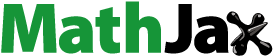
ABSTRACT
Salt stress is considered one of the major constraints limiting plant growth. Here, tomato plants were grown in hydroponic culture with two salt sodium chloride concentrations (S1 = 2.8 dS m−1 and S2 = 4.8 dS m−1). Under salt treatment, a significant decrease in chlorophyll content index and shoot and root dry weight were observed. We found that copper (Cu) was accumulated significantly in the shoot and sodium (Na) was significantly accumulated in the root. Furthermore, a significant nutrient imbalance indicated by a decrease in phosphorus (P), and potassium (K) uptake was measured. These decreases were accompanied by an increase in Na and Cu contents. A decrease in chlorophyll fluorescence yield was also observed indicating an inhibition at photosystem I acceptor sites. It seems that the downregulation of the electron transport between photosystem II and photosystem II under salt stress could be due to an imbalance in nutrient uptake.
Introduction
Salinity constraint is a major abiotic stress to plant health and soil quality as it affects the productivity of most crops. This serious problem has drastically increased in recent years mainly in arid and semi-arid areas (Munns Citation2002; Me¸trak et al. Citation2017; Bünemann et al. Citation2018). Salt stress influences a series of some major physiological processes such as photosynthesis, ion partitioning as well as Na+/K+ ratio, Reactive Oxygen Species (ROS) and hydraulic conductivity which affects the bioenergetic processes of electron transport chain (Kalaji and Pietkiewicz Citation1993; Neumann Citation1995; Steudle Citation2000; Munns Citation2002; Allakhverdiev and Murata Citation2008; Conde et al. Citation2011; Kalaji et al. Citation2011; Fakhrfeshani et al. Citation2015; Oukarroum et al. Citation2015; Almeida et al. Citation2017). Furthermore, salt stress seems to affect root anatomy and morphology parameters (Rivero et al. Citation2014; Robin et al. Citation2016).
Changes in morphological appearance in response to salinity stress are not enough to determine the effect and subsequently design the management strategies. It is therefore important to identify key physiological and biochemical factors for improving the salinity tolerance of plants (Munns and Tester Citation2008; Ahanger and Agarwal Citation2017; Ahanger et al. Citation2020). To date, three main mechanisms contributing to shoot tissue tolerance to salinity have been targeted: accumulation of Na+ in the vacuole, synthesis of compatible solutes and production of enzymes catalyzing detoxification of ROS. Increasing the abundance of vacuolar Na+/H+ antiporters (NHX), vacuolar H+ pyrophosphatases e.g. Pyrophosphate-energized vacuolar membrane proton pump 1, proteins involved in the synthesis of compatible solutes (such as proline and glycine betaine) and enzymes responsible for the detoxification of ROS had differing degrees of success in improving crop salinity tolerance.
Salt stress causes accumulation of ROS (Achard et al. Citation2008; Miller et al. Citation2010), which secondarily induced oxidative damage hampers the redox homeostasis resulting in declined photosynthetic efficiency (Miller et al. Citation2010; Xie et al. Citation2011; Khan et al. Citation2014), alters nitrogen and osmolyte metabolism (Ahanger and Agarwal Citation2017; Ahanger et al. Citation2020), mineral assimilation, phytohormone profile and expression of genes (Fallah et al. Citation2017; Ma et al. Citation2018). To avert the negative effects of salinity, plants have certain existing mechanisms like antioxidant system, osmotic adjustment and the efficient salt exclusion at root and vacuole level (Horie et al. Citation2012; Deinlein et al. Citation2014; Ahanger et al. Citation2020).
Most studies that show salinity-altered nutrient concentrations such as phosphorus (P) in plant tissues were conducted in soils. The interaction between salinity and nutrition of plants is highly dependent upon the plant species, plant developmental age, the composition and level of salinity and the concentration of macro and microelements in the substrate (Loupassaki et al. Citation2002; Shahriaripour et al. Citation2011). Plants synthesize proline, soluble sugars, glycine betaine, and other osmolytes to promote osmotic balance at the cellular level (Garg et al. Citation2002). Biosynthesis of osmo-protectants has been reported as an adaptive strategy to mediate salt stress. In addition to acting as osmo-solutes, they also act as N storage compounds and/or hydrophobic protectants for enzymes and cellular structures (Sami et al. Citation2016). It should also be noted that crops differ in their tolerance and ability to accumulate a high concentration of salts in their tissues. Thus, depending upon experiment conditions and selected plants, different results can be obtained. Salinity has been reported to affect phosphorus (P) mobility and bioavailability in the plant-soil system, and therefore root uptake (Grattan and Grieve Citation1992; Eisechie and Rodriguez Citation1999; Xie et al. Citation2017; Meena et al. Citation2018). In saline soils, mineral nutrients such as P availability are reduced because ionic strength effects, sorption processes in soil solution and low-solubility of Ca-P minerals in the soil. Since the solubility of P in saline soil solutions with high levels of Ca2+ is controlled by sorption processes on the solid phase of Ca-P minerals (Navarro et al. Citation2000).
Tomato plant is considered an important greenhouse crop in many semi-arid regions such as in the Mediterranean region where salinity has been considered a major constraint in crop production. We hypothesize that saline conditions affect nutrient uptake in hydroponic culture by tomato plants and alter the photosynthetic electron transport chain. In this work, physiological responses of tomato plants to two NaCl salt concentrations, S1 = 2.8 dS m−1 and S2 = 4.8 dS m−1, were investigated. We emphasized the change in chlorophyll a (Chl a) fluorescence, chlorophyll content index (CCI), root morphology parameters and accumulation of some mineral nutrients (Na, P, Cu, and K).
