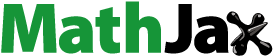
ABSTRACT
Rhizospheric bacteria may induce resistance to plant diseases. However, the underlying plant resistance mechanisms are unclear. We demonstrated the potential ability of the rhizobacterial strain Bacillus subtilis IAGS174 to elicit systemic resistance in tomato plants against Fusarium wilt pathogens. Comparative biochemical, histological, and molecular analyses were conducted to screen the differential responses between non-inoculated and B. subtilis IAGS174-inoculated tomato plants followed by pathogen challenge. B. subtilis IAGS174 had a significant inhibitory effect on disease development and reduced the disease index. B. subtilis IAGS174-primed plants exhibited significantly improved synthesis of total phenolics, flavonoids, and plant defense enzymes. Furthermore, priming increased the production of physical defense barriers including lignin. Additionally, RT-qPCR analysis revealed that disease resistance in bacteria-treated tomato plants was associated with increased expression levels of lignin-related and phenylpropanoid biosynthetic genes. Our findings support a positive role of B. subtilis IAGS174 in triggering immunity of tomato plants against a soil-borne disease.
Introduction
Tomato, an important solanaceous crop, is a major nutritional source of antioxidants and lycopene. Fusarium wilt diseases caused by the soil-inhabiting fungus, Fusarium oxysporum, result in major losses in many agricultural crops (Larkin and Fravel Citation1998). F. oxysporum has an extensive host range and damages many different horticultural crops. Fusarium wilt of tomato caused by F. oxysporum f. sp. lycopersici (FoL) is the major cause of tomato vascular wilt. Most tomato varieties are susceptible to this noxious disease. Breeding of vascular wilt-resistant varieties can offer some security against the spread of this disease. Nevertheless, the development of new virulent pathogenic strains is a persistent issue (Hooker Citation1981; Hartman and Fletcher Citation1991).
Plants and different microbes form beneficial relationships during the process of evolution. Biological control of plant diseases addresses the application of microbial agents or their properties to manage pathogenic activity (Abdallah et al. Citation2016). Biocontrol is considered a promising alternative approach that could improve strategies for managing soil-borne plant diseases (Gang et al. Citation2013). Bacillus strains are soil-inhabiting rhizobacteria that provide new approaches for managing plant diseases under conventional agriculture (Yasin and Ahmed Citation2016). These microbes provide resistance against a variety of plant diseases through different mechanisms. Some microbes compete for nutrients to hinder pathogen growth (Garbaye Citation1991), and some others can produce antagonistic compounds that inhibit pathogen growth (Chaurasia et al. Citation2005). Some Bacillus strains induce systemic resistance in assisted plants to safeguard against pathogenic infections (Choudhary and Johri Citation2009).
Plants may evolve a succession of complex physiochemical approaches to resist pathogenic entities. In many cases, phytohormones, including jasmonic acid (JA) and salicylic acid (SA), are responsible for the induction of systemic resistance in plants under stress (Segarra et al. Citation2006; Yuan et al. Citation2019). The provisional induction of elicitor-derived systemic resistance in plants requires less energy and food resources compared with the constitutive defense system (Thakur and Sohal Citation2013). Generally, roots are capable of diagnosing microbial elicitors and establishing the respective induced systemic resistance (ISR). Cyclic lipopeptides, siderophores, volatile organic compounds (VOCs), and lipopolysaccharides synthesized by microbes trigger ISR in assisted plants. The VOCs-synthesizing Bacillus subtilis GB03 may induce ISR in Arabidopsis plants through the ethylene-derived pathways (Sharifi and Ryu Citation2016). The cyclic lipopeptide-synthesizing B. subtilis may develop ISR in plants by triggering the JA- and SA-derived pathways (Ongena et al. Citation2007).
Stress alters the biosynthesis of defense-related biochemicals in plants (Mushtaq et al. Citation2020). Most resistant varieties accumulate higher quantities of phenols than susceptible varieties (Siddique et al. Citation2014). Higher quantities of phenolic compounds reduce the chances of infection by increasing cellular strength. Correspondingly, phenolics act as precursors of lignin and hence encourage cell wall lignification (Lyon et al. Citation1992). Lignin or lignin-like phenolic polymers are often synthesized in response to different stressors and act as physical barriers against pathogen invasion (Sattler and Funnell-Harris Citation2013). The phenylpropanoid pathway induces the synthesis of lignin and other phenolics, including phytoalexins. These phytochemicals have been shown to play crucial roles in plant defense (Dicko et al. Citation2005). Salicylic acid, which participates in plant protection, is also generated through the phenylpropanoid pathway. Currently, the protective role of lignin in induced resistance in plants has recently received extensive consideration.
Our previous study showed that B. subtilis IAGS174 can protect tomato plants from Fusarium wilt disease (Akram et al. Citation2013), but the detailed mechanisms underlying this resistance have not been studied. Thus, in the present study, biochemical, histological, cytochemical, and molecular examinations were performed to obtain a global overview of induced defense responses in tomato plants mediated by B. subtilis IAGS174 against Fusarium wilt disease. The most striking finding of this study is the re-modulation of lignin biosynthesis in tomato plants under the influence of a bacterial inducer. In addition, we discuss our findings in the context of inducible changes among the abundance of defense-related biochemicals, proteins, and histological aspects in tomato plants, with respect to the bacterial inducer and fungal wilt pathogen.
Materials and methods
Preparation of treatments
Seeds of tomato plants (Solanum lycopersicum Mill. cv. Finestar) were purchased from a commercial market based on its susceptibility against Fusarium wilt disease (Akram et al. Citation2014) and surfaced sterilized by adopting the standard sodium hypochlorite method. Seeds were sown in 15-inch diameter plastic pots containing sterilized commercial planting media (peat moss and white peat substrates mix, pH 5.8) purchased from Tref Jiffy, USA. The pathogenic inoculum (105 conidia/mL) of F. oxysporum f. sp. lycopersici, designated as FOL7, was prepared according to the method of Akram et al. (Citation2014). A 108 cfu/mL inoculum of Bacillus fortis IAGS174, a rhizobacterial strain, was prepared following Akram et al. (Citation2014). Briefly, B. fortis IAGS174 was grown in Luria Broth (LB) medium overnight. Media containing bacterial growth was centrifuged and pellet was resuspended in sterile distilled water to obtain the final bacterial concentration of 108 cfu/mL.
