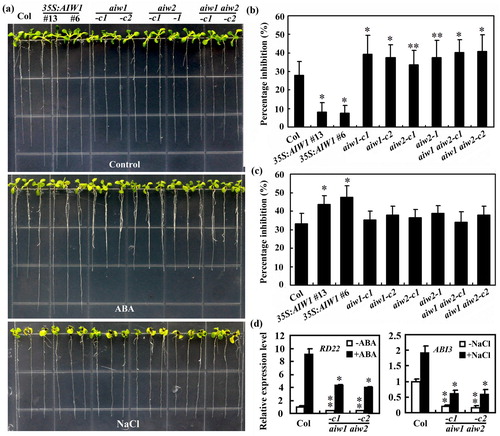
3.5. Salt tolerance is decreased in the 35s:AIW1 transgenic plants
ABA is key hormone that regulates plant response to abiotic stresses, having shown that AIW1 and AIW2 regulate ABA response in Arabidopsis, we further examined if AIW1 and AIW2 may also regulate plant response to abiotic stresses. We found that salt tolerance is reduced in the 35S:AIW1 transgenic plants ((a)). Quantitative results showed that root elongation of the Col wild-type seedlings was inhibited ∼35% by 150 mM NaCl, whereas that of 35S:AIW1 plant seedlings was ∼45%. On the other hand, all seedlings of the mutants including the aiw1 and aiw2 single and aiw1 aiw2 double mutants showed a near wild type response to salt treatments ((c)).
To examine how AIW1 and AIW2 may regulate ABA and salt responses in Arabidopsis, we examined the expression of some ABA signaling genes and genes known to be regulated by ABA and/or salt, such as ABF1, ABF3, ABI1, ABI3, RD22, and RD29B by qRT-PCR. We found that even though the responses of aiw1 aiw2 double mutants to ABA changed slightly, and to salt remained largely unchanged when compared with that in the Col wild type, the expression of RD22, an ABA responsive gene (Yamaguchi-Shinozaki and Shinozaki Citation1993), and ABI3, a transcription factor gene involved in the regulation of seed development and germination (Giraudat et al. Citation1992) were reduced to ABA and salt, respectively in the mutants ((d)).
4. Discussion
Several different types of transcription factors including a few WDR proteins are involved in the regulation of ABA responses in Arabidopsis (Lee et al. Citation2010, Citation2011; Hsiao et al. Citation2016; Mehdi et al. Citation2016; Song et al. Citation2016). We provide evidence here that AIW1 and AIW2 are ABA-induced WDR transcription repressor genes, and AIW1 and AIW2 play a role in the regulation of ABA response and salt tolerance in Arabidopsis.
From a previously transcriptome data set (Guo et al. Citation2011), we identified AIW1 as one of the functional uncharacterized ABA response genes. RT-PCR results showed that the expression levels of both AIW1 and its closely related gene AIW2 were increased in response to ABA treatments (), confirmed that AIW1 and AIW2 are ABA response genes. Both AIW1 and AIW2 contain 7 WD40 tandem repeats (), suggesting that they are WDR proteins. By analyzing ABA responses of the AIW1 overexpressing plants, aiw1 and aiw2 single and aiw1 aiw2 double mutants, we found that AIW1 and AIW2 are involved in the regulation of ABA response in Arabidopsis (–). The plants showed a slight difference in response to ABA in different assays, i.e. the 35S:AIW1 transgenic plants showed hyposensitive to ABA in seedling greening () and root elongation assays () but a wild type response to ABA in seed germination assays (), whereas the aiw1 aiw2 double mutants showed hypersensitive to ABA in seed germination assay (), slightly increased sensitive to ABA in root elongation assays (), and a wild type response in seedling greening assays (). These data suggest that AIW1 and AIW2 may have redundant functions in regulating ABA regulated seed germination, but may not have redundant functions in regulating ABA regulated seedling greening and root elongation. Amino acid sequence BLASTing on phytozome (https://phytozome.jgi.doe.gov/pz/portal.html) indicated that there are eight more WDR proteins are homologs of AIW1 and AIW2, it is worthwhile to further examine if these proteins may have redundant functions with AIW1 and AIW2 in regulating ABA response. On the other hand, these data, in general, suggest that ABA sensitive was reduced in the 35S:AIW1 transgenic plants, but increased in the aiw1 aiw2 double mutants, indicating that AIW1 and AIW2 are negative regulators for ABA responses in Arabidopsis.
