ABSTRACT
The use of nanoparticles in the appropriate portion to increase plant growth and development is an ongoing biological process. However, its exposure in an inconsiderable proportion become stressful and plant uses diverse regulatory mechanisms such as gene expression and miRNAs regulation to attain their cellular homeostasis. This study documented the influence of MgO NPs on genes and miRNAs expression, cell morphology, chlorophyll content, and physiological changes in Ananas comosus var. bracteatus at different treatment levels. It’s found that as MgO NPs exposed to the plant's increased, the average root length, average biomass, and average leaf length significantly decreased. A low concentration of MgO NPs (1 g/mL) increased the chlorophyll content of leaves, while 2 and 4 g/mL decreased it significantly. A 4 g/mL of MgO NPs increased the expression of miR-396 and miR-398 extremely, while repressed the expression of RHS12 and XTH genes significantly. Furthermore, the number of vascular bundles of root stele decreased obviously under 4 g/mL MgO NPs. 20 nm MgO NPs may have a destructive influence on the growth and development of Ananas comosus var. bracteatus by inhibiting chlorophyll.
Introduction
Nanoparticles are an ongoing prospering interdisciplinary part of research used in various fields (Ditta Citation2012). They can empower advanced applications in plants such as speed up a chemical reaction, pesticide exploitation, additive to feed, and proficient use as micronutrients (Srilatha Citation2011). Nanoparticles have prevalent physicochemical features such as high surface-to-volume proportion and size structure attributes (Khot et al. Citation2012). In effect, they are progressively applied in plants as an antibacterial agent, disinfectants, and micronutrients, which serve to improve quality plants (De et al. Citation2008). However, research reports have indicated that, once the nanoparticle is absorbed in an ambient environmental medium, the nanoparticles’ biotransformation may either improve or pose a menace to living organisms in that medium (Khan et al. Citation2019). Nanoparticles may not be as environmentally friendly as they are sometimes claimed or perceived to be (Monica and Cremonini Citation2009). There is still controversy about whether nanoparticles may serve as a threat to or somehow disrupt a plant’s biochemical processes (Song and Kim Citation2009). They must still be properly evaluated to determine their appropriate mode of application (Gericke and Pinches Citation2006). Research publications have reported on the uptake, influence, and buildup of different nanomaterials in plants, such as rice, bean, wheat, tomato (López-Vargas et al. Citation2018), watermelon (Dimkpa et al. Citation2018), and model plant tobacco (Liang et al. Citation2013). Nevertheless, knowledge in this area remains undeveloped. Moreover, the studies above reported the unclear influence of applying different nanomaterials on the plants (Mehmood Citation2018). For instance, the physiological reaction and nitrogen photoreduction was improved after rice plants exposed to 10 mg/L of TiO2 nanoparticles (Haider et al. Citation2017). Alternatively, after the treatment of Eruca sativa with 50 ppm of Ag NPs to evaluate phytotoxicity, the metabolic modification increased (Beyene et al. Citation2017). Moreover, no significant change in seed germination after the growth medium was treated with ZnO NPs (Maruthupandy et al. Citation2017). Interestingly, a nanoparticle's influence on a particular plant differs based on species types, environmental conditions, (Imada et al. Citation2016) and plant physiological features (Ma et al. Citation2010). Overall, around this is a critical quantity of the nanoparticle required to which the plant will either grow, respond to growth negatively, or the plant record it as stress (Seil and Webster Citation2012). Other results have generally confirmed that plants exposed to low nanoparticle concentration grew better than the control (Burklew et al. Citation2012). Others suffered lethal effects at higher concentrations (Liu Citation2006; Rai et al. Citation2018). However, there is a healthy relationship between metabolism, photosynthesis, plant growth, and nanoparticle concentration (Da Costa and Sharma Citation2016). Ananas comosus var. bracteatus is a perennial herbaceous monocot plant from Bromeliaceae’s family and subfamily of Bromelioideae. It is one of the five varieties of Ananas comosus (d’Eeckenbrugge et al. Citation2011). Its cultivation is commercially obtained in the tropics and subtropics (Aradhya et al. Citation1994). Ananas comosus var. bracteatus has bright, colorful leaves and attractive red fruit cultivated as an ornamental plant (Zucchi et al. Citation2019). Parts are of a high source of quality fiber (Souza et al. Citation2018). Over the years, Ananas comosus var. bracteatus was domesticated from the wild and has been studied to monitor the plant's bio-physiological growth (Hamad Citation2009). However, less is known about the possible effect of nanoparticles on its growth and environment. Moreover, the tissue-cultured shoots of Ananas comosus var. bracteatus are not strong and the leaves look yellow-green. It is important to make it stronger. Among the enormous types of nanomaterials, magnesium oxide nanoparticles (MgO NPs) can be nontoxic, easy to obtain, and useful to plants (Pugazhendhi et al. Citation2019). MgO NPs have better antibiotic resistance ability to reduce contamination and enhance plant tissue growth (Singh Citation2018). It has good influence on plant growth features such as improving photosynthesis ability to increase chlorophyll contents (Raliya et al. Citation2014), bacterial resistance (Cai, Chen, et al. Citation2018), and it may improve the quality of the tissue culture shoots of Ananas comosus var. bracteatus. MiR-156, miR-157, miR-159, miR-162, miR-169, miR-172, miR-396, miR-397, and miR-398 have already been identified as influencing plant growth and response to environmental stress (Plaksenkova et al. Citation2019). With that, we selected them to evaluate their regulatory expression level on Ananas comosus var. bracteatus exposed to MgO NPs stress. We also assayed stress-related genes, root hair specific, and cell wall genes to evaluate their expression level on Ananas comosus var. bracteatus exposed to MgO NPs. In this study, we measured the impact of MgO NPs delivered by root application on Ananas comosus var. bracteatus. We particularly focused on how the MgO NPs influence the growth and development of Ananas comosus var. bracteatus and the expression pattern of the related genes and miRNAs expression role during the treatment period. To our knowledge, this study is the first to provide a detailed assessment of the effects of nanoparticles on Ananas comosus var. bracteatus.