Materials and methods
Plant material and growth conditions
Tomato seeds (Lycopersicon esculentum var CAMPBELL 33 TECHNI) were germinated in the peat in darkness at a temperature-controlled (23°C). Similar size seedlings were placed in a culture chamber with a relative humidity between 70 and 80% and an ambient temperature of 23 ± 2°C during the day / 18 ± 2°C at night. The total photoperiod was 16 h/day. Plants were irrigated by distilled water. Twenty-three days after germination (DAG), seedlings were transferred to a plastic container (six seedlings per container) containing 4L of half-concentrated nutrient solution, without any treatment (to confer at plant adaptation period). The nutritive solution used Hoagland and Arnon (Citation1950). Nutrient solution (Table 1 in supplementary materials) consists of the following composition (ppm): N (270), K (234), Ca (200), Mg (49), Zn (0.48), Cu (0.02), B (0.45), Mn (0.5), Mo (0.01), and Fe (2.8). Phosphorus (P) was added to the nutrient solution as KH2PO4 at a concentration of 31 ppm P. Six days later, seedlings were exposed to two salinity (S) treatments (S1 = 2.8 dS m−1 and S2 = 4.8 dS m−1).
The pH of the solution was daily monitored and adjusted to 5.5 with either H2SO4 or KOH supply. The nutrient solution in each container was changed weekly. The containers were completely randomized and re-positioned weekly to minimize environmental effects. The growth continued for 45 days after germination or for 15 days-treatment and chlorophyll a fluorescence and some related fluorescence parameters, chlorophyll content index, root morphology, dry weight, and mineral content were analysed. Electrical Conductivity Meter (EC meter) was used to measure the salinity of the nutrient solution.
Leaf chlorophyll a fluorescence
Tomato plants kept in dark for 15 min before the measurements were started (for each treatment, 15 measurements were made by Handy PEA+, Hansatech instruments). The measurement consisted of a single strong 1 s light pulse (3000 μmol s−1 m−2 which is an excitation intensity to ensure closure of all Photosystem II (PSII) reaction centers) provided by an array of six light-emitting diodes (peak 650 nm). The Chl a fluorescence transients (ChlF) were digitized between 10 µs to 1 s by the instrument. From the fluorescence transient measured during the first second of illumination, following fluorescence parameters were calculated:
The maximum quantum yield of primary photochemistry
Fo (F20μs) and Fm correspond to the initial and maximum Chl a fluorescence. FV corresponds to the maximum variable Chl fluorescence;
Performance index (PI)
φPo corresponds to the efficiency by which an absorbed photon will be trapped by PSII reaction centers (RC).
The expression γo/(1−γo) is estimated by JIP-test as equal to the ratio of reaction centers and the absorbance (RC/ABS). Therefore: The expression ψo (=1−VJ) is the fraction of electrons transported beyond QA- per exciton trapped by the reaction centers (RC) of PSII. It is the probability that the energy of a trapped exciton is used for electron transport beyond QA. QA is the primary quinone electron acceptor of photosystem II.
The efficiency with which an electron can move from the reduced intersystem electron acceptors to the Photosystem I (PSI) end electron acceptors δRo (parameter related to electron transfer rate at PSI acceptor side):
Vt is defined as relative variable Chl a fluorescence at time t corresponding to (Ft −Fo)/(FM −Fo) and this expression can be taken as a measure of the fraction of the primary quinone electron acceptor of PSII in its reduced state [QA-/QA (total)].
The relative contribution of the I-P phase is expressed as delta VI = [(Fm−FI)/(Fm−Fo)] and it is related to photosystem I content (Oukarroum et al. Citation2009)
Chlorophyll content
The chlorophyll content index (CCI) was measured from the middle part of the leaf by using CL-O1 chlorophyll meter (Hansatech instruments).
Roots morphology parameters
Roots were carefully spread over a plastic box and scanned using an Epson Perfection LA2400 scanner. Data of total root length, root average diameter, volume and root surface area were acquired by processing the scanned root images using the WinRHIZO image analyzing system (Regent Instructions, Quebec, Canada).
Dry weight determinations and chemical analysis
Shoots and roots of control and treated plants were dried in an oven at 70°C for 2 days to determine dry weights. Also, elemental concentrations of Na, P, K, and Cu. Chemical elements were analyzed on a dry-weight basis using Inductively Coupled Plasma Optical Emission Spectrometry (Agilent 5110 ICP-OES, USA).
Statistical analysis
Data were subjected to analysis of variance (ANOVA) in SPSS 13.0 (SPSS Inc., USA) to examine the impacts of salinity on ChlF parameters, dry matter, chlorophyll content, root morphological traits, and macro and microelement concentrations.
Results
Understanding the physiological and biochemical mechanisms of salt stress is an important way to counter the negative effects of salinity and detect sensitive and tolerant traits in plants. Salt stress can, directly or indirectly, affect the photosynthetic activity of plant which is considered as one of the most important metabolic processes in plants. Reduction in photosynthetic activity alters also ChlF kinetics (Strasser et al. Citation2004 and references therein). Tomato leaves exhibit the typical ChlF transient OJIP during the first second of illumination in control and in salt treatments (). ChlF is used for understanding the reduction of the electron flow through PSII and PSI under two salt concentrations. The polyphasic fluorescence intensity increases from a minimum fluorescence intensity (Fo) to maximum fluorescence intensity (Fm) with two interval phases: the photochemical phase O-J and the thermal phase J-I-P (Strasser et al. Citation1995). We noticed here that the J-to-I-rise can be associated with the reduction of the PQ-pool (Schansker et al. Citation2003) and the I-to-P-rise with electron flow through PSI (Schreiber et al. Citation1989; Schansker et al. Citation2003).