ABA signal transduction resulted in the activation of ABA response genes and plant response to abiotic stresses (Rodriguez et al. Citation1998; Gosti et al. Citation1999; Fujii et al. Citation2007; Umezawa et al. Citation2010; Song et al. Citation2016). As a result, changes in the expression of ABA signaling or response genes affected not only plant responses to ABA, but also plant tolerance to abiotic stresses. For example, overexpression of the receptor gene PYR/PYL/RCAR enhanced drought tolerance (Park et al. Citation2015; Zhao et al. Citation2016), loss-of-function of the kinase genes SnRKs reduced drought tolerance (Fujita et al. Citation2009), overexpression of the transcription factor gene ERF96 enhanced salt tolerance (Wang et al. Citation2017), and loss-of-function of the transcription factor genes AITRs enhanced drought and salt tolerance (Tian et al. Citation2017). We found that overexpression of AIW1 affected plant response to ABA (–), and salt tolerance in the 35S:AIW1 transgenic plants was decreased (). However, the aiw1 aiw2 double mutants showed a wild type response to salt (). It will be also interested to examine if any of the 8 homolog gens of AIW1 and AIW2 may also be ABA response genes, and if they may function redundantly to regulate ABA response and salt tolerance in Arabidopsis.
The presence of WD40 motifs is the only common feature of WDR proteins (Neer et al. Citation1994; van Nocker and Ludwig Citation2003; Ouyang et al. Citation2012; Jain and Pandey Citation2018), therefore WDR proteins may composed of different types of proteins. Taken the ABA response regulating WDRs as examples, MSI1 is part of a histone deacetylase complex (Mehdi et al. Citation2016), DWA1, DWA2 and DWA3 are components of CUL4-based E3 ligases (Lee et al. Citation2010, Citation2011), and 5PTase13 is a myoinositol polyphosphate 5-phosphatase (Ananieva et al. Citation2008). Our results in Arabidopsis protoplast transfection assays show that AIW1 and AIW2 functioned as transcription repressors (). To examine how AIW1 and AIW2 may regulate ABA response in Arabidopsis, we compared the expression levels of the key ABA signaling regulator genes in the 35S:AIW1 transgenic plants and aiw1 aiw2 double mutants with Col wild type, but no difference were observed. Therefore it is likely AIW1 and AIW2 regulate some other genes that may affect plant responses to ABA and abiotic stresses.
Many studies have shown that WD motifs serve as a scaffold for protein–protein interaction, allowing WDR proteins to form multiple protein complexes with other proteins (van Nocker and Ludwig Citation2003; Jain and Pandey Citation2018; Feng et al. Citation2019). For example, TTG1 interacts with R2R3 MYB and bHLH proteins to form MBW activator complexes in regulating cell fate determination and anthocynin biosynthesis (Schiefelbein Citation2003; Pesch and Hülskamp Citation2004; Ramsay and Glover Citation2005; Wang and Chen Citation2014), even though TTG1 may not be required for the activation of downstream genes of the complexes (Wang et al. Citation2010); whereas the TPL (TOPLESS)/TPR (TOPLESS-RELATED) co-repressors interact with different types of proteins to repress gene expression (Collins et al. Citation2019). Thus it will be of great interest to examine if AIW1 and AIW2 may interact with other proteins to regulate ABA and abiotic stress responses, and what are the target genes if any.
Nevertheless, our results showed that AIW1 and AIW2 are ABA-induced WDR transcription repressor genes, and AIW1 and AIW2 function redundantly to regulate ABA and salt responses in Arabidopsis.
Acknowledgements
SW conceived the study. XW, WW, LY, TW, and SW designed the experiments. XW, WW, GZ, SL, DL, A, AH, SA, and CZ performed the experiments. XW, WW, and SW analyzed the data and drafted the manuscript. All the authors participated in the revision of the manuscript.
Disclosure statement
No potential conflict of interest was reported by the author(s).
Additional information
Funding
Notes on contributors
Xutong Wang
Xutong Wang is a doctoral candidate in botany in School of Life Sciences at Northeast Normal University, and an exchange student at Concordia University, Canada. Her work focuses on investigations of ABA signaling and plant abiotic stress responses, and MAPK signaling.