Materials and methods
Characterization of MgO nanoparticles
The MgO NP was synthesized at Beijing Innovative Microscopy Centre by a colloidal method at room temperature, according to Martínez-Mera et al. (Citation2007). The particle size is 20 nm.
Tissue culture plant growth, growth medium, and treatments
The base part of the complete green of Ananas comosus var. bracteatus ((A)) was first regenerated in Murashige and Skoog medium (MS, with sucrose 30 g/L and agarose 7 g/L, at pH 5.8). It was supplemented with 6-BA 3 mg/L, and NAA 2 mg/L to induce callus and the callus was regenerate into shoots ((B)). The regenerated plantlets with five to six leaves ((C)) were used as material for the whole experiments.
Figure 1. Plant materials of Ananas comosus var. bracteatus used in this study, (A) the base part of the tissue culture shoots used for callus induction, (B) callus with small shoots, and (C) shoot used for the experiment.
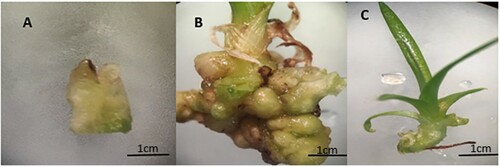
Subsequently, the shoots ((C)) were grown in MS medium for one week then transferred into a growth medium ((B)) with the following measurements of MgO NPs and control. The MgO NPs were suspended in 100 mL deionized water to obtain the final suspension concentration of 1 g/mL (T1), 2 g/ml (T2), and 4 g/ml (T3) for experimental use. Biases were curbed by measuring an equal quantity of medium with the designated concentrations of MgO NPs measured for each plant pot ((B)) in 2.5 in/6 cm standard square plant pot. 50 healthy of these plants were selected randomly for each treatment and placed under 16 h day/8 h night cycle at room temperature for six weeks.
Morphological parameters
A destructive measurement technique was used to obtain the physiological data for all the treatments. All the treatments were conducted with a minimum of three replicates. The growth features differentiation of the exposed plants; thus, leaf length, root length, growth rate, and biomass were determined. The leaf length was measured from the pointy part at one end of the leaf to where the leaf joins at the other end. The grid intersects technique was employed for the root length. Briefly, the root is traced on paper with the help of a ruler. The growth rate and the biomass were determined, according to Wang et al. (Citation2013) and Menzel et al. (Citation2009), respectively.
Chlorophyll content
The leaves of all the exposed plants were used for chlorophyll determination at the end of the sixth week. The chlorophyll extraction and detection were conducted as described (Raliya et al. Citation2015). The chlorophyll a, chlorophyll b, and total chlorophyll contents were assessed accordingly.
Microscope observation
Mature root, stem, and leaf samples from control and MgO NPs treated plants were prepared for anatomical observation under light microscopy by paraffin section. Samples were soaked in 70% FAA constituting, ethanol formaldehyde, and acetic acid in their respective compositions. They were then dehydrated, embedded in parafilm, and sliced. Finally, the samples were stained and observed under a microscope (OLYMPUS Model BX53F, DP80-TOKYO).
Selection of stress-related miRNAs
About nine miRNAs were selected for this work. Many miRNAs have been previously been reported to be involved in responses to stress in different plants. MiRNAs from Ananas comosus and other plants were reported to function in response to stress. However, some of these selected miRNAs were not found in Ananas comosus, so we looked for their target gene used in other plants and identified the genes in the Pineapple Genomics Database (http://pineapple.angiosperms.org/pineapple/html/index.html) and NCBI to predict these target’s and miRNA’s similar functions in Ananas comosus ().
Table 1. List of miRNA used in the research.
RNA extraction
After treated for six weeks, the plants were harvested, kept in liquid nitrogen, and stored in −80°C freezer for RNA extraction. MirVana miRNA Isolation Kit (Ambion, Austin, TX) was used to extract RNA according to the production protocol. The quality and quantity of the RNA were measured by Nanodrop ND-1000 (Nanodrop Technologies).
Analysis of the miRNAs using qRT-PCR
To quantify the miRNAs in Ananas comosus var. bracteatus, we used a stem-loop real-time PCR, miRNA qPCR Kit (SYBR Green), according to the manufacturer’s instructions. In this assay, the mature miRNAs were reverse transcribed into longer single-stranded cDNA sequences using a miRNA-specific stem-looped primer, and then, the qRT-PCR was then performed. In summary, a single-stranded miRNA cDNA sequence was generated from 1 mg of the total RNA collected from each treatment. The cDNA was generated with Mir- XTM miRNA First Strand cDNA Synthesis Reverse Transcription Kit with the miRNA-specific stem-looped RT primer provided. Tubulin was used as a reference gene to normalize the expression values on three replicates for each treatment and gene. Each biological replicate was strictly duplicated to limit the chances of error. The results were analyzed using ΔΔCT method. All graphs of parameters were made using Prism 5.
Data analysis
Statistical evaluation for all parameters was subjected to GenStat statistical analyzing software. The data were represented as the mean values ± SD (standard error). The significance values were tested for each treatment using a one-way ANOVA test with an LSD posthoc test. All treatments were tested with a 95% confidence interval.