Evaluation of biocontrol agents under greenhouse conditions
Experimental design
To examine the protective effects of B. subtilis IAGS174 symbiosis on FOL7 attack and the defensive responses of tomato plants, a total of four treatments were examined (C, T1, T2, and T3): (1) C: control plants devoid of bacterial and pathogen inoculation; (T1): plants treated with pathogen only; (T2): tomato plants treated with bacterial inducer only; and (T3): tomato plants inoculated with both pathogen and bacterial inducer. For bacterial inducer inoculation, an aqueous formulation (50 mL) containing the inoculum of B. subtilis (108 cfu/mL) was poured into assigned plastic pots before sowing. Plants were incubated in a greenhouse at 20/25 ± 3°C (night/day), irrigated with modified half-strength Hoagland’s nutrient solution at two-day intervals, and LED growth lights adjusted to a 16 h photoperiod were used to supplement sunlight intensity in the greenhouse. The pathogen was inoculated as a conidial suspension ten days after emergence. Tomato plants were uprooted on the 7th day after pathogen inoculation, instantly rinsed with autoclaved deionized water, frozen in liquid nitrogen, and subsequently stored at −80 °C. For the investigation of physiological attributes, corresponding samples from five tomato plants were collected and combined as one sample (n = 5).
The disease index (DI) was assessed three weeks after pathogen inoculation. The following wilting scores were evaluated: 0 = no wilting symptom; 1 = less than 25% yellowing in plants; 2 = about 50% of plants become yellow-brown; and 3 = the entire plant becomes brown and dies (Epp Citation1987). For the estimation of DI from wilting scores, the following formula used by Cachinero et al. (Citation2002) was employed:
where ni = no of plants exhibiting wilting symptoms, si = value of the symptoms score, N = number of plants studied, and S = maximum value of the symptoms score.
Extraction of DNA and estimation of fungal biomass
Real-time quantitative PCR (qPCR) was used to quantify the extent of pathogenic colonization in the vascular tissues of diseased plants. After 3 weeks of pathogen inoculation, tomato plants from were uprooted. Shoots of tomato plants were cut at the ground level, and foliage was removed. Subsequently, a fine powder of stem frozen in liquid nitrogen was prepared. Complete genomic DNA was extracted using a Plant DNA Isolation Kit (Sigma-Aldrich, Darmstadt, Germany) according to the manufacturer’s instructions and quantified by spectrophotometry.
F. oxysporum f. sp. lycopersici-specific primer pairs, including sp1-2f and sp1-2pr (Table S1), were used to perform real-time qPCR analysis (Inami et al. Citation2010). Samples were equilibrated with the S. lycopersicum β-tubulin gene using the primer pair LeTUB-R and LeTUB-F (Table S1) Pantelides et al. (Citation2010). The detailed process of real-time qPCR analysis can be found in Antoniou et al. (Citation2017). A standard curve was drawn for the quantification of the pathogen DNA by making 10-fold dilutions of the PCR product (Antoniou et al. Citation2017).
Analysis of induced systemic resistance
Quantification of phenolic compounds, flavonoids, and resistance related enzymes
Total phenols were quantified using the Folin–Ciocalteu reagent method as described by (Zieslin and Ben-Zaken Citation1993). Briefly, ground leaf tissue (2 g) was vortexed with 10 mL methanol solution (80%), and extracts were mixed with 10 mL double distilled water in the presence of 500 mL Folin–Ciocalteu reagent. The optical density of the reaction solution was measured at 725 nm to evaluate the phenol content after comparison with the standard curve of gallic acid. For quantification of flavonoid content, the OD of the reaction mixture was measured at 510 nm and a calibration curve was drawn using a catechin standard curve, as suggested by Zhishen et al. (Citation1999)
For extraction of defense-related enzymes, foliage sample (0.8 g) was ground in liquid nitrogen in the presence of 6 mL of 100 mM ice-cold boric acid buffer (pH 8.8) to extract phenylalanine ammonia-lyase (PAL). In contrast, 50 mM sodium phosphate buffer (pH 7.8) was used to quantify peroxidase (POD) and polyphenol oxidase (PPO). The extraction mixture was subjected to centrifugation at 4000 × g for 20 min, and the clear supernatant was used as the enzymatic source. All procedures were performed at 4 °C. The variations in the quantities of PAL, POD, and PPO enzymes were measured by colorimetric assays, as suggested by Dickerson et al. (Citation1984), Hammerschmidt et al. (Citation1982), and Mayer et al. (Citation1966), respectively.
Quantification of SA and phenolics by GC/MS analysis
Endogenous SA and different phenolic acids were quantified from the leaf tissues of tomato plants by performing GC/MS analysis in separate analyses. Endogenous SA was extracted using the procedure of (Huang et al. Citation2015). Briefly, powdered leaf material (1 g) was vortexed with 10 mL of 80% chilled ethanol overnight in the presence of m-methylbenzoic acid as an internal standard (Ahmad et al. Citation2016). Then, 10 mL ethyl acetate was poured into the tube, mixed briefly, and centrifuged at 10000 rpm for 10 min at 4 °C. The collected supernatant was mixed with 0.2 g graphitized carbon black (GCB) and 0.6 g primary secondary amine (PSA), vortexed briefly, and the supernatant was obtained following centrifugation at 5000 rpm for 10 min. The obtained supernatant was dried using a stream of nitrogen and mixed with ethyl acetate for further examination. GC/MS investigation was performed on a Shimadzu GCMS-QP2010 Ultra (Shimadzu, Kyoto, Japan) GC/MS system fitted with a DB-5 capillary column. The analysis conditions can be seen in detail in Huang et al. (Citation2015). Bound phenolic acids were extracted from ground leaf tissues (0.5 g) in the presence of 40 mL of 62.5% aqueous methanol containing 1 g/L butylated hydroxytoluene (BHT) and derivatized by TMCS and BSTFA reagents as suggested by Proestos and Komaitis (Citation2013). The silylated samples were inserted into the same Shimadzu GCMS-QP2010 Ultra GC/MS system fitted with a DB-5 capillary column. The injector and detector were adjusted to 280 and 290 °C, respectively. GC was executed in splitless mode with a 60 s splitless time. A cocktail of in-house available authentic standards was used to quantify SA and bound phenolic acids.
Quantification of lignin, callose, and cellulose contents
Lignin content in the stems of tomato plants was quantified using the thioglycolic acid method according to Zhang et al. (Citation2010). The optical density (OD) of the extracted lignin solution was observed at 280 nm against a NaOH blank, and the lignin content was expressed as mg g− 1 DW. Different lignin structural components were quantified using a modified alkaline cupric (II) oxidation method ofGoñi and Montgomery (Citation2000). Lignin-associated compounds were converted into trimethylsilyl derivatives and identified by GC/MS. The different lignin structural groups were included as guaiacyl (G), syringyl (S), and p-hydroxyphenyl (H).