Wei Wang
Wei Wang is a doctoral candidate in botany in School of Life Sciences at Northeast Normal University. Her work focuses on investigations of the roles of transcription factors in plant growth and development, and plant responses to abiotic stresses.
Yating Wang
Yating Wang is a doctoral candidate in botany in School of Life Sciences at Northeast Normal University. Her work focuses on investigations of ABA responsive genes in plant growth and development, and plant response to abiotic stresses.
Ganghua Zhou
Ganghua Zhou is a master student in Genetics in School of Life Sciences at Northeast Normal University. His work focuses on investigations of ABA responsive genes in plant abiotic stress responses.
Shanda Liu
Shanda Liu was a master student in Genetics in School of Life Sciences at Northeast Normal University. He worked on investigations of ABA and auxin signaling. He is currently a doctoral candidate at Max-Planck Institute for Plant Breeding Research, Germany.
Dongqiu Li
Dongqiu Li was a master student in Genetics in School of Life Sciences at Northeast Normal University. She worked on investigations of ABA responsive genes in plant abiotic stress responses. She is currently a secondary school teacher in Changchun.
Adnan
Adnan is a master student in Genetics in School of Life Sciences at Northeast Normal University. Her work focuses on the roles of transcription factors in ABA signaling and plant abiotic stress responses.
Saddam Hussain
Saddam Hussain is a doctoral candidate in botany in School of Life Sciences at Northeast Normal University. His work focuses on investigations of ABA responsive genes in plant growth and development, and plant response to abiotic stresses.
Sajjad Ahmed
Sajjad Ahmed was a master student in Genetics in School of Life Sciences at Northeast Normal University. He worked on investigations of ABA responsive genes in plant abiotic stress responses. He is currently a doctoral candidate at York University, Canada.
Chen Zhang
Chen Zhang is a master candidate in Genetics in School of Life Sciences at Northeast Normal University. Her work focuses on investigations of ABA responsive genes in plant abiotic stress responses.
Li Yan
Dr Li Yan is a professor in School of Life Sciences at Linyi University, and a member of the Plant Molecular Genetics & Crop Gene Editing research group. Her research interest focuses on the regulation of plant response to abiotic stresses.
Tianya Wang
Dr Tianya Wang is lecturer in School of Life Sciences at Northeast Normal University, and a member of Key Laboratory of Molecular Epigenetics of MOE. Her research interest focuses on epigenetic regulation of plant secondary metabolisms.
Shucai Wang
Dr Shucai Wang is a professor in School of Life Sciences at Linyi University, and leader of the Plant Molecular Genetics & Crop Gene Editing research group. His research interest focuses on the roles of transcription factors in hormone signaling, plant growth and development, and plant response to abiotic stress responses.
References
- Ahmad R, Liu Y, Wang TJ, Meng Q, Yin H, Wang X, Wu Y, Nan N, Liu B, Xu ZY. 2019. GOLDEN2-LIKE transcription factors regulate WRKY40 expression in response to abscisic acid. Plant Physiol. 179:1844–1860. doi: 10.1104/pp.18.01466
- Ananieva EA, Gillaspy GE, Ely A, Burnette RN, Erickson FL. 2008. Interaction of the WD40 domain of a myoinositol polyphosphate 5-phosphatase with SnRK1 links inositol, sugar, and stress signaling. Plant Physiol. 148:1868–1882. doi: 10.1104/pp.108.130575