Figure 1. A typical Chlorophyll a polyphasic fluorescence rise OJIP, exhibited by salt-stressed tomato plants. The transients are plotted on a logarithmic time scale. the marks indicate the time points used by the JIP-test for the calculation of structural and functional parameters. The signals are the fluorescence intensity Fo (at 20 μs); the fluorescence intensities FJ (at 2 ms) and FI (at 30 ms); the maximal fluorescence intensity, FP = FM. Each transient represents the mean of 15 measurments.
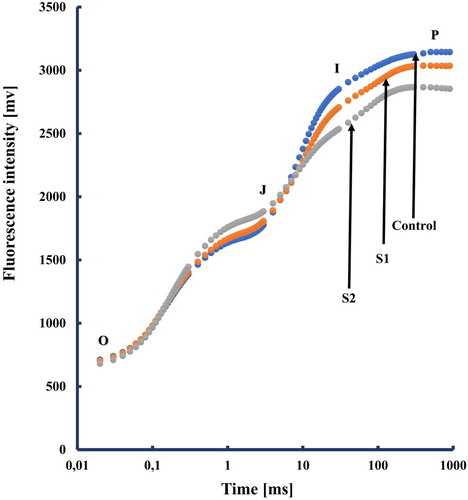
To further investigate how the electron transport chain is changed under salt treatment, the changes in PSII photochemistry were investigated in tomato plants kept in dark. According to JIP-test (Strasser et al. Citation2004; Oukarroum et al. Citation2007, Citation2015), the behavior of PSII under different stress conditions is quantified through functional and structural parameters derived from the fluorescence transients (O–J–I–P). To translate ChlF transient on quantitative fluorescence parameters, the maximum yield of primary photochemistry of PSII (Fv/Fm) was calculated and appeared to be not affected by salt treatment ().
Figure 2. Maximum quantum yield of primary photochemistry (Fv/Fm), Performance index (PI) and the efficiency with which an electron can move from the reduced intersystem electron acceptors to the PSI end electron acceptors δRo. Each value represents the mean of five independent experiments with about 15 repetitions.
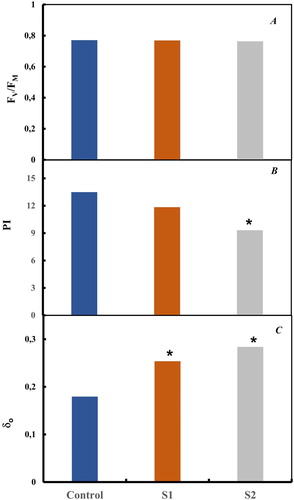
The effect of salt treatment on changes that occurred in the performance index (PI) in fully expanded tomato leaves is shown in . In this work, a significant decrease in PI parameter was significantly pronounced after 2 weeks of S2 treatment. The reduced electron transport chain resulted from PSII to PSI function could indicate the observed decrease in PI. However, and as an unexpected result, the efficiency with which an electron can move from the reduced intersystem electron acceptors to the PSI end-electron acceptors (δRo) showed a significant increase compared to control (P < 0.05) for salt treatment.
Exposure of tomato plants to salt treatment induced a significant decrease of Chl content index (). After 2 weeks of salt treatments, Chl content index decreased by 36% and 38% compared to control (p < 0.05) respectively at S1 and S2. Comparing Chl content index with the parameter ΔVI, a linear correlation was observed (). We noted here that the relative reduction in ΔVI has been reported to be related to a loss of PSI reaction centers. In a previous study, in drought-stressed barley plants, it has been shown that I-P-loss seems to be correlated to a loss of PS I reaction centers content as determined by 820 nm transmission measurements (Oukarroum et al. Citation2009).
Figure 3. Change in chlorophyll content index and correlation between delat VI and chlorophyll content index. Each value represents the mean of three independent experiments with about 15 repetitions.
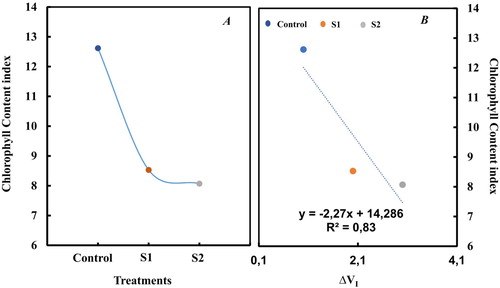
In this work and after two weeks of salt treatment, the morphology, length, surface, volume and diameter of the root were studied (). These characteristics showed a significant reduction compared to the control except for the diameter parameter for treatment S1 where a significant increase was noticed (Arif et al. Citation2019; Dinneny Citation2019; Terletskaya et al. Citation2019). In previous works, Céccoli et al. (Citation2011) and Neumann (Citation1995) reported also that salinity alters anatomical and morphology of roots such as diameter and length parameters.
Figure 4. Change in root length, root average diameter, volume and root surface in salt-stressed plants. Each value represents the mean of three independent experiments.
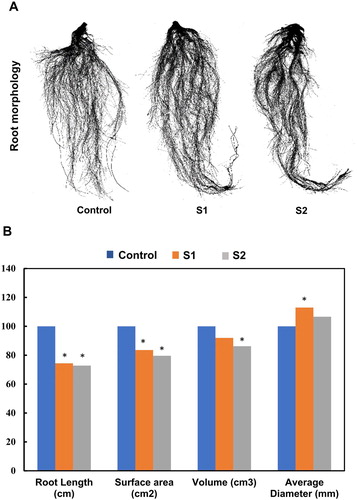
In , a significant decrease in the dry weight of the leaves, stem, and root was also observed for the highest salt treatment. It seems that physiological shoot growth is less influenced compared to those of root growth, this result is in concordance with the results obtained by Céccoli et al. (Citation2011). Our results showed that S2 treatment was the treatment that significantly affected plants.