Cellulose quantification was performed using the acetic-nitric reagent method of (Scott Jr and Melvin Citation1953). Briefly, the dried stem powder was hydrolyzed with acetic-nitric reagent, extracted twice with acetone, and resuspended in 67% (v/v) H2SO4. Cellulose content was determined by measuring the OD at 625 nm. Callose content was determined as previously described (Köhle et al. Citation1985). In brief, stem powder (0.5 g) was extracted with 95% EtOH (1:5; w:v). After centrifugation, the pellet was dissolved in 0.4 mL of 1-M NaOH to solubilize the callose. After centrifugation, 0.8 mL of the supernatant was vortexed with 0.84 mL of 1-M HCl, 2.36 mL of 1.0-M glycine-NaOH, and 1.6 mL of 0.1% aniline blue. Following incubation, the OD was measured using a spectrofluorometer at an excitation wavelength of 400 nm and an emission wavelength of 485 nm.
Histological and cytochemical analysis of induced resistance
Stem samples were excised 5 cm from the ground level at 7 days post inoculation (dpi) from each treatment. Stem segments (2 cm) were stored in a solution of ethanol–acetic acid–formalin (90:5:5 v/v/v). Subsequently, stem tissues were diagonally cross-sectioned using a vibration microtome. The Wiesner reagent was used for staining of lignin (Pomar et al. Citation2002). Stem sections were stored in 1% (w/v) phloroglucinol solution containing 10.1 M hydrochloric acid-ethanol in phloroglucinol (75/25, v/v) for 10 min, followed by application of HCl (18%) for 5 min. Observations were made using a fluorescence microscope under bright field conditions. Transverse sections from the stem (400 µm thick), starting from one to four internodes, were used for the analyses of xylem vessel colonization and thickness analysis. The fungi were stained using lactophenol cotton blue staining (Shi et al. Citation1992) and the amount of xylem vessel colonization was evaluated quantitatively as described by Jiménez-Fernández et al. (Citation2013)
Gene expression analysis
The expression patterns of lignin biosynthesis, and defense-related genes, including PR1, PR2, PR3, PAL, LOX, and AOC, were investigated in treated plants using real-time PCR. Details of the primers used are provided in Table S1. Leaf samples were rapidly frozen in liquid nitrogen and ground into a fine powder. Total RNA isolation was performed using TRIzol reagent, according to the manufacturer instructions (Takara, Japan). Next, first-strand cDNA was produced using the EasyScript First-Strand cDNA Synthesis SuperMix kit (TransGen Biotech, Beijing, China). Real-time qPCR was performed in triplicate with SYBR Premix Dimer Eraser (Perfect Real Time) (Takara, Dalian, China) as described by Niu et al. (Citation2017). Actin was used as a reference gene to normalize the total quantity of cDNA in each reaction. The amplification efficiency was analyzed using standard curves, based on the 10-fold cDNA diluted series. The primers used for the gene expression studies are listed in Table S1.
Statistical analysis
There were five replications for all treatments, organized in a completely randomized design, and every trial was repeated twice. Statistical analysis was performed using SAS8.0 (SAS Institute, Cary, NC, USA). The data were examined using a one-way analysis of variance. Significant differences among treatment means were evaluated using Duncan’s multiple range test at P < 0.05.
Results
Potential of bacterial inducer on suppression of Fusarium wilt
Tomato plants were cultivated under symbiosis with a bacterial inducer and challenged with the Fusarium wilt pathogen. As shown by the pot experiment, a significant (P < 0.05) suppression effect was observed after treatment with a bacterial inducer (B. subtilis IAGS 174). The bacterial inducer decreased the Fusarium wilt disease index by up to 63.2% compared with the pathogen control plant (). Furthermore, real-time qPCR was performed to determine whether the reduction in disease index was due to the reduction in the colonization of the pathogen inside the vascular tissues of tomato plants. PCR analysis showed that fungal pathogens were present in the vascular tissues of plants treated with both pathogen and pathogen + bacterial inducer, but were significantly lower (58.3%) in tomato plants co-cultivated with the bacterial inducer ().
Figure 1. Fusarium wilt disease severity on tomato plants inoculated with B. subtilis IAGS174 and pathogen biomass quantification in planta. (A). Disease severity was calculated three weeks after pathogen inoculation. Vertical bars indicate the standard error of mean. Columns with different letters are statistically significantly different according to Duncan’s new multiple range test at P < 0.05. (B). Quantification of fungal biomass in planta using total plant DNA isolated from the aerial parts of tomato plants. Vertical bars indicate the standard error of mean. Columns with different letters are statistically significantly different according to Duncan’s new multiple range test at P < 0.05. (C). Fusarium wilt symptoms on tomato plants grown in the symbiosis of B. subtilis IAGS 174. Control = Non-treated control plants; T1= FoL; T2= B. subtilis IAGS174; T3= B. subtilis IAGS174 + FoL.
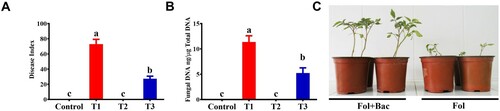
Analysis of induced systemic resistance
Quantification of total phenolics, flavonoids, and defense related enzymes
To understand how symbiosis of bacterial inducers make tomato plants resistant to soil-borne Fusarium wilt disease, we aimed to characterize the histological, biochemical, and molecular basis of induced resistance in tomato plants. Treatment with bacterial inducer + pathogen increased the total phenolic content by up to 2.3-fold compared with the control (). However, pathogen alone increased the total phenolic content by 1.4-fold compared with the untreated control (). Similarly, flavonoid production increased 1.9- and 1.3-fold in plants treated with bacterial inducer + pathogen and pathogen alone, respectively, when compared with the untreated control plants ().
Table 1. Effect of B. subtilis IAGS174 and F. oxysporum on production of different defense related compounds in tomato plants.
Similarly, the application of a bacterial inducer caused a significant increase in the quantities of different defense-related enzymes such as PO, PPO, and PAL. PO activity increased significantly up to 2.7- and 2.1-fold in tomato plants receiving bacterial inducer in combination with pathogen and pathogen alone, respectively, when compared with the control treatment (). The highest significant increase of 3.6-fold was found in the inedible production of PPO in bacterial inducer + pathogen-treated plants compared with the control treatment. PAL activity was 1.8-fold higher in the bacterial inducer + pathogen-treated plants than in the pathogen alone treatment (). Bacterial inducer alone significantly increased the inducible production of PO, PPO, and PAL. However, a pronounced effect was observed when plants received both microbes (bacterial inducer + pathogen) ().