- Bouyer D, Geier F, Kragler F, Schnittger A, Pesch M, Wester K, Balkunde R, Timmer J, Fleck C, Hülskamp M. 2008. Two-dimensional patterning by a trapping/depletion mechanism: the role of TTG1 and GL3 in Arabidopsis trichome formation. PLoS Biol. 6:e141. doi: 10.1371/journal.pbio.0060141
- Chen JG, Ullah H, Temple B, Liang J, Guo J, Alonso JM, Ecker JR, Jones AM. 2006. RACK1 mediates multiple hormone responsiveness and developmental processes in Arabidopsis. J Exp Bot. 57:2697–2708. doi: 10.1093/jxb/erl035
- Chen S, Zhang N, Zhang Q, Zhou G, Tian H, Hussain S, Ahmed S, Wang T, Wang S. 2019. Genome editing to integrate seed size and abiotic stress tolerance traits in Arabidopsis reveals a role for DPA4 and SOD7 in the regulation of inflorescence architecture. Int J Mol Sci. 20. 2695. doi: 10.3390/ijms20112695
- Chen YT, Liu HX, Stone S, Callis J. 2013. ABA and the ubiquitin E3 ligase KEEP ON GOING affect proteolysis of the Arabidopsis thaliana transcription factors ABF1 and ABF3. Plant J. 75:965–976. doi: 10.1111/tpj.12259
- Clough SJ, Bent AF. 1998. Floral dip: a simplified method for Agrobacterium-mediated transformation of Arabidopsis thaliana. Plant J. 16:735–743. doi: 10.1046/j.1365-313x.1998.00343.x
- Collins J, O'Grady K, Chen S, Gurley W. 2019. The C-terminal WD40 repeats on the TOPLESS co-repressor function as a protein-protein interaction surface. Plant Mol Biol. 100:47–58. doi: 10.1007/s11103-019-00842-w
- Dai X, Zhou L, Zhang W, Cai L, Guo H, Tian H, Schieflbein J, Wang S. 2016. A single amino acid substitution in the R3 domain of GLABRA1 leads to inhibition of trichome formation in Arabidopsis without affecting its interaction with GLABRA3. Plant Cell Environ. 39:897–907. doi: 10.1111/pce.12695
- Ding ZJ, Yan JY, Li GX, Wu ZC, Zhang SQ, Zheng SJ. 2014. WRKY41 controls Arabidopsis seed dormancy via direct regulation of ABI3 transcript levels not downstream of ABA. Plant J. 79:810–823. doi: 10.1111/tpj.12597
- Feng R, Zhang C, Ma R, Cai Z, Lin Y, Yu M. 2019. Identification and characterization of WD40 superfamily genes in peach. Gene . pii: S0378-1119(19)30558-X.
- Fujii H, Verslues PE, Zhu JK. 2007. Identification of two protein kinases required for abscisic acid regulation of seed germination, root growth, and gene expression in Arabidopsis. Plant Cell. 19:485–494. doi: 10.1105/tpc.106.048538
- Fujii H, Zhu JK. 2009. Arabidopsis mutant deficient in 3 abscisic acid-activated protein kinases reveals critical roles in growth, reproduction, and stress. Proc Natl Acad Sci USA. 106:8380–8385. doi: 10.1073/pnas.0903144106
- Fujita M, Fujita Y, Maruyama K, Seki M, Hiratsu K, Ohme-Takagi M, Tran L-SP, Yamaguchi-Shinozaki K, Shinozaki K. 2004. A dehydration-induced NAC protein, RD26, is involved in a novel ABA-dependent stress-signaling pathway. Plant J. 39:863–876. doi: 10.1111/j.1365-313X.2004.02171.x
- Fujita Y, Nakashima K, Yoshida T, Katagiri T, Kidokoro S, Kanamori N, Umezawa T, Fujita M, Maruyama K, Ishiyama K, et al. 2009. Three SnRK2 protein kinases are the main positive regulators of abscisic acid signaling in response to water stress in Arabidopsis. Plant Cell Physiol. 50:2123–2132. doi: 10.1093/pcp/pcp147
- Galway ME, Masucci JD, Lloyd AM, Walbot V, Davis RW, Schiefelbein JW. 1994. The TTG gene is required to specify epidermal cell fate and cell patterning in the Arabidopsis root. Dev Biol. 166:740–754. doi: 10.1006/dbio.1994.1352
- Gao X, Chen J, Dai X, Zhang D, Zhao Y. 2016. An effective strategy for reliably isolating heritable and Cas9-free Arabidopsis mutants generated by RISPR/Cas9-mediated genome editing. Plant Physiol. 171:1794–1800. doi: 10.1104/pp.16.00663
- Giraudat J, Hauge BM, Valon C, Smalle J, Parcy F, Goodman HM. 1992. Isolation of the Arabidopsis ABI3 gene by positional cloning. Plant Cell. 4:1251–1261.