Figure 5. Change in leaves (A), stem (B) and root weights (C) in salt-stressed plants. Each value represents the mean of three independent experiments.
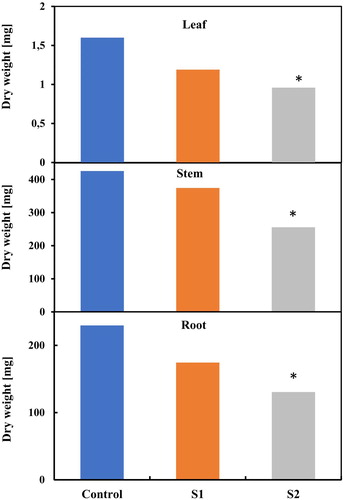
Plant mineral content affected by salt stress has been reported in previous studies (Hasana and Miyake Citation2017; Thu et al. Citation2017). Here, to determine how nutrients uptake was affected by the salt treatment in hydroponic culture, chemical elements Na, P, K and Cu were analyzed at the end of this experiment in the root and aerial part (). Our results show that significant accumulation of Na was observed for treatment S2 in the root whereas, after 2 weeks of salt treatment, it was in the aerial part at S1 where the accumulation of Na was observed.
Figure 6. Change in Na (A), P (B), K (C) and Cu (D) contents (ppm) in shoot and root of salt-stressed plants, stem and root weight in salt-stressed plants. Data were normalized to control. Each value represents the mean of three independent experiments.
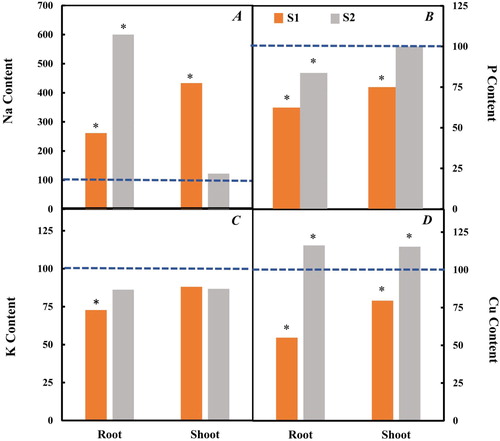
A different partitioning of Na+ ions between root and shoot was reported (Ochiai and Matoh Citation2002; Keisham et al. Citation2018). However, it was observed that P presents a significant decrease for the two treatments except during treatment S2 in the aerial part. It is well documented that P is a major element for the shoot and root growth and low-P uptake under salt stress could induce a reduction in biomass development (Navarro et al. Citation2001; Demiral Citation2017; Khan et al. Citation2018). Also, it has been reported that reduction in growth under salt stress could be attributed to a nutritional imbalance and excessive Na+ uptake (Hand et al. Citation2017; Isayenkov and Maathuis Citation2019).
Discussion
Plant growth is related to photosynthetic performance and changes in carbon economy under saline conditions (Webster et al. Citation2016; Asrar et al. Citation2017). Salinity stress often results in biomass reduction as a survival strategy of plants which is related with failure in carbon assimilation (Fall et al. Citation2017; Pompeiano et al. Citation2017). In such situations, plants distribute higher assimilated carbon to energy and maintenance rather than development of plant parts (Asrar et al. Citation2017). Photosynthetic rates are reported to be dependent to salinity concentration and duration of salt exposure (Fall et al. Citation2017; Pompeiano et al. Citation2017). A decrease in net CO2 assimilation is related to stomatal closure to regulate transpiration rate and water use efficiency (Liu et al. Citation2011). Long term salinity alters biochemical reactions (like Rubisco carboxylase/oxygenase activity and regeneration of Ribulose-1,5-bisphosphate (RuBP) and triose phosphates) that regulate gas-exchange. Moreover, it is reported that the major functional chloroplast protein complexes, i.e. PSI, PSII, ATP-synthase and Cytb6f, involved in harvesting light energy (Dekker and Boekema Citation2005) are altered under saline conditions along with Rubisco protein (Xu et al. Citation2010; Li et al. Citation2011).
In our work and under salt treatment, a decrease of J-I-P fluorescence yield was observed, and this change was found to be related to the downregulation of the electron transport chain (Stirbet and Govindjee Citation2011, Citation2012). These findings suggest a limitation of both donor and accepter-side of PSI (decrease in J-I-P yield). However, the maximum yield of primary photochemistry of PSII (Fv/Fm) appeared to be not affected by salt treatment and this result confirms the high stability of the potential PSII photochemical efficiency. We noted here that some previous studies demonstrate that salt stress may affect this fluorescence parameter Fv/Fm, this contradictory result appears to be depending on the studied plant species and the specific experimental conditions. That means that salt treatment in tomato leaves induced regulation of the intersystem electron transport between PSII and PSI and may indicate a cellular adaptation to counter the negative effect of salinity and ensure equilibrium in photosynthetic electron transport. These insights indicate the adaptation complexity of the plant adaptation to salt stress.
We noted here a decrease in the amount of K in the root and shoot part in salt-stressed plants. Interestingly, it has bed reported that salinity induced Na+ injury, which affects K+ uptake by root cells (Conde et al. Citation2011). Furthermore, Na+ accumulation inactivates both photosynthetic and respiratory electron transport (Allakhverdiev et al. Citation2000), which was observed in our work by a decrease in the photosynthetic performance index (PI) and I-P phase (loss of PSI reaction centers).