Quantification of SA and phenolic acids by GC/MS analysis
Bacterial inducer inoculation significantly enhanced the concentration of SA and different bound phenolic acids in tomato plants ( and ). Treatment of tomato with bacterial inducer + pathogen increased SA content up to 3.1- and 1.7-fold when compared with untreated control and pathogen alone treatments, respectively ().
Figure 2. Effect of B. subtilis IAGS174 in quantities of different phenolic acids in tomato plants after F. oxysporum inoculation. Change in quantities of different bound phenolic acids was observed in the leaves of the tomato plants inoculated with bacterial inducer and Fusarium wilt pathogen. GC/MS analysis was performed after five days of pathogen application. Control = Non-treated control plants; T1= Bacterial inducer; T2= Pathogen control; T3= Bacterial inducer + pathogen. (*)= P>0.05; (**)= P>0.01 as governed by ANOVA.
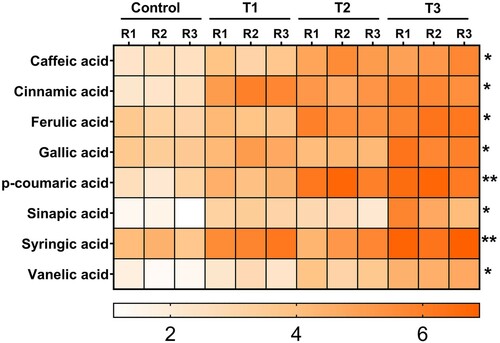
As seen for total phenolic and SA contents, accumulation of bound phenolic acids increased in bacterial inducer-and bacterial inducer + pathogen-treated tomato plants when compared with the control plants (). Bacterial inducers significantly (P > 0.05) increased the inducible production of bound phenolic acids (e.g. caffeic acid, cinnamic acid, ferulic acid, and gallic acid) in combination with pathogen challenge, whereas less pronounced differences were detected for these bound phenolic acids when bacterial inducer alone and pathogen alone treatments were compared with control plants ().
Histological and cytochemical analysis of induced resistance
Along with induction of biochemical defense, additional reinforcement of structural barriers in the form of callose, cellulose, and lignin deposition were observed in the bacterial inducer-treated plants when compared with the control (; and ). Callose production in tomato stems was increased by 37.3% and 11.7% in the bacterial inducer + pathogen-treated plants when compared with the untreated control and pathogen alone treatments, respectively (). In the same regard, bacterial inducer + pathogen-treated plants had 41% more cellulose content than plants treated with the pathogen alone ().
Figure 3. Chromatographic separations of lignin monomer subunits (A) and phenolic acids (B). 1= Vanillin, 2= Acetovanillone, 3= p-hydroxybenzoic acid, 4= Syringaldehyde, 5= Hydroxybenzaldehyde, 6= Hydroxyacetophenone, 7= Vanillic acid, 8= Syringic acid, 9= p-Coumaric acid, 10= IS, 11= Hydroxybenzoic acid, 12= Vanillic acid, 13 = p-Coumaric acid, 14= Gallic acid, 15= Ferulic acid, 16= Caffeic acid.
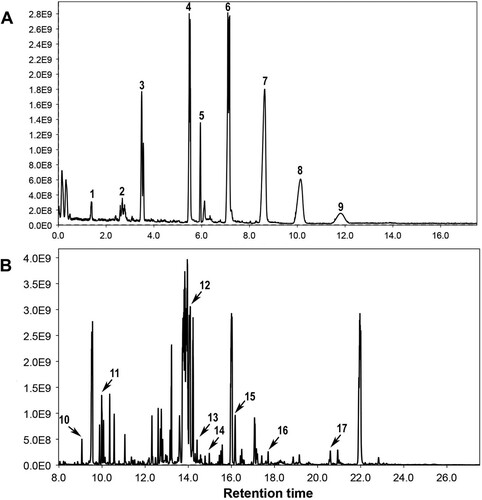
Figure 4. Effect of B. subtilis IAGS174 in quantities of lignin monomers subunits in tomato plants after F. oxysporum inoculation. Monomer subunits were quantified by performing GC/MS analysis five days post pathogen inoculation. Control = Non-treated control plants; T1= Pathogen control; T2= Bacterial inducer; T3= Bacterial inducer + pathogen. Vertical bars indicate the standard error of mean. Columns with different letters are statistically significantly different according to Duncan’s new multiple range test at P < 0.05.

Similarly, the total lignin content in the stems of the bacterial inducer + pathogen-treated plants was two-fold higher than that in the untreated control plants (). GC/MS analysis was performed to quantify the different lignin monomers/subunits released by the CuO method. A reference chromatogram showing the separation of the different lignin monomer subunits is shown in A. shows the inducible changes in the amounts of different lignin monomer subunits (G, H, and S) of lignin as affected by the bacterial elector and pathogen in either combination. In tomato plants treated with the bacterial inducer + pathogen, maximum increases in G, H, and S lignin units were observed in comparison to the non-treated control plants (). Pathogen and bacterial inoculation alone also significantly increased the production of different lignin units compared with the control tomato plants (). Histological observations showed dense localization of lignin around xylem vessels in stem sections of tomato plants receiving bacterial inducer + pathogen (). Pathogen alone also influenced the lignin deposition, and relatively more localization of lignin was observed in pathogen-treated plants than in non-treated control plants ().
Figure 5. Effect of B. subtilis IAGS174 on lignin deposition in stems of tomato plants after F. oxysporum inoculation. Lignin deposition was seen after staining with standard HCL-phloroglucinol method. A = Plants receiving B. subtilis IAGS174 followed by FoL inoculation; B = Plants receiving FoL alone; C = Plants receiving B. subtilis IAGS174 alone; D = Un-treated control plants. Scale bar shows 100 µm.
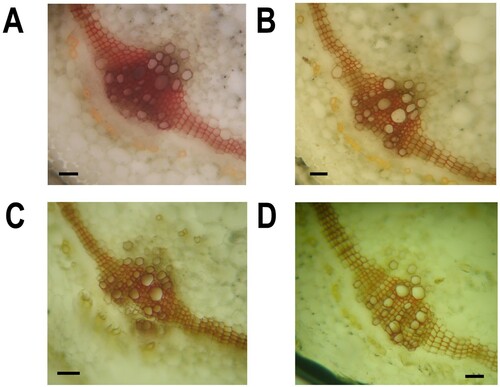
Furthermore, bacterial elicitation caused lesser vascular occlusion and increased thickness of the xylem vessels (). The degree of vascular occlusion significantly (P < 0.05) varied between the bacterial inducer + pathogen and pathogen alone treatments (). The presence of a bacterial inducer along with the pathogen reduced vascular occlusion by up to 39.6% compared with the pathogen control plants. In addition, bacterial inducer + pathogen treatment significantly increased (27.4%) the thickness of xylem vessels compared with pathogen alone treatment (). Interestingly, xylem vessel thickness in the bacterial colonization alone treatment was not significantly different from the pathogen treatment ().