- Gosti F, Beaudoin N, Serizet C, Webb AA, Vartanian N, Giraudat J. 1999. ABI1 protein phosphatase 2C is a negative regulator of abscisic acid signaling. Plant Cell. 11:1897–1909. doi: 10.1105/tpc.11.10.1897
- Guo J, Chen JG. 2008. RACK1 genes regulate plant development with unequal genetic redundancy in Arabidopsis. BMC Plant Biol. 8:108. doi: 10.1186/1471-2229-8-108
- Guo J, Wang J, Xi L, Huang WD, Liang J, Chen JG. 2009. RACK1 is a negative regulator of ABA responses in Arabidopsis. J Exp Bot. 60:3819–3833. doi: 10.1093/jxb/erp221
- Guo J, Wang S, Valerius O, Hall H, Zeng Q, Li JF, Weston DJ, Ellis BE, Chen JG. 2011. Involvement of Arabidopsis RACK1 in protein translation and its regulation by abscisic acid. Plant Physiol. 155:370–383. doi: 10.1104/pp.110.160663
- Hajdukiewicz P, Svab Z, Maliga P. 1994. The small, versatile pPZP family of Agrobacterium binary vectors for plant transformation. Plant Mol Biol. 25:989–994. doi: 10.1007/BF00014672
- He Y, Zhu M, Wang L, Wu J, Wang Q, Wang R, Zhao Y. 2018. Programmed self-elimination of the CRISPR/Cas9 construct greatly accelerates the isolation of edited and transgene-free rice plants. Mol Plant. 11:1210–1213. doi: 10.1016/j.molp.2018.05.005
- Hsiao YC, Hsu YF, Chen YC, Chang YL, Wang CS. 2016. A WD40 protein, AtGHS40, negatively modulates abscisic acid degrading and signaling genes during seedling growth under high glucose conditions. J Plant Res 129:1127–1140. doi: 10.1007/s10265-016-0849-5
- Jain BP, Pandey S. 2018. WD40 repeat proteins: signalling scaffold with diverse functions. Protein J. 37:391–406. doi: 10.1007/s10930-018-9785-7
- Janda L, Tichy P, Spízek J, Petrícek M. 1996. A deduced Thermomonospora curvata protein containing serine/threonine protein kinase and WD-repeat domains. J Bacteriol. 178:1487–1489. doi: 10.1128/JB.178.5.1487-1489.1996
- Jensen MK, Kjaersgaard T, Nielsen MM, Galberg P, Petersen K, O’Shea C, Skriver K. 2010. The Arabidopsis thaliana NAC transcription factor family: structure-function relationships and determinants of ANAC019 stress signalling. Biochem J. 426:183–196. doi: 10.1042/BJ20091234
- Jung C, Seo JS, Han SW, Koo YJ, Kim CH, Song SI, Nahm BH, Choi YD, Cheong J-J. 2008. Overexpression of AtMYB44 enhances stomatal closure to confer abiotic stress tolerance in transgenic Arabidopsis. Plant Physiol. 146:623–635. doi: 10.1104/pp.107.110981
- Lee JH, Terzaghi W, Deng XW. 2011. DWA3, an Arabidopsis DWD protein, acts as a negative regulator in ABA signal transduction. Plant Sci. 180:352–357. doi: 10.1016/j.plantsci.2010.10.008
- Lee JH, Yoon HJ, Terzaghi W, Martinez C, Dai M, Li J, Byun MO, Deng XW. 2010. DWA1 and DWA2, two Arabidopsis DWD protein components of CUL4-based E3 ligases, act together as negative regulators in ABA signal transduction. Plant Cell. 22:1716–1732. doi: 10.1105/tpc.109.073783
- Lee YJ, Kim DH, Kim Y-W, Hwang I. 2001. Identification of a signal that distinguishes between the chloroplast outer envelope membrane and the endomembrane system in vivo. Plant Cell. 13:2175–2190. doi: 10.1105/tpc.010232
- Liu H, Stone SL. 2010. Abscisic acid increases Arabidopsis ABI5 transcription factor levels by promoting KEG E3 ligase self-ubiquitination and proteasomal degradation. Plant Cell. 22:2630–2641. doi: 10.1105/tpc.110.076075
- Liu S, Hu Q, Luo S, Yang X, Wang X, Wang S. 2015. Expression of wild-type PtrIAA14.1, a polpar Aux/IAA gene causes morphological changes in Arabidopsis. Front Plant Sci. 6:388.