Results and discussions
The effect of MgO NPs on the growth and development of Ananas comosus var. bracteatus
The potential effect of nanoparticles on the plant's biological processes is gaining ground. MgO NPs show a negative influence on the growth of Ananas comosus var. bracteatus plants (). After six weeks of growth with different concentrations of MgO NPs, the exposed plants show an apparent decrease in the root length, biomass, and leaf length. MgO NPs affected the leaf length significantly. In the control, the average leaf length was 25.8 mm, while the average leaf lengths of T1, T2, and T3 plants were 23.1, 19.3, and 16.00 mm, respectively ((A)). As the concentration of MgO NPs increases, the inhibiting effects on the root growth increased. For T1 and T2 plants, the average root length decreased to 14.8 and 12.0 mm, and significantly lower than that of the control (17.0 mm). The average root length of T3 exposed plants was only 0.6 mm, significantly lower than the control, T1, and T2 plants ((B)). When the MgO NPs concentration is higher than 2 g/mL, the inhibiting effects on the root growth increased apparently. The growth rate of the control group was represented as 98%. However, the average growth rate of the treatment groups was represented as 90%, 83%, and 80%, respectively. MgO nanoparticle supplementary had negative influences on biomass increase of the plants. The average biomass per plant for the control group was 18.6 mg, while the three treatment groups were 16.3, 14.8, and 13.1 mg, respectively ((D)). It is suggested that the application of MgO NPs inhibit the root growth of Ananas comosus var. bracteatus and this may result in the decrease of leaf growth and plant biomass. High concentration titanium dioxide and zinc oxide nanoparticles decreased root length and the physiological appearance of tomato (Raliya et al. Citation2014) Studies have shown that the primary mode related to plant introduced to MgO NPs stress is the root elongation and development (Ma et al. Citation2010). More importantly, the reduction in root length and leaves length results were derived from Sargassum wightti under an increase in MgO NPs concentration (Pugazhendhi et al. Citation2019).
Effect of MgO NPs on leaf chlorophyll content
The chlorophyll content of T1 plant leaves increased significantly from 0.714 to 0.976 mg/g compared with the control, similar in chlorophyll a with higher value in T1 than the control and other treatments. Moreover, the chlorophyll b of T2 increased comparing to the control and other treatment ((A)). Generally, after the exposure of Ananas comosus var. bracteatus to 2 and 4 g/mL of MgO NPs for six weeks, the Chl content decreased compared with the 1 g/mL and control. The results suggested that a low concentration of MgO NPs increased the biosynthesis of chlorophyll in leaves whiles a high concentration decreased the chlorophyll content ((A)). The Chl content was used as an indicator to evaluate the photosynthetic performance of plants. Magnesium (Mg) is an important component of the chlorophyll and photosynthesis process, low concentration supply of Mg has a positive effect but a relatively high concentration inhibited the biosynthesis of chlorophyll (Moynier and Fujii Citation2017). Chlorophyll is required during photo-assimilation, photophosphorylation with ATP formation (Cai, Lui, et al. Citation2018), and photo-oxidation in leaf tissues and oxygen reactive plant species (Moynier and Fujii Citation2017). The interaction of the NPs with photosystems can then enlarge and create root pores simultaneously (Martínez-Fernández et al. Citation2016). Low concentration supplementary of MgO NPs increased the light reactions of photosynthesis, and the enhanced photosynthetic process thereby increased the biomass in peanut (Cai, Chen, et al. Citation2018).
Effects of MgO NPs on the anatomical structure of Ananas comosus var. bracteatus
To assess the structural modifications in the Ananas comosus var. bracteatus plants after exposure to MgO NPs, the samples of roots, stems, and leaves were used for microscope observation. Since plants exposed to 4 g/mL MgO NPs for six weeks showed considerable changes in growth compared with the control and other treatments, it was selected to show the anatomical structure changes. In the leaves of the control ((A)) and T3 ((B)), the basic structure, such as upper epidermis, lower epidermis, water storage tissue, vascular bundles (xylem, phloem), and mesophylls were intacted ((A,B)). The cortex was normal in the stem of control and T3 treated plants, and parenchyma cells in the cortex regions are normal. The vascular bundles are distributed irregularly in the stele and circled by the pericycle. Adventitious roots were generating from the stele of the stem ((D)). A matured Ananas comosus var. bracteatus root structure showed a visible epidermal tissue, cortex, and stele. The stele is circled by pericycle cells, and the cell wall of the pericycle cells was thickened and deeply stained on three sides. Vascular bundles formed a circle in the center of the roots and the cell wall of the xylem cells was thicken and deeply stained. The number of vascular bundles in the exposed roots to 4 g/mL of MgO NPs reduced a lot, and the size of stele shranked apparently ((E,F)). A high concentration of MgO NPs showed negative effects on the root development of Ananas comosus var. bracteatus and reduced the number of vascular bundles and the size of stele apparently, which may result in the decrease of the growth of the plants (York et al. Citation1986). There was little or no changes in cell formation of the root, stem, or leaves treated with 250 µg/mL of MgO NPs in Nicotiana tabacum indicating the effects of MgO NPs on the anatomic structure is based on the concentration (Krishnaraj et al. Citation2012).