Our results were coherent with the literature published previously. However, It is in our interest to note that, nutrients P and K decrease with high salt concentration is accompanied by a significant increase in Na and Cu contents in root and shoot (Demiral Citation2017; Hand et al. Citation2017; Thu et al. Citation2017). It should also be noted that the quantity of Cu varies according to the plant parts of accumulation. Indeed, a significant reduction was observed in the root and an increase in the aerial part for the two salt treatments. In conclusion, the observed reduction in studied physiological parameters could be due to a negative change in nutrient uptake affect disequilibrium on ion homeostasis and then a cascade of changes in physiological and biochemical processes. Interaction of salinity and Cu and plant Cu uptake change might play a significant role in the response and adaptation of plants to salt stress.
Supplemental Material
Download MS Word (13.9 KB)Acknowledgements
A.L., and A.O conceptualized the lab studies; A.L., C.B., and R.N., designed the lab studies; H.K., A.S., M.G., performed the studies, analyzed the samples and data; A.L., Y.Z., and A.O., wrote the paper. All authors discussed the results and commented on the manuscript.
Disclosure statement
No potential conflict of interest was reported by the author(s).
Additional information
Funding
Notes on contributors
Aicha Loudari
Aicha Loudari is currently a PhD student in the SoilPhorLife project set up between the Mohamed VI Polytechnic University of Benguerir (Morocco) and the University of Liège, she is studying the interactions between salinity and availability of Phosphorus in the plant-soil system and seeks to develop a model of these interactions. Before that, she obtained her bachelor's degree in cellular and molecular biology in 2014 at the Moulay Ismail University in Meknes (Morocco) and her Master in Plant Biotechnology in 2016 at the Mohammed V University in Rabat (Morocco) where she worked on the in vitro culture of saffron and its bioactive molecules.
Chahinez Benadis
Chahinez Benadis was born and raised in ALGERIA. She holds a PhD on Biotechnology in 2015, from the faculty of science, Oran. Her PhD thesis focused on the valorization of rhizospheric microorganisms by producing biofertilizer to enhance legumes yield production. Broadly, her methodological research is oriented to develop sustainable agricultural system by introducing precision fertilization strategy. Winner of the 2015 L'Oréal-UNESCO For Women in Science Awards. Since 2019, she served as a post-doctoral researcher at Mohammed VI Polytechnic university. Recently, her researches are increasingly focused on plant agro-physiology and oriented towards the development of beneficial microorganisms for plants cultivation in order to select microorganisms and microalgae adapted to current biotic and abiotic constraints conditions.
Rachida Naciri
Rachida Naciri is a Researcher Engineer, she has a master's degree in Agronomy from National School of Agriculture of Meknes (ENA), Morocco in 2015. Since 2019, she joined Mohammed VI Polytechnic University as R&D research engineer in plant improvement and physiology. Her research interests include plant stress physiology, plant nutrition, and plan histological techniques.
Aziz Soulaimani
Aziz Soulaimani is a Researcher Engineer the Agricultural Innovation and Technology Transfer Center (A Network of Experimental Farms throughout Africa) at Mohammed VI Polytechnic University. He is responsible of the Plant and Soil analysis Laboratory and his research interests include soil, plant nutrition.
Youssef Zeroual
Dr. Youssef Zeroual, PhD in Microbiology and Biotechnology. He joined OCP in 2007 as Researcher in Biotechnology and Agronomy in R&D department and from 2019 he is in charge of soil management program in OCP Innovation department. His background is oriented to soil microbiology, interaction soil-plant- microbes, soil management, biofertilizers and Biostimulant , Biomass valorization.
Mohamed El Gharous
Dr. Mohamed El Gharous professor at the School of Agriculture and environment and Director of the Agricultural Innovation and Technology Transfer Center (A Network of Experimental Farms throughout Africa) at Mohammed VI Polytechnic University. Dr. Mohamed El Gharous received his bachelor's degree option experimental sciences in 1976. He received his engineering degree in horticulture in 1980 from IAV Hassan II, Rabat and his master and Ph-D in Agronomy and Soil Science from Oklahoma State University, United States of America, respectively in 1987 and 1991. Dr. Mohamed El Gharous also served as Director of the Regional Center for Agricultural Research Settat for 16 years, board member of the Faculty of Science and Technology of Settat, member of the Joint Committee Franco-Moroccan Research Projects for Development (PRAD) and Chairman of different non-governmental associations.
Hazem M. Kalaji
Professor Mohamed Hazem Kalaji graduated in 1983 from Agricultural Faculty, Aleppo University, Syria. In 1993, at Warsaw University of Life Sciences (SGGW), he received a PhD degree in Agricultural Sciences in the field of Agronomy with specialization of Plant Physiology and later on, in 2013, he obtained Doctor of Science degree (Habilitation) in agronomy. Both degrees were distinguished (Honour level) by SGGW. In 2015, Associate professor Kalaji got the position of Extraordinary Professor at the same university. Kalaji belongs to a group of worldwide recognized specialists in the field of plant physiology, in particular in the field of photosynthesis. The conducted research concerns: functioning, bioenergetics and efficiency of photosynthetic apparatus of plants, productivity of plant assimilation apparatus, plant reactions to abiotic and biotic stressors, chlorophyll fluorescence and gas exchange of plants. In recent years, he has been conducting research towards the creation of innovative solutions for agriculture and environmental protection based on the combination of Plant talk and Machine Learning. As part of international contacts, he has established cooperation with several dozens of institutions and universities from around the world (e.g. India, China, Switzerland, USA, Brazil, Spain, Saudi Arabia, Italy and many others). Associate professor Kalaji reviewed several Polish and foreign postdoctoral and doctoral dissertations, over 700 national and international projects and over 100 scientific papers of international scientific journals. He is also the editor of several international journals. During 2012-2018, he was the most cited scientists in the field of chlorophyll fluorescence.