- Liu WC, Zheng SQ, Yu ZD, Gao X, Shen R, Lu YT. 2018. WD40-REPEAT 5a represses root meristem growth by suppressing auxin synthesis through changes of nitric oxide accumulation in Arabidopsis. Plant J. 93:883–893. doi: 10.1111/tpj.13816
- Lu HP, Liu SM, Xu SL, Chen WY, Zhou X, Tan YY, Huang JZ, Shu QY. 2017. CRISPR-S: an active interference element for a rapid and inexpensive selection of genome-edited, transgene-free rice plants. Plant Biotechnol. J. 15:1371–1373. doi: 10.1111/pbi.12788
- Ma Q, Xia Z, Cai Z, Li L, Cheng Y, Liu J, Nian H. 2019. GmWRKY16 enhances drought and salt tolerance through an ABA-mediated pathway in Arabidopsis thaliana. Front Plant Sci. 9(9):1979. doi: 10.3389/fpls.2018.01979
- Ma X, Zhang Q, Zhu Q, Liu W, Chen Y, Qiu R, Wang B, Yang Z, Li H, Lin Y, et al. 2015. A robust CRISPR/Cas9 system for convenient, high-efficiency multiplex genome editing in monocot and dicot plants. Mol Plant. 8:1274–1284. doi: 10.1016/j.molp.2015.04.007
- Ma Y, Szostkiewicz I, Korte A, Moes D, Yang Y, Christmann A, Grill E. 2009. Regulators of PP2C phosphatase activity function as abscisic acid sensors. Science. 324:1064–1068.
- Marchler-Bauer A, Bo Y, Han L, He J, Lanczycki CJ, Lu S, Chitsaz F, Derbyshire MK, Geer RC, Gonzales NR, et al. 2017. CDD/SPARCLE: functional classification of proteins via subfamily domain architectures. Nucl Acids Res. 45:D200–D203. doi: 10.1093/nar/gkw1129
- Mehdi S, Derkacheva M, Ramström M, Kralemann L, Bergquist J, Hennig L. 2016. The WD40 domain protein MSI1 functions in a histone deacetylase complex to fine-tune abscisic acid signaling. Plant Cell. 28:42–54. doi: 10.1105/tpc.15.00763
- Neer EJ, Schmidt CJ, Nambudripad R, Smith TF. 1994. The ancient regulatory-protein family of WD-repeat proteins. Nature. 371:297–300. doi: 10.1038/371297a0
- Ouyang Y, Huang X, Lu Z, Yao J. 2012. Genomic survey, expression profile and co-expression network analysis of OsWD40 family in rice. BMC Genomics. 13:100. doi: 10.1186/1471-2164-13-100
- Park SY, Fung P, Nishimura N, Jensen DR, Fujii H, Zhao Y, Lumba S, Santiago J, Rodrigues A, Chow TF, et al. 2009. Abscisic acid inhibits type 2C protein phosphatases via the PYR/PYL family of START proteins. Science. 324:1068–1071.
- Park SY, Peterson FC, Mosquna A, Yao J, Volkman BF, Cutler SR. 2015. Agrochemical control of plant water use using engineered abscisic acid receptors. Nature. 520:545–548. doi: 10.1038/nature14123
- Pesch M, Hülskamp M. 2004. Creating a two-dimensional pattern de novo during Arabidopsis trichome and root hair initiation. Curr Opin Genet Dev. 14:422–427. doi: 10.1016/j.gde.2004.06.007
- Ramsay NA, Glover BJ. 2005. MYB–bHLH–WD40 protein complex and the evolution of cellular diversity. Trends Plant Sci. 10:63–70. doi: 10.1016/j.tplants.2004.12.011
- Rodriguez PL, Leube MP, Grill E. 1998. Molecular cloning in Arabidopsis thaliana of a new protein phosphatase 2C (PP2C) with homology to ABI1 and ABI2. Plant Mol Biol. 38:879–883. doi: 10.1023/A:1006012218704
- Rushton DL, Tripathi P, Rabara RC, Lin J, Ringler P, Boken AK, Langum TJ, Smidt L, Boomsma DD, Emme NJ, et al. 2012. WRKY transcription factors: key components in abscisic acid signalling. Plant Biotechnol J. 10:2–11. doi: 10.1111/j.1467-7652.2011.00634.x
- Santiago J, Rodrigues A, Saez A, Rubio S, Antoni R, Dupeux F, Park SY, Marquez JA, Cutler SR, Rodriguez PL. 2009. Modulation of drought resistance by the abscisic acid receptor PYL5 through inhibition of clade A PP2Cs. Plant J. 60:575–588. doi: 10.1111/j.1365-313X.2009.03981.x