MgO NPs accumulation in Ananas comosus var. bracteatus tissues
The decrease of the average root length ((B)) and root vascular bundle numbers ((F)) suggested that MgO NPs affect root growth and development apparently. To reveal the absorption and transportation of MgO NPs, microscopic analysis of the leaf, stem and root of the control and T3 groups were done as described (Imada et al. Citation2016). No trace of MgO NPs content was observed in the leaf, stem, and root of control plants ((ACE). In the T3 treated plant tissues, almost no trace of MgO NPs content was observed in the leaves ((B)) and little traces were observed in the stem ((D)), while obvious traces of MgO NPs content was observed in the root ((F)). This suggested that the MgO NPs particles were absorbed by the roots and accumulated in the roots, a little was transported to the stem and almost no was transported to the leaves. The accumulation of MgO NPs in the roots affected the structure development and growth of the roots, which affected the absorption function of the roots. More dominant, the accumulation of MgO NPs observed in the lateral ends of the roots was higher ((D)). The accumulation of MgO NPs in the xylem and pith tissues of tomato leading to the outgrowth of parenchyma cells (Imada et al. Citation2016). The accumulation rate of the NPs by the plant roots was influenced by the properties of the NPs and the environmental conditions. The changes in the anatomical structure of the root system ((F)) and the inhibition of the growth and elongation of the roots ((B)) may be caused by the accumulation of the MgO NPs in the roots. Other studies, confirmed that NPs can accumulation in the wheat root then distribute through whole plant tissue (Krizkova et al. Citation2008) and the accumulation rate by the root (Schwab et al. Citation2016) can be influenced by the environmental condition (Moynier and Fujii Citation2017).
Changes in gene expression in MgO NPs exposed plants
In this study, we analyzed the expression levels of stress-related genes, root and cell development genes, such as APX6, ADH, and RHS12, XTH, respectively. The expression of APX6 was upregulated 1.72 fold under T1 and 2.71 fold under T2, and then slightly decreased to 2.44 fold under T3 compared to the control ((A)). The upregulated expression of the APX6 gene gave the plants the ability to withstand the nanoparticle stress suggested in Arabidopsis thaliana (Li et al. Citation2019). The expression of ADH upregulated to 1.36 fold and 3.59 fold under T1 and T2 respectively but decreases to 1.72 fold under T3 ((B)). For both stress genes APX6 and ADH, the fold change was statistically significant, with which the genes expression at 2 g/mL showed a massive increase compared to the control and the other treatments (). Previous studies have provided evidence that over-expression of these stress tolerance genes could aid plant growth under nanoparticle stress (Babitha et al. Citation2013). We can therefore predict that APX6 and ADH gene is helpful for the tolerance of Ananas comosus var. bracteatus plant growth under MgO NPs stress. XTH gene expression extremely increased 5.6 folds at 1 g/mL compared with the control, however at 2 and 4 g/mL, it decreased significantly at 1.8 and 2.4 folds, respectively ((C)). It can be observed that the transcript level of the XTH gene up-regulated under the MgO NPs stress, proposing the change in the root cell structure in (F), thereby change in Ananas comosus var. bracteatus morphological structure. The exposure to the NPs penetrates to the cell wall plasma membrane of the epidermal layer of the root and to the vascular tissues (Tang et al. Citation2018). It is suggested that the higher expression of xyloglucan under 1 g/mL might open up the primary and secondary cell wall to absorb more sunlight for the photosynthesis process hence increase in chlorophyll content ((A)). The expression of RHS12 was upregulated 4.2 folds in T1 and T2 but downregulated at 4 g/mL. More importantly, at 4g/ml of MgO NPs exposed plants, the expression of RHS12 expressed significantly lower at −1.5 fold than the control ((D)). These findings suggested that Ananas comosus var. bracteatus exposing to MgO NPs experienced a suppressing homeostatic phosphate enzymes action effect. Early response to nanoparticles in Arabidopsis inhibited root-hair development and reduction in root formation (García-Sánchez et al. Citation2015). Aside from these, the decreasing expression of RHS12 and XTH genes in Ananas comosus var. bracteatus under MgO NPs stress could be attributed to the accumulation of MgO NPs in the root. In Arabidopsis, the nanoparticle transported in the cell wall and found accumulated at plasmodesmata in the root thereby reduced the growth (Geisler-Lee et al. Citation2012).
MgO NPs changed microRNAS expression in Ananas comosus var. bracteatus
Plants specifically respond to environmental stress through their molecular pathways such as miRNAs. In plants, the miRNAs are post-transcriptional regulators and mediators of gene expression (Ding et al. Citation2013). The key mediatory factor of miRNAs in gene expression is the ability to respond to stress (Atkinson and Urwin Citation2012). At 1 g/mL/T1, all the tested miRNAs were upregulated except miR-159, and miR-162, which downregulated (). However, at T2 and T3 all the selected miRNAs upregulated except miR-396. The expression level of miR-159 and miR-162 were downregulated significantly in response to low (1 mg/L) MgO NPs exposure ((CD)). However, the expression of the remaining miRNAs listed increased in fold change at 4 mg/L MgO NPs treatment except for miR-397. Under 4 g/mL of MgO NPs, miR-398 and miR-396 showed greater fold change than other treatments with an average fold change in expression of 6.4 and 13.8 respectively ((GI)). In T2, miR-397 and miR-398 show a much greater change in fold than all the other miRNAs ((HI)), but miR-396 was downregulated compare to control. Many biological functions in plants were the results of miRNAs expression and stress responses. These miRNAs regulated the expression of a specific target gene by the demands for growth and responses to stress, such as drought (Ding et al. Citation2013), salinity (Xie et al. Citation2015), and heavy metals around the plant growth environment (Zhou et al. Citation2008). In this study, the miRNAs expression results show that miR-159, miR-162, miR-169, miR-397, and miR-398 significantly upregulated as MgO NPs concentration increased, thus the expression level was dependent on the concentration of the nanoparticles. At 1 g/mL of MgO NPs, miR-159, and miR-162 downregulated, but miR-172, miR-157, miR-398, and miR-156 upregulated with the greatest change in fold representing 4.2, 1.5, 1.6, and 1.9, respectively. These miRNAs high expression levels indicate there may be some molecules or pathways responding to MgO NPs stress in Ananas comosus var. bracteatus. MiR-159 is known to respond to drought during seed germination (Reyes and Chua Citation2007). Seeds imbibe water to reduce the abscisic acid level to help the seed take water to initiate germination (Ye et al. Citation2012). The presence of drought increased ABA level then germination is denied. miRNA-159 targeted two MYB transcription factors (MYB101, MYB33), and ABA expression is suppressed making the Arabidopsis seedlings hypersensitive (Reyes and Chua Citation2007). The decrease expression of miR-156 produced fewer leaves in Arabidopsis (Wu and Poethig Citation2006). The high expression level of miR-156 in all the MgO NPs treatments leads to less leaf length in var. bracteatus (). In miRNA biogenesis, miRNA-162 target Dicer-Like 1 protein during miRNAs maturation process, the Dicer protein cleave to pri-miRNA into pre-miRNA to become mature miRNA (Kidner and Martienssen Citation2005). Interestingly, miR-169 was downregulated to enhance drought tolerance in tomato stomata to reduce transpiration (Zhang et al. Citation2011). Overexpression of miR-172 altered leaf morphology and roots phloem development (Tang et al. Citation2018). The great changes of miR-172 expression may function in the alternation of the root morphology and structure at high MgO NPs stress.