Gene expression analysis
In addition to biochemical and histological responses, bacterial inducers caused significant (P< 0.05) increases in the expression levels of different defense-related genes involved in lignin biosynthesis and the phenylpropanoid pathway ().
Figure 7. Effect of B. subtilis IAGS174 on expression levels of lignin biosynthetic genes in tomato plants after F. oxysporum inoculation. RT-qPCR analysis was performed five days post pathogen inoculation. Control = Non-treated control plants; T1= Pathogen control; T2= Bacterial inducer; T3= Bacterial inducer + pathogen. Vertical bars indicate the standard error of mean. Columns with different letters are statistically significantly different according to Duncan’s new multiple range test at P < 0.05.
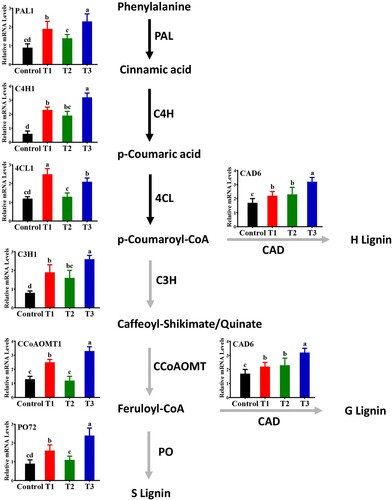
Quantitative data showed that combined treatment of bacterial inducer + pathogen increased expression levels of PAL1 (2.3-fold), C4H1 (3.1-fold), C3H1 (2.7-fold), PO72 (2.4-fold), and CCoAOMT1 (3.4-fold) in tomato plants compared with the untreated control (). The findings showed that, in response to bacterial inducer or pathogen infection alone, these defense-related genes were also upregulated, but to a lesser extent compared to the combined treatment of pathogen and bacterial inducer (). Notably, the expression level of 4CL1 was highly upregulated (2.7-fold) by pathogen alone compared with the non-treated control. These findings highlight that root treatment with bacterial inducers promotes systemically induced defense-related genes in tomato plants, and bacterial inducers differentially trigger transcript levels of defense-related genes.
Discussion
Many research trials have dictated the use of microbial inducers in suppressing pathogen infection or environmental stress. These studies involved plants exposed to biotic or abiotic stresses in the presence of microbial inducers capable of inducing systemic resistance. A few studies aimed to thoroughly understand the physiological or cytochemical basis, along with the biochemical markers of induced systemic resistance in tomato plants during F. oxysporum infection. Hence, we conducted a multidisciplinary analysis-based approach including biochemical, molecular, and histological investigations to gain an in-depth understanding of the inducible defense responses in tomato plants mediated by a bacterial inducer (B. subtilis IAGS 174) against Fusarium wilt disease.
Moreover, phenolic compounds serve as protective shields against pathogen colonization by imposing toxic environments against invading pathogens. Plants have efficient phenolic biosynthesis systems to avoid pathogen colonization and maintain plant health. Previous studies have shown that, under pathogen attack, certain beneficial microbes may augment phenylpropanoid biosynthesis systems via transcriptional induction of genes encoding defense-related enzymes.
B. subtilis IAGS 174-primed tomato plants showed a stronger accumulation of total phenolics and flavonoids following infection. These compounds exhibit antimicrobial and antioxidant properties and help plants to evade pathogenic infections (Kumar et al. Citation2020). The levels of some enzymes (PO, PPO, and PAL) that catalyze key steps in the phenylpropanoid pathway were significantly higher during pathogen infection in bacterial primed tomato plants compared to that in plants treated with pathogen alone. Phenylpropanoids act as precursors to a wide range of phenolic compounds and lignins (Dixon and Paiva Citation1995). Peroxidase triggers the polymerization of lignin (Quiroga et al. Citation2000). In contrast, polyphenoloxidase oxidizes phenolic compounds to toxic quinones, thereby restricting the growth of pathogens (Macheix et al. Citation1991). Phenylalanine ammonia lyase helps with lignin formation, thereby creating a physical barrier that restricts pathogen evasion (Pina and Errea Citation2008). All these findings support that bacterial inducers positively regulate systemic defense responses in tomato plants and establish signaling to up-regulate phytoalexin biosynthesis via the phenylpropanoid pathway.
When pathogens attack plants, in combination with biochemical and/or molecular responses, physical strengthening also contributes to restricting pathogen spread. Therefore, histological observations were performed qualitatively and quantitatively to assess the histological and cytochemical basis of induced resistance in tomato plants mediated by the bacterial inducer against wilt pathogen. During pathogen penetration attempts, the cell wall of host plants acts as a physical barrier through wall reinforcement via cellulose, callose, and lignin deposition. Cellulose acts as a structural barrier in the cell wall against invading pathogens (Kesten et al. Citation2017). Similarly, the accumulation of callose and lignin is a cell wall-strengthening strategy employed in plants against external stresses (Desprez et al. Citation2002). In this study, the contents of cellulose, callose, and lignin were significantly increased in tomato plants supplemented with a bacterial inducer, both in the presence and absence of wilt pathogen. Microscopic observations showed the localization of lignin in the area of the vascular bundles. Quantitative studies also supported these findings. Increased production of total lignin was observed in tomato plants under the influence of a bacterial inducer. Lignin subunits consisting of phenolic polymers frequently accumulate in plants under stress regimes (Sattler and Funnell-Harris Citation2013). The present GC/MS study showed amplified production of lignin subunits, which mainly consist of phenolic polymers, in tomato plants receiving bacterial inducers. These phenolic polymers might have played an important role in restricting pathogen growth inside the host plant, ultimately leading to a reduced disease index. The difference in the degree of xylem occlusion by F. oxysporum was significantly affected by the bacterial inducer. In our study, symbiosis of bacterial inducers significantly reduced xylem occlusion in tomato plants under wilt pathogen attack. The highest xylem occlusion was observed in tomato plants receiving the pathogen alone. In contrast, bacterial inducers also increased xylem vessel thickness in tomato plants. This can be attributed to the increased production of cellulose, callose, and lignin, which may have been deposited inside the cell walls of xylem vessels. Taken together, lignin, cellulose, and callose increased the mechanical protection of tomato plants against wilt pathogens and reinforced the vascular system for better translocation of water and minerals (Nawrocka et al. Citation2018). Increased deposition of lignin and callose has also been shown in previous studies investigating the protection of tomato and rice plants against vascular pathogens (Taheri and Tarighi Citation2012; Nawrocka et al. Citation2018).