- Schiefelbein J. 2003. Cell-fate specification in the epidermis: a common patterning mechanism in the root and shoot. Curr Opin Plant Biol. 6:74–78. doi: 10.1016/S136952660200002X
- Shang Y, Yan L, Liu Z-Q, Cao Z, Mei C, Xin Q, Wu F-Q, Wang X-F, Du S-Y, Jiang T, et al. 2010. The Mg-chelatase H subunit of arabidopsis antagonizes a group of WRKY transcription repressors to relieve ABA-responsive genes of inhibition. Plant Cell. 22:1909–1935. doi: 10.1105/tpc.110.073874
- Song L, Huang SC, Wise A, Castanon R, Nery JR, Chen H, Watanabe M, Thomas J, Bar-Joseph Z, Ecker JR. 2016. A transcription factor hierarchy defines an environmental stress response network. Science. 354. pii: aag1550. doi: 10.1126/science.aag1550
- Stone SL, Williams LA, Farmer LM, Vierstra RD, Callis J. 2006. Keep on going, a RING E3 ligase essential for Arabidopsis growth and development, is involved in abscisic acid signaling. Plant Cell. 18:3415–3428. doi: 10.1105/tpc.106.046532
- Tian H, Chen S, Yang W, Wang T, Zheng K, Wang Y, Cheng Y, Zhang N, Liu S, Li D, et al. 2017. A novel family of transcription factors conserved in angiosperms is required for ABA signalling. Plant Cell Environ. 40:2958–2971. doi: 10.1111/pce.13058
- Tian H, Guo H, Dai X, Cheng Y, Zheng K, Wang X, Wang S. 2015. An ABA down-regulated bHLH transcription repressor gene, bHLH129 regulates root elongation and ABA response when overexpressed in Arabidopsis. Sci Rep. 5:17587. doi: 10.1038/srep17587
- Tiwari SB, Hagen G, Guilfoyle TJ. 2003. The roles of auxin response factor domains in auxin-responsive transcription. Plant Cell. 15:533–543. doi: 10.1105/tpc.008417
- Tran L-SP, Nakashima K, Sakuma Y, Simpson SD, Fujita Y, Maruyama K, Fujita M, Seki M, Shinozaki K, Yamaguchi-Shinozaki K. 2004. Isolation and functional analysis of Arabidopsis stress-inducible NAC transcription factors that bind to a drought-responsive cis-element in the early responsive to dehydration stress 1 promoter. Plant Cell. 16:2481–2498. doi: 10.1105/tpc.104.022699
- Umezawa T, Nakashima K, Miyakawa T, Kuromori T, Tanokura M, Shinozaki K, Yamaguchi-Shinozaki K. 2010. Molecular basis of the core regulatory network in aba responses: sensing, signaling and transport. Plant Cell Physiol. 51:1821–1839. doi: 10.1093/pcp/pcq156
- van Nocker S, Ludwig P. 2003. The WD-repeat protein superfamily in Arabidopsis: conservation and divergence in structure and function. BMC Genomics. 4:50. doi: 10.1186/1471-2164-4-50
- Walker AR, Davison PA, Bolognesi-Winfield AC, James CM, Srinivasan N, Blundell TL, Esch JJ, Marks MD, Gray JC. 1999. The TRANSPARENT TESTA GLABRA1 locus, which regulates trichome differentiation and anthocyanin biosynthesis in Arabidopsis, encodes a WD40 repeat protein. Plant Cell. 11:1337–1349. doi: 10.1105/tpc.11.7.1337
- Wang P, Xue L, Batelli G, Lee S, Hou Y-J, Van Oosten MJ, Zhang H, Tao WA, Zhu J-K. 2013. Quantitative phosphoproteomics identifies SnRK2 protein kinase substrates and reveals the effectors of abscisic acid action. Proc Natl Acad Sci USA. 110:11205–11210. doi: 10.1073/pnas.1308974110
- Wang S, Barron C, Schiefelbein J, Chen J-G. 2010. Distinct relationships between GLABRA2 and single-repeat R3 MYB transcription factors in the regulation of trichome and root hair patterning in Arabidopsis. New Phytol. 185:387–400. doi: 10.1111/j.1469-8137.2009.03067.x
- Wang S, Chang Y, Guo J, Chen JG. 2007. Arabidopsis Ovate family protein 1 is a transcriptional repressor that suppresses cell elongation. Plant J. 50:858–872. doi: 10.1111/j.1365-313X.2007.03096.x
- Wang S, Chen JG. 2014. Regulation of cell fate determination by single-repeat R3 MYB transcription factors in Arabidopsis. Front Plant Sci. 5:133.