Figure 7. Average miRNAs fold changes in expression in Ananas comosus var. bracteatus response to MgO NPs treatments (0/CTL, 1 g/mL/T1, 2 g/mL/T2, and 4 g/mL/T3). Values are mean of replicates with SD. Statistical significance is denoted by letters a–d. Changed in the letters denote statistical significance.
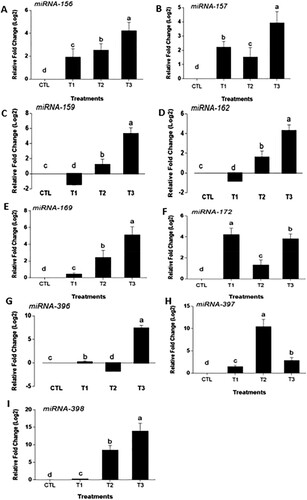
Moreover, miR-396 is known to function in cell proliferation and drought tolerance in Arabidopsis (Rodriguez et al. Citation2010) and tobacco (Yang and Yu Citation2009), respectively. In Arabidopsis, the expression of miR-396 and TCP4 increased, then the deceleration of cell proliferation decline in the seedlings’ growth and development (Rodriguez et al. Citation2010). Similarly, miR-396 was upregulated to drought stress in tobacco, leading to reduced leaves stomatal index. The upregulation of miR-396 in Ananas comosus var. bracteatus plants under 4 g/mL of MgO NPs stress may be attributed to the decrease of root cell proliferation ((F)), roots, and leaves length ((AB)). In many cases, such as photosynthetic electron transport in the chloroplast, drought stress, and inadequate nutrient response, miR-397 is beneficial in these areas in plant growth (Abdel-Ghany and Pilon Citation2008). Copper starvation decreased the growth rate while miR-397 was upregulated in Arabidopsis seedling (Burkhead et al. Citation2009). It can be deduced that miR-397 might play a similar role in Ananas comosus var. bracteatus, as it is upregulated under MgO NPs treatments. Furthermore, at 1 g of MgO NPs stress, the upregulation of miR-397 may function in the chlorophyll content increasing in T1 plants ((A)). miR-398 upregulated at 4 g/mL of MgO nanoparticles with 13.8 fold significantly higher than other treatments ((I)). Similar results in Arabidopsis of dynamic regulation of miR398 in response to ABA and salt stress (Jia et al. Citation2009). Similar results Arabidopsis that copper treatment increased plant biomass and miR-398 expression level (Zhu et al. Citation2011). It is suggested that the expression changes of these miRNAs may play important roles in the growth and development change of var. bracteatus under MgO NPs stress.
Conclusion
This study suggested that MgO NPs treatments had negative effects on the growth and development of Ananas comosus var. Bracteatus. The supplementary of MgO NPs downregulated the chlorophyll content of the leaves and decreased the growth of the leaf and root. The MgO NPs is absorbed by the roots and mostly accumulated in the roots after six weeks of culture. A high concentration of MgO NPs inhibited the vascular bundle formation of the roots. The expression of the resistance-related, root development-related miRNAs changed significantly under MgO NPs treatment specifically at 4 g/mL. We Future research can be performed to clarify the biochemical mechanisms of nanoparticle impact on plant chlorophyll degradation, cell degranulation, and several gene expression from the treated plants.
Acknowledgments
The authors are thankful to Sichuan Agricultural University, China, for providing support to this work. M. O. A conducted the research and wrote the manuscript, X. Z. conducted the chlorophyll experiment, M. M. review the manuscript. Y. Z. analyzed the results. J. L. referenced manuscript. H. H. planted the pants. J. L. prepared the MS media. H. Z. examined the samples under a microscope. L. F. computed the results of physiological changes. W. Y. measured the nanoparticles concentration. J. M. evaluated the manuscript for further corrections.
Disclosure statement
No potential conflict of interest was reported by the author(s).
Additional information
Funding
Notes on contributors
Mark Owusu Adjei
Mark Owusu Adjei, PhD Student in Plant physiology and molecular Research at the Sichuan Agricultural University, Chengdu-China.
Xuzixin Zhou
Xuzixin Zhou, PhD Student in Plant physiology and molecular Research at the Sichuan Agricultural University, Chengdu-China.
Meiqin Mao
Meiqin Mao, PhD Student in Plant physiology and molecular Research at the Sichuan Agricultural University, Chengdu-China.
Yanbin Xue
Yanbin Xue, PhD Student in Plant physiology and molecular Research at the Sichuan Agricultural University, Chengdu-China.