As lignification is an important mechanism of disease resistance in plants, efforts have been made to understand the molecular mechanism underlying the increased lignification under the influence of bacterial inducers. From RT-qPCR analysis, genes that play key roles in the lignin biosynthesis pathway (PAL1, C4H1, 4CL1, C3H1, CCoMT1, and PO72) were strongly upregulated in primed plants, impairing Fusarium wilt resistance in tomato plants. These genes encode proteins involved in different intermediate steps of lignin biosynthesis. PAL proteins catalyze the leading committed phase in the phenylpropanoid pathway (Cass et al. Citation2015) and assist in lignin formation, as previously mentioned. These processes are also responsible for the synthesis of SA, which acts as an indispensable signaling molecule in the systemic resistance of plants (Mauch-Mani and Slusarenko Citation1996). C4H transforms trans-cinnamic acid to p-coumaric acid in the lignin synthesis pathway (Gravot et al. Citation2004). C3H participates in the monolignol pathway, is associated with reactive oxygen metabolism, and is involved in plant defense responses (Barros et al. Citation2019). In contrast, CAD is the last enzyme that participates in the biosynthetic pathway of lignin (Preisner et al. Citation2014). The up-regulation of these genes is consistent with the increased lignin biosynthesis and deposition in tomato plants, as observed in the histological analysis. Therefore, bacterial inducers can modulate lignin synthesis and participate in plant disease resistance.
It is evident that the induced resistance against plant pathogens involves a complex network of signaling molecules responsible for cross-communication in different metabolic pathways (Malinovsky et al. Citation2014). A previous GC/MS study was performed to observe variations in bound phenolic acids acting as precursor molecules for the biosynthesis of lignin and phytoalexins (e.g. stilbenes, coumarins, and flavonoids), which play important roles in plant defense (Weiergang et al. Citation1996). We observed a significant increase in the production of these phenolic acids under the influence of a bacterial inducer. In relation to plant defense, these compounds mayt act as signaling molecules that elicit systemic defense responses against invading pathogens. Similarly, bacterial inducers stimulate the accumulation of SA, which is a key component of plant defense pathways (Ruuhola et al. Citation2003). Overall, B. subtilis IAGS 174 may employ multiple defense mechanisms to synergistically restrict pathogens and control Fusarium wilt in tomato.
Conclusion
In conclusion, B. subtilis IAGS174 induced systemic resistance in tomato plants against Fusarium wilt of tomato through multiple mechanisms including biochemical, histological, and molecular re-modulations. B. subtilis IAGS174 triggered the production of defense-related enzymes, histological and cytochemical barriers, and different phenolic acids acting as precursor metabolites of the phenylpropanoid pathway. Furthermore, B. subtilis IAGS174 also induced the transcripts of lignin biosynthetic genes. Similarly, B. subtilis IAGS174 treated plants showed increased ratio of G to S (17:2) lignin subunits as G subunits are considered resistant to depolymerization. Thus, this bacterial inducer can act as a biological agent that can effectively control Fusarium wilt in tomato plants.
Disclosure statement
No potential conflict of interest was reported by the author(s).
Additional information
Funding
Notes on contributors
Waheed Akram
Conceived and designed experiments: WA, TA, and BA Performed experiments: WA, AA, SF, SA; analyzed data: NAY, WA, BA, and GL. Wrote and revised manuscript: WA, GL, and NAY.
Aqeel Ahmad
Conceived and designed experiments: WA, TA, and BA Performed experiments: WA, AA, SF, SA; analyzed data: NAY, WA, BA, and GL. Wrote and revised manuscript: WA, GL, and NAY.
Nasim Ahmad Yasin
Conceived and designed experiments: WA, TA, and BA Performed experiments: WA, AA, SF, SA; analyzed data: NAY, WA, BA, and GL. Wrote and revised manuscript: WA, GL, and NAY.
Tehmina Anjum
Conceived and designed experiments: WA, TA, and BA Performed experiments: WA, AA, SF, SA; analyzed data: NAY, WA, BA, and GL. Wrote and revised manuscript: WA, GL, and NAY.
Basharat Ali
Conceived and designed experiments: WA, TA, and BA Performed experiments: WA, AA, SF, SA; analyzed data: NAY, WA, BA, and GL. Wrote and revised manuscript: WA, GL, and NAY.
Sabin Fatima
Conceived and designed experiments: WA, TA, and BA Performed experiments: WA, AA, SF, SA; analyzed data: NAY, WA, BA, and GL. Wrote and revised manuscript: WA, GL, and NAY.
Shakeel Ahmed
Conceived and designed experiments: WA, TA, and BA Performed experiments: WA, AA, SF, SA; analyzed data: NAY, WA, BA, and GL. Wrote and revised manuscript: WA, GL, and NAY.
Mario Juan Simirgiotis
Conceived and designed experiments: WA, TA, and BA Performed experiments: WA, AA, SF, SA; analyzed data: NAY, WA, BA, and GL. Wrote and revised manuscript: WA, GL, and NAY.
Guihua Li
Conceived and designed experiments: WA, TA, and BA Performed experiments: WA, AA, SF, SA; analyzed data: NAY, WA, BA, and GL. Wrote and revised manuscript: WA, GL, and NAY.
References
- Abdallah RAB, Mokni-Tlili S, Nefzi A, Jabnoun-Khiareddine H, Daami-Remadi M. 2016. Biocontrol of Fusarium wilt and growth promotion of tomato plants using endophytic bacteria isolated from nicotiana glauca organs. Biol Control. 97:80–88.
- Ahmad N, Zuo Y, Lu X, Anwar F, Hameed S. 2016. Characterization of free and conjugated phenolic compounds in fruits of selected wild plants. Food Chem. 01/01:80–89.
- Akram W, Anjum T, Ahmad A. 2014. Basal susceptibility of tomato varieties against different isolates of Fusarium oxysporum f. sp. lycopersici. International Journal of Agriculture and Biology. 16:171–176.
- Akram W, Anjum T, Ali B, Ahmad A. 2013. Screening of native Bacillus strains to induce systemic resistance in tomato plants against Fusarium wilt in split root system and its field applications. International Journal of Agriculture and Biology. 15:1289–1294.