- Wang S, Tiwari SB, Hagen G, Guilfoyle TJ. 2005. Auxin Response Factor7 restores the expression of auxin-responsive genes in mutant Arabidopsis leaf mesophyll protoplasts. Plant Cell. 17:1979–1993. doi: 10.1105/tpc.105.031096
- Wang X, Hou C, Zheng K, Li Q, Chen S, Wang S. 2017. Overexpression of ERF96, a small ethylene response factor gene enhances salt tolerance in Arabidopsis. Biol Plantarum. 61:693–701. doi: 10.1007/s10535-017-0734-7
- Wang X, Wang X, Hu Q, Dai X, Tian H, Zheng K, Wang X, Mao T, Chen JG, Wang S. 2015a. Characterization of an activation-tagged mutant uncovers a role of GLABRA2 in anthocyanin biosynthesis in Arabidopsis. Plant J. 83:300–311. doi: 10.1111/tpj.12887
- Wang ZP, Xing HL, Dong L, Zhang HY, Han CY, Wang XC, Chen QJ. 2015b. Egg cell-specific promoter-controlled CRPSPR/Cas9 efficiently generates homozygous mutants for multiple target genes in Arabidopsis in a single generation. Genome Biol. 16:144. doi: 10.1186/s13059-015-0715-0
- Xu ZY, Kim SY, Hyeon do Y, Kim DH, Dong T, Park Y, Jin JB, Joo SH, Kim SK, Hong JC, et al. 2013. The Arabidopsis NAC transcription factor ANAC096 cooperates with bZIP-type transcription factors in dehydration and osmotic stress responses. Plant Cell. 25:4708–4724. doi: 10.1105/tpc.113.119099
- Yamaguchi-Shinozaki K, Shinozaki K. 1993. The plant hormone abscisic acid mediates the drought-induced expression but not the seed-specific expression of rd22, a gene responsive to dehydration stress in Arabidopsis thaliana. Mol Gen Genet. 238:17–25. doi: 10.1007/BF00279525
- Yi C, Deng XW. 2005. COP1 – from plant photomorphogenesis to mammalian tumorigenesis. Trends Cell Biol. 15:618–625. doi: 10.1016/j.tcb.2005.09.007
- Yoshida T, Mogami J, Yamaguchi-Shinozaki K. 2014. ABA-dependent and ABA-independent signaling in response to osmotic stress in plants. Curr Opin Plant Biol. 21:133–139. doi: 10.1016/j.pbi.2014.07.009
- Zhao M, Morohashi K, Hatlestad G, Grotewold E, Lloyd A. 2008. The TTG1-bHLH-MYB complex controls trichome cell fate and patterning through direct targeting of regulatory loci. Development. 135:1991–1999. doi: 10.1242/dev.016873
- Zhao Y, Chan Z, Gao J, Xing L, Cao M, Yu C, Hu Y, You J, Shi H, Zhu Y, et al. 2016. ABA receptor PYL9 promotes drought resistance and leaf senescence. Proc Natl Acad Sci USA. 113:1949–1954. doi: 10.1073/pnas.1522840113
- Zheng K, Wang Y, Wang S. 2019. The non-DNA binding bHLH transcription factor PACLOBUTRAZOL RESISTANCES are involved in the regulation of ABA and salt responses in Arabidopsis. Plant Physiol Biochem. 139:239–245. doi: 10.1016/j.plaphy.2019.03.026
- Zhong R, Burk DH, Morrison WH, Ye Z-H. 2004. FRAGILE FIBER3, an Arabidopsis gene encoding a type II inositol polyphosphate 5-phosphatase, is required for secondary wall synthesis and actin organization in fiber cells. Plant Cell. 16:3242–3259. doi: 10.1105/tpc.104.027466