Jiawen Liu
Jiawen Liu, Master's Student in Plant physiology and molecular Research at the Sichuan Agricultural University, Chengdu-China.
Hao Hu
Hao Hu, Master's Student in Plant physiology and molecular Research at the Sichuan Agricultural University, Chengdu-China.
Jiaheng Luo
Jiaheng Luo, Master's Student in Plant physiology and molecular Research at the Sichuan Agricultural University, Chengdu-China.
Huiling Zhang
Huiling Zhang, Master's Student in Plant physiology and molecular Research at the Sichuan Agricultural University, Chengdu-China.
Wei Yang
Wei Yang, Master's Student in Plant physiology and molecular Research at the Sichuan Agricultural University, Chengdu-China.
Lijun Feng
Lijun Feng, Master's Student in Plant physiology and molecular Research at the Sichuan Agricultural University, Chengdu-China.
Jun Ma
Jun Ma, Professor in Plant Physiology and Molecular Researcher at the Sichuan Agricultural University.
References
- Abdel-Ghany SE, Pilon M. 2008. MicroRNA-mediated systemic down-regulation of copper protein expression in response to low copper availability in Arabidopsis. J Biol Chem. 283:15932–15945.
- Aradhya MK, Zee F, Manshardt RM. 1994. Isozyme variation in cultivated and wild pineapple. Euphytica. 79:87–99.
- Arshad M, Gruber MY, Hannoufa A. 2018. Transcriptome analysis of microRNA156 overexpression alfalfa roots under drought stress. Sci Rep. 8:1–13.
- Atkinson NJ, Urwin PE. 2012. The interaction of plant biotic and abiotic stresses: from genes to the field. J Exp Bot. 63:3523–3543.
- Avallone S, Guiraud J-P, Brillouet J-M, Teisson C. 2003. Enzymatic browning and biochemical alterations in black spots of pineapple [Ananas comosus (L.) Merr.]. Curr Microbiol. 47:0113–0118.
- Babitha K, Ramu S, Pruthvi V, Mahesh P, Nataraja KN, Udayakumar M. 2013. Co-expression of AtbHLH17 and AtWRKY28 confers resistance to abiotic stress in Arabidopsis. Transgenic Res. 22:327–341.
- Beyene HD, Werkneh AA, Bezabh HK, Ambaye TG. 2017. Synthesis paradigm and applications of silver nanoparticles (AgNPs), a review. Sustain Mater Technol. 13:18–23.
- Burkhead JL, Gogolin Reynolds KA, Abdel-Ghany SE, Cohu CM, Pilon M. 2009. Copper homeostasis. New Phytol. 182:799–816.
- Burklew CE, Ashlock J, Winfrey WB, Zhang B. 2012. Effects of aluminum oxide nanoparticles on the growth, development, and microRNA expression of tobacco (Nicotiana tabacum). Plos One. 7:e34783.
- Cai L, Chen J, Liu Z, Wang H, Yang H, Ding W. 2018. Magnesium oxide nanoparticles: effective agricultural antibacterial agent against Ralstonia solanacearum. Front Microbiol. 9:790.
- Cai L, Liu M, Liu Z, Yang H, Sun X, Chen J, Xiang S, Ding W. 2018. MgONPs can boost plant growth: Evidence from increased seedling growth, morpho-physiological activities, and Mg uptake in tobacco (Nicotiana tabacum L. Molecules. 23:3375.
- Da Costa M, Sharma P. 2016. Effect of copper oxide nanoparticles on growth, morphology, photosynthesis, and antioxidant response in Oryza sativa. Photosynthetica. 54:110–119.
- De M, Ghosh PS, Rotello VM. 2008. Applications of nanoparticles in biology. Adv Mater. 20:4225–4241.
- Debernardi JM, Rodriguez RE, Mecchia MA, Palatnik JF. 2012. Functional specialization of the plant miR396 regulatory network through distinct microRNA–target interactions. PLoS Genet. 8:e1002419.
- d’Eeckenbrugge GC, Sanewski GM, Smith MK, Duval M-F, Leal F. 2011. Ananas. In: Wild Crop Relatives: Genomic and Breeding Resources. 21–41.
- Dimkpa CO, Singh U, Adisa IO, Bindraban PS, Elmer WH, Gardea-Torresdey JL, White JC. 2018. Effects of manganese nanoparticle exposure on nutrient acquisition in wheat (Triticum aestivum L.). Agronomy. 8:158.
- Ding Y, Tao Y, Zhu C. 2013. Emerging roles of microRNAs in the mediation of drought stress response in plants. J Exp Bot. 64:3077–3086.
- Ding Y, Wang G, Fu Y, Zhu C. 2010. The role of miR398 in plant stress responses. Yi Chuan= Hereditas. 32:129–134.
- Ditta AJ. 2012. How helpful is nanotechnology in agriculture? Nanotechnology. 3:033002.
- Dong C-H, Pei HJ. 2014. Over-expression of miR397 improves plant tolerance to cold stress in Arabidopsis thaliana. Plant Biology. 57:209–217.
- García-Sánchez S, Bernales I, Cristobal S. 2015. Early response to nanoparticles in the Arabidopsis transcriptome compromises plant defence and root-hair development through salicylic acid signalling. BMC Genomics. 16:1–17.
- Geisler-Lee J, Wang Q, Yao Y, Zhang W, Geisler M, Li K, Huang Y, Chen Y, Kolmakov A, Ma X. 2012. Phytotoxicity, accumulation and transport of silver nanoparticles by Arabidopsis thaliana. Nanotoxicology. 7:323–337.
- Gericke M, Pinches A. 2006. Biological synthesis of metal nanoparticles. Hydrometallurgy. 83:132–140.