- Antoniou A, Tsolakidou M-D, Stringlis I, Pantelides I. 2017. Rhizosphere microbiome recruited from a suppressive compost improves plant fitness and increases protection against vascular wilt pathogens of tomato. Front Plant Sci. 11/01:2022.
- Barros J, Escamilla-Trevino L, Song L, Rao X, Serrani-Yarce JC, Palacios MD, Engle N, Choudhury FK, Tschaplinski TJ, Venables BJ, et al. 2019. 4-Coumarate 3-hydroxylase in the lignin biosynthesis pathway is a cytosolic ascorbate peroxidase. Nat Commun. 10:1–11.
- Cachinero JM, Hervas A, Jiménez-Díaz RM, Tena M. 2002. Plant defence reactions against fusarium wilt in chickpea induced by incompatible race 0 of Fusarium oxysporum f. sp. ciceris and nonhost isolates of F. oxysporum. Plant Pathol. 51:765–776.
- Cass CL, Peraldi A, Dowd PF, Mottiar Y, Santoro N, Karlen SD, Bukhman YV, Foster CE, Thrower N, Bruno LC, et al. 2015. Effects of PHENYLALANINE AMMONIA LYASE (PAL) knockdown on cell wall composition, biomass digestibility, and biotic and abiotic stress responses in brachypodium. J Exp Bot. 66:4317–4335.
- Chaurasia B, Pandey A, Palni LMS, Trivedi P, Kumar B, Colvin N. 2005. Diffusible and volatile compounds produced by an antagonistic Bacillus subtilis strain cause structural deformations in pathogenic fungi in vitro. Microbiol Res. 160:75–81.
- Choudhary DK, Johri BN. 2009. Interactions of Bacillus spp. and plants–with special reference to induced systemic resistance (ISR). Microbiol Res. 164:493–513.
- Desprez T, Vernhettes S, Fagard M, Refrégier G, Desnos T, Aletti E, Py N, Pelletier S, Höfte H. 2002. Resistance against herbicide isoxaben and cellulose deficiency caused by distinct mutations in same cellulose synthase isoform CESA6. Plant Physiol. 128:482–490.
- Dickerson DP, Pascholati SF, Hagerman AE, Butler LG, Nicholson RL. 1984. Phenylalanine ammonia-lyase and hydroxycinnamate: CoA ligase in maize mesocotyls inoculated with Helminthosporium maydis or Helminthosporium carbonum. Physiol Plant Pathol. 25:111–123.
- Dicko MH, Gruppen H, Barro C, Traoré AS, van Berkel WJH, Voragen AGJ. 2005. Impact of phenolic compounds and related enzymes in sorghum varieties for resistance and susceptibility to biotic and abiotic stresses. J Chem Ecol. 31:2671–2688.
- Dixon RA, Paiva NL. 1995. Stress-induced phenylpropanoid metabolism. Plant Cell. 7:1085.
- Epp D. 1987. Somaclonal variation in banana: a case study with Fusarium wilt. Banana and Plantain Breeding Strategies. 187:140–150.
- Gang G, Bizun W, Weihong M, Xiaofen L, Xiaolin Y, Chaohua Z, Jianhong M, Huicai Z. 2013. Biocontrol of Fusarium wilt of banana: key influence factors and strategies. African Journal of Microbiology Research. 7:4835–4843.
- Garbaye J. 1991. Biological interactions in the mycorrhizosphere. Experientia. 47:370–375.
- Goñi MA, Montgomery S. 2000. Alkaline CuO oxidation with a microwave digestion system: lignin analyses of geochemical samples. Anal Chem. 72:3116–3121.
- Gravot A, Larbat R, Hehn A, Lievre K, Gontier E, Goergen J-L, Bourgaud F. 2004. Cinnamic acid 4-hydroxylase mechanism-based inactivation by psoralen derivatives: cloning and characterization of a C4H from a psoralen producing plant—ruta graveolens—exhibiting low sensitivity to psoralen inactivation. Arch Biochem Biophys. 422:71–80.
- Hammerschmidt R, Nuckles EM, Kuć J. 1982. Association of enhanced peroxidase activity with induced systemic resistance of cucumber to colletotrichum lagenarium. Physiol Plant Pathol. 20:73–82.
- Hartman JR, Fletcher JT. 1991. Fusarium crown and root rot of tomatoes in the UK. Plant Pathol. 40:85–92.
- Hooker WJ. 1981. Compendium of potato diseases. St. Paul, MN: American Phytopathological Society.
- Huang Z-H, Wang Z-L, Shi B-L, Wei D, Chen J-X, Wang S-L, Gao B-J. 2015. Simultaneous determination of salicylic acid, jasmonic acid, methyl salicylate, and methyl jasmonate from ulmus pumila leaves by GC-MS. Int J Anal Chem. 2015:698630–698636.
- Inami K, Yoshioka C, Hirano Y, Kawabe M, Tsushima S, Teraoka T, Arie T. 2010. Real-time PCR for differential determination of the tomato wilt fungus, Fusarium oxysporum f. sp. lycopersici, and its races. J Gen Plant Pathol. 76:116–121.
- Jiménez-Fernández D, Landa BB, Kang S, Jiménez-Díaz RM, Navas-Cortés JA. 2013. Quantitative and microscopic assessment of compatible and incompatible interactions between chickpea cultivars and Fusarium oxysporum f. sp. ciceris races. PLoS One. 8:e61360.
- Kesten C, Menna A, Sánchez-Rodríguez C. 2017. Regulation of cellulose synthesis in response to stress. Curr Opin Plant Biol. 40:106–113.
- Köhle H, Jeblick W, Poten F, Blaschek W, Kauss H. 1985. Chitosan-elicited callose synthesis in soybean cells as a Ca2+-dependent process. Plant Physiol. 77:544–551.
- Kumar S, Abedin MM, Singh A, Das S. 2020. Role of Phenolic Compounds in Plant-Defensive Mechanisms. p. 517-532.
- Larkin RP, Fravel DR. 1998. Efficacy of various fungal and bacterial biocontrol organisms for control of Fusarium wilt of tomato. Plant Dis. 82:1022–1028.
- Lyon GD, Heilbronn J, Forrest RS, Johnston DJ. 1992. The biochemical basis of resistance of potato to soft rot bacteria. Neth J Plant Path. 98:127–133.
- Macheix JJ, Sapis JC, Fleuriet A, Lee CY. 1991. Phenolic compounds and polyphenoloxidase in relation to browning in grapes and wines. Critical Reviews in Food Science & Nutrition. 30:441–486.