Abdallah Oukarroum
Dr. Abdallah Oukarroum is a plant physiologist with extensive experience with the study of the photosynthetic apparatus. During his master's degree at the University Ibn Zohr in Morocco. Through his Ph.D thesis, conducted at the University of Geneva in Switzerland, Abdallah Oukarroum acquired an extensive experience in study of alterations in photosynthetic apparatus of plants under environmental stress mainly drought, salt and heat stress. As well, he established two new indexes which rank plant varieties with respect to drought and heat tolerance. At University of Quebec in Montreal in Canada, Abdallah Oukarroum as a post-doc and as a Researcher/Lecturer studied inhibitory effects and bioaccumulation of metals and metallic nanoparticles at membrane and cellular level on aquatic plants. Currently Abdallah Oukarroum is associate professor at University Mohammed VI Polytechnic (UM6P) in Morocco. He is interested in the study of the physiological and biochemical responses to different abiotic stress of superior's plants (terrestrials and aquatic plants), microalga and lichens.
References
- Achard P, Renou JP, Berthomé R, Harberd NP, Genschik P. 2008. Plant DELLAs restrain growth and promote survival of adversity by reducing the levels of reactive oxygen species. Curr Biol. 18:656–660.
- Ahanger MA, Agarwal RM. 2017. Salinity stress induced alterations in antioxidant metabolism and nitrogen assimilation in wheat (Triticum aestivum L.) as influenced by potassium supplementation. Plant Physiol Biochem. 115:449–460.
- Ahanger MA, Mir RA, Alyemeni MN, Ahmad P. 2020. Combined effects of brassinosteroid and kinetin mitigates salinity stress in tomato through the modulation of antioxidant and osmolyte metabolism. Plant Physiol Biochem. 147:31–42.
- Allakhverdiev SI, Murata N. 2008. Salt stress inhibits photosystems II and I in cyanobacteria. Photosynth Res. doi:10.1007/s11120-008-9334-x.
- Allakhverdiev SI, Sakamoto A, Nishiyama Y, Murata N. 2000. Inactivation of photosystems I and II in response to osmotic stress in Synechococcus. Contribution of water channels. Plant Physiol. doi:10.1104/pp.122.4.1201.
- Almeida DM, Margarida Oliveira M, Saibo NJM. 2017. Regulation of Na+ and K+ homeostasis in plants: towards improved salt stress tolerance in crop plants. Genet Mol Biol. doi:10.1590/1678-4685-gmb-2016-0106.
- Arif MR, Islam MT, Robin AHK. 2019. Salinity stress alters root morphology and root hair traits in Brassica napus. Plants. doi:10.3390/plants8070192.
- Asrar H, Hussain T, Hadi SMS, Gul B, Nielsen BL, Khan MA. 2017. Salinity induced changes in light harvesting and carbon assimilating complexes of Desmostachya bipinnata (L.) Staph. Environ Exp Bot. 135:86–95.
- Bünemann EK, Bongiorno G, Bai Z, Creamer RE, De Deyn G, de Goede R, Fleskens L, Geissen V, Kuyper TW, Mäder P, et al. 2018. Soil quality – a critical review. Soil Biol Biochem. doi:10.1016/j.soilbio.2018.01.030.
- Céccoli G, Ramos JC, Ortega LI, Acosta JM, Perreta MG. 2011. Salinity induced anatomical and morphological changes in Chloris gayana Kunth roots. Biocell. 35(1):9–17.
- Conde A, Silva P, Agasse A, Conde C, Gerós H. 2011. Mannitol transport and mannitol dehydrogenase activities are coordinated in olea Europaea under salt and osmotic stresses. Plant Cell Physiol. doi:10.1093/pcp/pcr121.
- Deinlein U, Stephan AB, Horie T, Luo W, Xu G, Schroeder JI. 2014. Plant salt-tolerance mechanisms. Trends Plant Sci. 19(6):371–379.
- Dekker JP, Boekema EJ. 2005. Supramolecular organization of thylakoid membrane proteins in green plants. Biochim Biophys Acta. 1706:12–39.
- Demiral MA. 2017. Effect of salt stress on concentration of nitrogen and phosphorus in root and leaf of strawberry plant. Eur J Soil Sci. doi:10.18393/ejss.319198.
- Dinneny JR. 2019. Developmental responses to water and salinity in root systems. Annu Rev Cell Dev Biol. doi:10.1146/annurev-cellbio-100617-062949.
- Eisechie HA, Rodriguez V. 1999. Does salinity inhibit alfalfa leaf growth by reducing tissue concentration of essential mineral nutrients? J Agron Crop Sci. doi:10.1046/j.1439-037X.1999.00300.x.
- Fakhrfeshani M, Shahriari-Ahmadi F, Niazi A, Moshtaghi N, Zare-Mehrjerdi M. 2015. The effect of salinity stress on Na +, K+ concentration, Na+ /K+ ratio, electrolyte leakage and HKT expression profile in roots of Aeluropus littoralis. J Plant Mol Breed. 3(2):1–10.
- Fall F, Diouf D, Fall D. 2017. Growth and physiological responses of Sporobolus robustus kunth seedlings to salt stress. Arid Land Res Manag. 31(1):46–56.