- Haider AJ, AL-Anbari RH, Kadhim GR, Salame CT. 2017. Exploring potential environmental applications of TiO2 nanoparticles. Energy Procedia. 119:332–345.
- Hamad AM. 2009. Micropropagation of pineapple (Ananas Comosus) cv. Smooth cayenne and Moris. University of Malaya, Malaysia.
- He J, Jiang Z, Gao L, You C, Ma X, Wang X, Xu X, Mo B, Chen X, Liu L. 2019. Genome-wide transcript and small RNA profiling reveals transcriptomic responses to heat stress. Plant Physiol. 181:609–629.
- Imada K, Sakai S, Kajihara H, Tanaka S, Ito S. 2016. Magnesium oxide nanoparticles induce systemic resistance in tomato against bacterial wilt disease. Plant Pathol. 4:551–560.
- Jia X, Wang W-X, Ren L, Chen Q-J, Mendu V, Willcut B, Dinkins R, Tang X, Tang G. 2009. Differential and dynamic regulation of miR398 in response to ABA and salt stress in Populustremula and Arabidopsisthaliana. Plant Mol Biol. 71:51–59.
- Jiu S, Leng X, Haider MS, Dong T, Guan L, Xie Z, Li X, Shangguan L, Fang J. 2019. Identification of copper (Cu) stress-responsive grapevine microRNAs and their target genes by high-throughput sequencing. R Soc Open Sci. 6:180735.
- Khan I, Saeed K, Khan I. 2019. Nanoparticles: properties, applications and toxicities. Arab J Chem. 12:908–931.
- Khot LR, Sankaran S, Maja JM, Ehsani R, Schuster EW. 2012. Applications of nanomaterials in agricultural production and crop protection: a review. Crop Prot. 35:64–70.
- Kidner CA, Martienssen RA. 2005. The developmental role of microRNA in plants. Curr Opin Plant Biol. 8:38–44.
- Krishnaraj C, Jagan E, Ramachandran R, Abirami S, Mohan N, Kalaichelvan P. 2012. Effect of biologically synthesized silver nanoparticles on Bacopa monnieri (Linn.) Wettst. plant growth metabolism. Process Biochem. 47:651–658.
- Krizkova S, Ryant P, Krystofova O, Adam V, Galiova M, Beklova M, Babula P, Kaiser J, Novotny K, Novotny J. 2008. Multi-instrumental analysis of tissues of sunflower plants treated with silver (I) ions–plants as bioindicators of environmental pollution. Sensors. 8:445–463.
- Li ZQ, Li JT, Bing J, Zhang GF. 2019. The role analysis of APX gene family in the growth and developmental processes and in response to abiotic stresses in Arabidopsis thaliana. Yi Chuan= Hereditas. 41:534–547.
- Liang T, Yin Q, Zhang Y, Wang B, Guo W, Wang J, Xie J. 2013. Effects of carbon nanoparticles application on the growth, physiological characteristics and nutrient accumulation in tobacco plants. J Front. 11:954–958.
- Liu W-T. 2006. Nanoparticles and their biological and environmental applications. J Biosci Bioeng. 102:1–7.
- López-Vargas ER, Ortega-Ortíz H, Cadenas-Pliego G, de Alba Romenus K, Cabrera de la Fuente M, Benavides-Mendoza A, Juárez-Maldonado A. 2018. Foliar application of copper nanoparticles increases the fruit quality and the content of bioactive compounds in tomatoes. Appl Sci. 8:1020.
- Ma Y, Kuang L, He X, Bai W, Ding Y, Zhang Z, Zhao Y, Chai Z. 2010. Effects of rare earth oxide nanoparticles on root elongation of plants. Chemosphere. 78:273–279.
- Martínez-Fernández D, Barroso D, Komárek M, Research P. 2016. Root water transport of Helianthus annuus L. under iron oxide nanoparticle exposure. Environ Sci Pollut Res. 23:1732–1741.
- Martínez-Mera I, Espinosa-Pesqueira M, Pérez-Hernández R, Arenas-Alatorre J. 2007. Synthesis of magnetite (Fe3O4) nanoparticles without surfactants at room temperature. Mater Lett. 61:4447–4451.
- Maruthupandy M, Zuo Y, Chen J-S, Song J-M, Niu H-L, Mao C-J, Zhang S-Y, Shen Y-H. 2017. Synthesis of metal oxide nanoparticles (CuO and ZnO NPs) via biological template and their optical sensor applications. Appl Surf Sci. 397:167–174.
- Mehmood A. 2018. Brief overview of the application of silver nanoparticles to improve growth of crop plants. IET Nanobiotechnol. 12:701–705.
- Menzel MI, Tittmann S, Buehler J, Preis S, Wolters N, Jahnke S, Walter A, Chlubek A, Leon A, Hermes N. 2009. Non-invasive determination of plant biomass with microwave resonators. Plant Cell Environ. 32:368–379.
- Monica RC, Cremonini R. 2009. Nanoparticles and higher plants. Caryologia. 62:161–165.
- Moradi K, Khalili F. 2018. Assessment of pattern expression of miR172 and miR169 in response to drought stress in Echinacea purpurea L. Biocatal Agric Biotechnol. 16:507–512.
- Moynier F, Fujii T. 2017. Theoretical isotopic fractionation of magnesium between chlorophylls. Sci Rep. 7:1–6.
- Nukuntornprakit O-A, Chanjirakul K, van Doorn WG, Siriphanich J. 2015. Chilling injury in pineapple fruit: fatty acid composition and antioxidant metabolism. Postharvest Biol Technol. 99:20–26.