- Malinovsky FG, Fangel JU, Willats WGT. 2014. The role of the cell wall in plant immunity. Front Plant Sci. 5:178.
- Mauch-Mani B, Slusarenko AJ. 1996. Production of salicylic acid precursors is a major function of phenylalanine ammonia-lyase in the resistance of Arabidopsis to peronospora parasitica. Plant Cell. 8:203–212.
- Mayer AM, Harel E, Ben-Shaul R. 1966. Assay of catechol oxidase—a critical comparison of methods. Phytochemistry. 5:783–789.
- Mushtaq T, Shah AA, Akram W, Yasin NA. 2020. Synergistic ameliorative effect of iron oxide nanoparticles and Bacillus subtilis S4 against arsenic toxicity in cucurbita moschata: polyamines, antioxidants, and physiochemical studies. Int J Phytoremediation. 22:1408–1419.
- Nawrocka J, Małolepsza U, Szymczak K, Szczech M. 2018. Involvement of metabolic components, volatile compounds, PR proteins, and mechanical strengthening in multilayer protection of cucumber plants against Rhizoctonia solani activated by Trichoderma atroviride TRS25. Protoplasma. 255:359–373.
- Niu X, Chen M, Huang X, Chen H, Tao A, Xu J, Qi J. 2017. Reference gene selection for qRT-PCR normalization analysis in kenaf (hibiscus cannabinus L.) under abiotic stress and hormonal stimuli. Front Plant Sci. 8:771. Epub 2017/05/30.
- Ongena M, Jourdan E, Adam A, Paquot M, Brans A, Joris B, Arpigny JL, Thonart P. 2007. Surfactin and fengycin lipopeptides of Bacillus subtilis as elicitors of induced systemic resistance in plants. Environ Microbiol. 9:1084–1090.
- Pantelides IS, Tjamos SE, Paplomatas EJ. 2010. Insights into the role of ethylene perception in tomato resistance to vascular infection by verticillium dahliae. Plant Pathol. 59:130–138.
- Pina A, Errea P. 2008. Differential induction of phenylalanine ammonia-lyase gene expression in response to in vitro callus unions of prunus spp. J Plant Physiol. 165:705–714.
- Pomar F, Merino F, Barceló AR. 2002. O-4-Linked coniferyl and sinapyl aldehydes in lignifying cell walls are the main targets of the Wiesner (phloroglucinol-HCl) reaction. Protoplasma. 220:0017–0028.
- Preisner M, Kulma A, Zebrowski J, Dymińska L, Hanuza J, Arendt M, Starzycki M, Szopa J. 2014. Manipulating cinnamyl alcohol dehydrogenase (CAD) expression in flax affects fibre composition and properties. BMC Plant Biol. 14:1–18.
- Proestos C, Komaitis M. 2013. Analysis of naturally occurring phenolic compounds in aromatic plants by RP-HPLC coupled to diode array detector (DAD) and GC-MS after silylation. Foods (Basel, Switzerland). 2:90-99. Epub 2013/03/13.
- Quiroga M, Guerrero C, Botella MA, Barceló A, Amaya I, Medina MI, Alonso FJ, de Forchetti SM, Tigier H, Valpuesta V. 2000. A tomato peroxidase involved in the synthesis of lignin and suberin. Plant Physiol. 122:1119–1128.
- Ruuhola T, Julkunen-Tiitto R, Vainiotalo P. 2003. In vitro degradation of willow salicylates. J Chem Ecol. 29:1083–1097.
- Sattler S, Funnell-Harris D. 2013. Modifying lignin to improve bioenergy feedstocks: strengthening the barrier against pathogens? Front Plant Sci. 4:70.
- Scott Jr TA, Melvin EH. 1953. Determination of dextran with anthrone. Anal Chem. 25:1656–1661.
- Segarra G, Jáuregui O, Casanova E, Trillas I. 2006. Simultaneous quantitative LC–ESI-MS/MS analyses of salicylic acid and jasmonic acid in crude extracts of cucumis sativus under biotic stress. Phytochemistry. 67:395–401.
- Sharifi R, Ryu C-M. 2016. Are bacterial volatile compounds poisonous odors to a fungal pathogen botrytis cinerea, alarm signals to Arabidopsis seedlings for eliciting induced resistance, or both? Front Microbiol. 7:196.
- Shi J, Mueller WC, Beckman CH. 1992. Vessel occlusion and secretory activities of vessel contact cells in resistant or susceptible cotton plants infected with Fusarium oxysporum f. sp. vasinfectum. Physiol Mol Plant Pathol. 40:133–147.
- Siddique Z, Akhtar KP, Hameed A, Sarwar N, Imran Ul H, Khan SA. 2014. Biochemical alterations in leaves of resistant and susceptible cotton genotypes infected systemically by cotton leaf curl burewala virus. Journal of Plant Interactions. 9:702–711.
- Taheri P, Tarighi S. 2012. The role of pathogenesis-related proteins in the tomato-Rhizoctonia solani interaction. Journal of Botany. 2012:1–6.
- Thakur M, Sohal BS. 2013. Role of elicitors in inducing resistance in plants against pathogen infection: a review. Int Sch Res Notices. 1–10.
- Weiergang I, Hipskind JD, Nicholson RL. 1996. Synthesis of 3-deoxyanthocyanidin phytoalexins in sorghum occurs independent of light. Physiol Mol Plant Pathol. 49:377–388.
- Yasin NA, Ahmed S. 2016. Induction of defence-related biochemicals by rhizosphere bacteria against black spot disease of rose. Biol Agric Hortic. 32:34–46.
- Yuan M, Huang Y, Ge W, Jia Z, Song S, Zhang L, Huang Y. 2019. Involvement of jasmonic acid, ethylene and salicylic acid signaling pathways behind the systemic resistance induced by Trichoderma longibrachiatum H9 in cucumber. BMC Genomics. 20:1–13.
- Zhang L, Wang G, Chang J, Liu J, Cai J, Rao X, Zhang L, Zhong J, Xie J, Zhu S. 2010. Effects of 1-MCP and ethylene on expression of three CAD genes and lignification in stems of harvested tsai Tai (brassica chinensis). Food Chem. 123:32–40.
- Zhishen J, Mengcheng T, Jianming W. 1999. The determination of flavonoid contents in mulberry and their scavenging effects on superoxide radicals. Food Chem. 64:555–559.
- Zieslin N, Ben-Zaken R. 1993. Peroxidase activity and presence of phenolic substances in peduncles of rose flowers. Plant Physiology and Biochemistry (Paris). 31:333–339.