- Fallah F, Nokhasi F, Ghaheri M. 2017. Effect of salinity on gene expression, morphological and biochemical characteristics of Stevia rebaudiana Bertoni under in vitro conditions. Cell Mol Biol. 63(7):102–106.
- Garg AK, Kim JK, Owens TG, Ranwala AP, Do Choi Y, Kochian LV, Wu RJ. 2002. Trehalose accumulation in rice plants confers high tolerance levels to different abiotic stresses. Proc Natl Acad Sci U S A. 99(25):15898–15903.
- Grattan SR, Grieve CM. 1992. Mineral element acquisition and growth response of plants grown in saline environments. Agric Ecosyst Environ. doi:10.1016/0167-8809(92)90151-Z.
- Hand MJ, Taffouo VD, Nouck AE, Nyemene KPJ, Tonfack LB, Meguekam TL, Youmbi E. 2017. Effects of salt stress on plant growth, nutrient partitioning, chlorophyll content, leaf relative water content, accumulation of osmolytes and antioxidant compounds in pepper (Capsicum annuum L.) cultivars. Notul Bot Horti Agrobot Cluj Napoca. doi:10.15835/nbha45210928.
- Hasana R, Miyake H. 2017. Salinity stress alters nutrient uptake and causes the damage of root and leaf anatomy in maize. KnE Life Sci. doi:10.18502/kls.v3i4.708.
- Hoagland DR, Arnon DI. 1950. The water-culture method for growing plants without soil. Calif Agric Exp Stn Bull. 347:36–39.
- Horie T, Karahara I, Katsuhara M. 2012. Salinity tolerance mechanisms in glycophytes: an overview with the central focus on rice plants. Rice. 5(1):1–18.
- Isayenkov SV, Maathuis FJM. 2019. Plant salinity stress: many unanswered questions remain. Front Plant Sci. doi:10.3389/fpls.2019.00080.
- Kalaji HM, Govindjee, Bosa K, Kościelniak J, Zuk-Gołaszewska K. 2011. Effects of salt stress on photosystem II efficiency and CO2 assimilation of two Syrian barley landraces. Environ Exp Bot. doi:10.1016/j.envexpbot.2010.10.009.
- Kalaji MH, Pietkiewicz S. 1993. Salinity effects on plant growth and other physiological processes. Acta Physiol Plant. 143:89–124.
- Keisham M, Mukherjee S, Bhatla SC. 2018. Mechanisms of sodium transport in plants – progresses and challenges. Int J Mol Sci. doi:10.3390/ijms19030647.
- Khan MIR, Asgher M, Khan NA. 2014. Alleviation of salt-induced photosynthesis and growth inhibition by salicylic acid involves glycinebetaine and ethylene in mungbean (Vigna radiata L). Plant Physiol Biochem. 80:67–74.
- Khan MZ, Islam MA, Azom MG, Amin MS. 2018. Short term influence of salinity on uptake of phosphorus by Ipomoea aquatica. Int J Plant Soil Sci. doi:10.9734/ijpss/2018/44822.
- Li W, Zhang C, Lu Q, Wen X, Lu C. 2011. The combined effect of salt stress and heat shock on proteome profiling in Suaeda salsa. J Plant Physiol. 168:1743–1752.
- Liu Y, Du H, Wang K, Huang B, Wang Z. 2011. Differential photosynthetic responses to salinity stress between two perennial grass species contrasting in salinity tolerance. HortScience. 46(2):311–316.
- Loupassaki MH, Chartzoulakis KS, Digalaki NB, Androulakis II. 2002. Effects of salt stress on concentration of nitrogen, phosphorus, potassium, calcium, magnesium, and sodium in leaves, shoots, and roots of six olive cultivars. J Plant Nutr. doi:10.1081/PLN-120014707.
- Ma NL, Che Lah WA, Kadir A. 2018. Susceptibility and tolerance of rice crop to salt threat: physiological and metabolic inspections. PloS One. 13(2):e0192732.
- Meena MD, Narjary B, Sheoran P, Jat HS, Joshi PK, Chinchmalatpure AR, Yadav G, Yadav RK, Meena MK. 2018. Changes of phosphorus fractions in saline soil amended with municipal solid waste compost and mineral fertilizers in a mustard-pearl millet cropping system. Catena. doi:10.1016/j.catena.2017.09.002.
- Me¸trak M, Chachulski Ł, Navruzshoev D, Pawlikowski P, Rojan E, Sulwiński M, Suska-Malawska M. 2017. Nature’s patchwork: How water sources and soil salinity determine the distribution and structure of halophytic plant communities in arid environments of the Eastern Pamir. PLoS One. doi:10.1371/journal.pone.0174496.
- Miller G, Suzuki N, Ciftci-Yilmaz S, Mittler R. 2010. Reactive oxygen species homeostasis and signalling during drought and salinity stresses. Plant Cell Environ. 33:453–467.
- Munns R. 2002. Comparative physiology of salt and water stress. Plant Cell Environ. doi:10.1046/j.0016-8025.2001.00808.x.
- Munns R, Tester M. 2008. Mechanisms of salinity tolerance. Annu Rev Plant Biol. 59:651–681.
- Navarro JM, Botella MA, Cerdá A, Martinez V. 2001. Phosphorus uptake and translocation in salt-stressed melon plants. J Plant Physiol. doi:10.1078/0176-1617-00147.
- Navarro JM, Botella MÁ, Cerdá A, Martínez V. 2000. Effect of salinity x calcium interaction on cation balance in melon plants grown under two regimes of orthophosphate. J Plant Nutr. doi:10.1080/01904160009382076.