- Ozhuner E, Eldem V, Ipek A, Okay S, Sakcali S, Zhang B, Boke H, Unver T. 2013. Boron stress responsive microRNAs and their targets in barley. PloS one. 8:e59543.
- Plaksenkova I, Jermaļonoka M, Bankovska L, Gavarāne I, Gerbreders V, Sledevskis E, Sniķeris J, Kokina I. 2019. Effects of Fe3O4 nanoparticle stress on the growth and development of rocket Eruca sativa. J Nanomater. 2019:2678247.
- Pugazhendhi A, Prabhu R, Muruganantham K, Shanmuganathan R, Natarajan SJ. 2019. Anticancer, antimicrobial and photocatalytic activities of green synthesized magnesium oxide nanoparticles (MgONPs) using aqueous extract of Sargassum wightii. J Photochem Photobiol. 190:86–97.
- Rai PK, Kumar V, Lee S, Raza N, Kim K-H, Ok YS, Tsang DC. 2018. Nanoparticle-plant interaction: implications in energy, environment, and agriculture. Environ Int. 119:1–19.
- Raliya R, Nair R, Chavalmane S, Wang W-N, Biswas P. 2015. Mechanistic evaluation of translocation and physiological impact of titanium dioxide and zinc oxide nanoparticles on the tomato (Solanum lycopersicum L.) plant. Metallomics. 7:1584–1594.
- Raliya R, Tarafdar J, Singh S, Gautam R, Choudhary K, Maurino VG, Saharan V. 2014. MgO nanoparticles biosynthesis and its effect on chlorophyll contents in the leaves of clusterbean (Cyamopsis tetragonoloba L.). Adv Sci Eng Med. 6:538–545.
- Reyes JL, Chua NH. 2007. ABA induction of miR159 controls transcript levels of two MYB factors during Arabidopsis seed germination. Plant J. 49:592–606.
- Rodriguez RE, Mecchia MA, Debernardi JM, Schommer C, Weigel D, Palatnik JF. 2010. Control of cell proliferation in Arabidopsis thaliana by microRNA miR396. Development. 137:103–112.
- Schwab F, Zhai G, Kern M, Turner A, Schnoor JL, Wiesner MR. 2016. Barriers, pathways and processes for uptake, translocation and accumulation of nanomaterials in plants – critical review. Nanotoxicology. 10:257–278.
- Seil JT, Webster T. 2012. Antimicrobial applications of nanotechnology: methods and literature. Int J Nanomed. 7:2767.
- Singh CR. 2018. Review on problems and its remedy in plant tissue culture. Asian J Biol Sci. 11:165–172.
- Song JY, Kim BS. 2009. Rapid biological synthesis of silver nanoparticles using plant leaf extracts. Bioprocess Biosyst Eng. 32:79.
- Souza FVD, de Souza EH, Neto ARS, Marconcini JM, de Assis SA. 2018. 10 Production for Other Uses. The Pineapple: Botany de Assis, Production and Uses 222.
- Srilatha BJ. 2011. Nanotechnology in agriculture. J Nanomed Nanotechnol. 2(7):5.
- Tang M, Bai X, Niu L-J, Chai X, Chen M-S, Xu Z-F. 2018. Mir172 regulates both vegetative and reproductive development in the perennial woody plant Jatropha Curcas. Plant Cell Physiol. 59:2549–2563.
- Wang C, Xiao B, Guo X, Wu S. 2013. A growth measuring approach for maize based on computer vision. In: International Conference on Computer and Computing Technologies in Agriculture. Springer; p. 183–189.
- Wu G, Park MY, Conway SR, Wang J-W, Weigel D, Poethig RS. 2009. The sequential action of miR156 and miR172 regulates developmental timing in Arabidopsis. Cell. 138:750–759.
- Wu G, Poethig RS. 2006. Temporal regulation of shoot development in Arabidopsis thaliana by miR156 and its target SPL3. Development. 133:3539–3547.
- Xie F, Wang Q, Sun R, Zhang B. 2015. Deep sequencing reveals important roles of microRNAs in response to drought and salinity stress in cotton. J Exp Bot. 66:789–804.
- Yang F, Yu D. 2009. Overexpression of Arabidopsis MiR396 enhances drought tolerance in transgenic tobacco plants. Acta Bot Yunnanica. 31:421–426.
- Ye N, Zhu G, Liu Y, Zhang A, Li Y, Liu R, Shi L, Jia L, Zhang J. 2012. Ascorbic acid and reactive oxygen species are involved in the inhibition of seed germination by abscisic acid in rice seeds. J Exp Bot. 63:1809–1822.
- Yew C, Kumar S. 2010. MicroRNA regulates gene expression during fruit development in pineapple. In VII International Pineapple Symposium 902; p. 177–184. doi:10.17660/ActaHortic.2011.902.17.
- York WS, Darvill AG, McNeil M, Stevenson TT, Albersheim P. 1986. Isolation and characterization of plant cell walls and cell wall components. Methods Enzymol. 118:3–40.
- Zhang X, Zou Z, Gong P, Zhang J, Ziaf K, Li H, Xiao F, Ye Z. 2011. Over-expression of microRNA169 confers enhanced drought tolerance to tomato. Biotechnol Lett. 33:403–409.
- Zhou ZS, Huang SQ, Yang ZM. 2008. Bioinformatic identification and expression analysis of new microRNAs from Medicago truncatula. Biochem Biophys Res Commun. 374:538–542.
- Zhu C, Ding Y, Liu H. 2011. Mir398 and plant stress responses. Physiol Plant. 143:1–9.
- Zucchi MR, da Silva MW, Sibov ST, Pires LL. 2019. Ornamental and landscape potential of a bromeliad native to the Cerrado. Ornam Hortic. 25:425–433.