- Neumann PM. 1995. Inhibition of root growth by salinity stress: toxicity or an adaptive biophysical response? Struct Funct Roots. doi:10.1007/978-94-017-3101-0_39.
- Ochiai K, Matoh T. 2002. Characterization of the Na+ delivery from roots to shoots in rice under saline stress: excessive salt enhances apoplastic transport in rice plants. Soil Sci Plant Nutr. doi:10.1080/00380768.2002.10409214.
- Oukarroum A, Bussotti F, Goltsev V, Kalaji HM. 2015. Correlation between reactive oxygen species production and photochemistry of photosystems I and II in Lemna gibba L. plants under salt stress. Environ Exp Bot. doi:10.1016/j.envexpbot.2014.08.005.
- Oukarroum A, Madidi S, El Schansker G, Strasser RJ. 2007. Probing the responses of barley cultivars (Hordeum vulgare L.) by chlorophyll a fluorescence OLKJIP under drought stress and re-watering. Environ Exp Bot. doi:10.1016/j.envexpbot.2007.01.002.
- Oukarroum A, Schansker G, Strasser RJ. 2009. Drought stress effects on photosystem i content and photosystem II thermotolerance analyzed using Chl a fluorescence kinetics in barley varieties differing in their drought tolerance. Physiol Plant. doi:10.1111/j.1399-3054.2009.01273.x.
- Pompeiano A, Landi M, Meloni G, Vita F, Guglielminetti L, Guidi L. 2017. Allocation pattern, ion partitioning, and chlorophyll a fluorescence in Arundo donax L. in responses to salinity stress. Plant Biosyst. 151(4):613–622.
- Rivero RM, Mestre TC, Mittler R, Rubio F, Garcia-Sanchez F, Martinez V. 2014. The combined effect of salinity and heat reveals a specific physiological, biochemical and molecular response in tomato plants. Plant Cell Environ. doi:10.1111/pce.12199.
- Robin AHK, Matthew C, Uddin MJ, Bayazid KN. 2016. Salinity-induced reduction in root surface area and changes in major root and shoot traits at the phytomer level in wheat. J Exp Bot. doi:10.1093/jxb/erw064.
- Sami F, Yusuf M, Faizan M, Faraz A, Hayat S. 2016. Role of sugars under abiotic stress. Plant Physiol Biochem. 109:54–61.
- Schansker G, Srivastava A, Govindjee, Strasser RJ. 2003. Characterization of the 820-nm transmission signal paralleling the chlorophyll a fluorescence rise (OJIP) in pea leaves. Funct Plant Biol. doi:10.1071/FP03032.
- Schreiber U, Neubauer C, Klughammer C. 1989. Devices and methods for room-temperature fluorescence analysis. Philos Trans R Soc B. doi:10.1098/rstb.1989.0007.
- Shahriaripour R, Pour AT, Mozaffari V. 2011. Effects of salinity and soil phosphorus application on growth and chemical composition of pistachio seedlings. Commun Soil Sci Plant Anal. doi:10.1080/00103624.2011.535065.
- Steudle E. 2000. Water uptake by roots: effects of water deficit. J Exp Bot doi:10.1093/jexbot/51.350.1531.
- Stirbet A, Govindjee. 2011. On the relation between the Kautsky effect (chlorophyll a fluorescence induction) and photosystem II: basics and applications of the OJIP fluorescence transient. J Photochem Photobiol B Biol. doi:10.1016/j.jphotobiol.2010.12.010.
- Stirbet A, Govindjee. 2012. Chlorophyll a fluorescence induction: a personal perspective of the thermal phase, the J-I-P rise. Photosynth Res. doi:10.1007/s11120-012-9754-5.
- Strasser RJ, Srivastava A, Govindjee. 1995. Polyphasic chlorophyll a fluorescence transient in plants and cyanobacteria. Photochem Photobiol. doi:10.1111/j.1751-1097.1995.tb09240.x.
- Strasser RJ, Tsimilli-Michael M, Srivastava A. 2004. Analysis of the chlorophyll a fluorescence transient. doi:10.1007/978-1-4020-3218-9_12.
- Terletskaya N, Duisenbayeva U, Rysbekova A, Kurmanbayeva M, Blavachinskaya I. 2019. Architectural traits in response to salinity of wheat primary roots. Acta Physiol Plant. doi:10.1007/s11738-019-2948-0.
- Thu TTP, Yasui H, Yamakawa T. 2017. Effects of salt stress on plant growth characteristics and mineral content in diverse rice genotypes. Soil Sci Plant Nutr. doi:10.1080/00380768.2017.1323672.
- Webster RJ, Driever SM, Kromdijk J. 2016. High C3 photosynthetic capacity and high intrinsic water use efficiency underlies the high productivity of the bioenergy grass Arundo donax. Sci Rep. 6:20694.
- Xie W, Wu L, Wang J, Zhang Y, Ouyang Z. 2017. Effect of salinity on the transformation of wheat straw and microbial communities in a saline soil. Commun Soil Sci Plant Anal. doi:10.1080/00103624.2017.1373787.
- Xie YJ, Xu S, Han B, Wu MZ, Yuan XX, Han Y, Gu Q, Xu DK, Yang Q, Shen WB. 2011. Evidence of Arabidopsis salt acclimation induced by up-regulation of HY1 and the regulatory role of RbohD-derived reactive oxygen species synthesis. Plant J. 66:280–229.
- Xu C, Sibicky T, Huang B. 2010. Protein profile analysis of salt-responsive proteins in leaves and roots in two cultivars of creeping bentgrass differing in salinity tolerance. Plant Cell Rep. 29:595–615.